Chronobiology
Introduction
Some researchers see chronobiology as a discipline separate from sleep research and sleep medicine, and there are entire conferences devoted exclusively to comparative and human circadian rhythms research. According to this view, sleep and wake are merely sets of parameters that have circadian modulation. In contrast, others see all of chronobiology as a subset of sleep research. Fortunately, these distinctions are mostly artificial; and, in fact, there is growing integration of the findings of circadian rhythm basic science research with those of basic and applied sleep research, the benefit being an improved understanding of the pathophysiology of many of the disorders of sleep. This benefit is not limited merely to the so-called circadian rhythm sleep disorders (see Chapter 5), but also applies to all other categories of sleep disorders including insomnia. Thus, a comprehensive understanding of human chronobiology is essential to optimal evaluation and treatment of any patient with a sleep–wake complaint.
Circadian Regulatory System
A well-known finding in circadian physiology is that core body temperature typically rises across the circadian day and falls during the circadian night1 independent of perturbations such as slow wave sleep deprivation.2 Urine production falls during the circadian night.3 A large release of cortisol precedes the beginning of the circadian day.4 These are just a few examples of rhythmic changes in physiological processes that likely aid the consolidation of sleep at night and the level of alert functioning in the daytime. Furthermore, the circadian system also has a direct effect on sleep–wake regulation, actively driving wakefulness during the daytime and actively driving sleep at night.5,6
A number of different laboratory protocols have been utilized to study human circadian rhythms. Early research on the human circadian system utilized the 90-minute day.7,8 Here, young adults were allowed to remain awake for 60 minutes before being given a 30-minute opportunity to sleep; this 90-minute cycle was then repeated for 24 hours or more. This design allowed researchers to examine the distribution of sleep and wake episodes across the 24-hour day, learning how sleep propensity (the likelihood that sleep will occur) and sleep structure (e.g., the pattern of sleep stage cycling) varied across the day and night. A more recent modification of this protocol is the ultrashort sleep–wake schedule,9–12 which has wake episodes of only 13 minutes alternating with sleep opportunities of only 7 minutes. Although these approaches provide interesting data on circadian modulation of sleep and wakefulness, the ability to draw meaningful conclusions about circadian modulation under normal conditions is limited since participants in these protocols never accumulate the normal 14–18 hours of continuous wakefulness or the usual 7–9 hours of sleep.
The constant routine protocol, as reviewed by Duffy and Dijk,13 requires participants to remain awake while semi-recumbent on bed rest – under constant illumination and temperature, and with small frequent equally spaced meals – for longer than 24 hours; the goal here is to remove or evenly distribute confounding or masking effects such as sleep–wake state, level of physical activity, light, digestion, temperature, and posture. The constant routine is the gold standard for assessing the phase of the circadian system (i.e., its timing relative to the clock) and its amplitude, but because sleep deprivation occurs during this protocol, and because the durations of the wake episodes are long and typically confounded by circadian phase, the assessment of the effects of circadian phase alone on a given function is hampered.
Free-running protocols1,14,15 are long-duration studies (typically lasting several weeks) conducted in an environment isolated from obvious time cues; participants self-select their bed and wake-up times. The circadian system drifts at its intrinsic period, and the sleep–wake cycle may desynchronize from the circadian system and take on a period typically much longer than 24 hours. Unfortunately, this self-selection of light–dark cycles – in free-run studies – often allows exposure to levels of light sufficient to produce patterns of relative coordination, or systematic biasing of circadian phase based on light exposure, that corrupts attempts to measure variables such as intrinsic circadian period.16
Perhaps the most complex and labor-intensive way to investigate human circadian rhythms is with the forced desynchrony protocol. Pioneered by Kleitman,17 and refined in several laboratories,5,18,19 participants are scheduled in a multi-week protocol to a sleep–wake period (T-cycle) significantly different from the near-24-hour circadian period. Under this protocol, and in the absence of obvious time cues, the circadian system free runs and sleep and wake episodes appear across a wide range of circadian phases. Thus, each data point can be assigned one coordinate for circadian phase and another for, perhaps, duration of the elapsed wakefulness or duration into the scheduled sleep episode, and the data measured might be reaction time or a 30-second epoch of sleep. As reviewed later in this chapter, results from the forced desynchrony protocol have allowed a rich understanding of circadian modulation of sleep and wakefulness.
Circadian research has also demonstrated many fundamental properties of this system. The ability of the circadian system to facilitate the proper timing of physiological processes is dependent on periodic input of timing signals. The environmental light–dark cycle is the primary periodic input or zeitgeber (time giver) in human circadian rhythms. Without access to robust cues of bright light during daytime and darkness at night, the circadian system relies entirely on its near-24-hour intrinsic period of oscillation. As this oscillation is not exactly 24 hours in duration but is consistent in period length, absence of daily resetting from zeitgebers leads to phase drifting, typically in a later direction (though occasionally in an earlier one), relative to the 24-hour day. This type of free-running pattern is what occurs in many blind individuals; their timing system develops a progressive drift, and they complain of intermittent nighttime insomnia or daytime sleepiness. In contrast, with daily exposure to the external 24-hour light–dark cycle (with light either from the sun or from artificial light of sufficient intensity), the circadian system maintains stable alignment to the 24-hour day and produces the desired, non-drifting, sleep–wake cycle. This is known as entrainment. In environments free of strong zeitgebers, the period of the intrinsic circadian rhythm has been shown to be approximately 24.2 hours in healthy young and older adults.6,20 Intrinsic periods have also been measured in adolescent children aged 10–15 and found to be approximately 24.3 hours.18 Given that the circadian system has a non-24-hour free-running intrinsic period, it must be reset daily to maintain entrainment to the 24-hour day. Given that the average period length is (usually) slightly longer than 24 hours, the circadian phase (usually) must adjust earlier each day to remain aligned with the light–dark cycle. Larger phase shifts are required to adapt after rapid crossing of time zones (as occurs with jet travel). Hence, there is a critical reliance of the circadian system on the timing and intensity of zeitgebers (e.g., the light–dark cycle) to adjust and maintain phase.
The timing of a light stimulus affects the magnitude and direction of circadian phase shifting. An early demonstration of the relationship between the circadian phase of light exposure and the response of circadian phase shifting in a mammal was made in flying squirrels.21 A graphical depiction of this type of response curve was developed and is known as the phase response curve (PRC).21 Light exposure at certain circadian phases has minimal phase shifting effects, while light exposure at other circadian phases results in phase delays (shifting later) or phase advances (shifting earlier) of circadian rhythms with the amount and direction of shift dependent upon the timing, intensity, and duration of exposure. In humans on a normal sleep pattern (with relatively stable bedtimes and wake times, sleeping at night, and being awake during the daytime), light exposure at clock times ranging from late in the biological day to early in the biological night will produce a phase delay, whereas light exposure at times from late in the biological night to the early hours of the biological day will produce a phase advance.22–24 At a critical circadian phase, typically several hours prior to the morning wake time and near the trough of core body temperature, there is a crossover zone. Light exposure will lead to the largest phase delays when delivered just prior to the crossover zone, and the largest phase advances when delivered just after the crossover zone. Figure 4-1 illustrates the phase shifting effects of bright light.
Figure 4-1 Type 1 Phase Response Curve for Light.
The horizontal axis represents circadian phase position with hour intervals, where 0 corresponds to the trough of core body temperature, which normally occurs 1–3 hours prior to average wake time. The vertical axis represents the amount and direction of phase shifting, with the convention of phase advances being plotted as positive values and phase delays being plotted as negative values. Figure reproduced with permission from Khalsa SB, Jewett ME, Cajochen C, Czeisler CA. A phase response curve to single bright light pulses in human subjects. J. Physiol. 6/15/2003 2003;549(Pt 3):945–952.
PRCs have also been constructed to demonstrate the circadian phase shifting effects of exogenous melatonin administration. The PRCs for melatonin and light exposure are nearly opposite in their timing. Thus, melatonin administration during the period from afternoon through the early evening produces a circadian phase advance, while melatonin administration during the period from late nighttime through early morning hours produces a circadian phase delay.25–27 Figure 4-2 illustrates the phase shifting effects of melatonin administration.
Figure 4-2 Phase Response Curve for Melatonin.
The upper horizontal axis represents circadian phase position with hour intervals, where 0 corresponds to the dim light melatonin onset (DLMO), which normally occurs 1–3 hours prior to average bedtime. Corresponding clock time is shown on the lower axis for clarity. The vertical axis represents the amount and direction of phase shifting, with the convention of phase advances being plotted as positive values and phase delays being plotted as negative values. Figure reproduced with permission from Burgess HJ, Revell VL, Eastman CI. A three pulse phase response curve to three milligrams of melatonin in humans. J. Physiol. 1/15/2008 2008;586(2):639–647.
Zeitgeber intensity also affects the amount of resultant circadian phase shifting. The original PRCs22–24 were constructed with very bright artificial indoor light (i.e., approximately 5000–10 000 lux, which is an intensity of light exposure similar to that observed outdoors at sunrise or sunset). However, the relationship between light intensity and the amount of phase shifting is nonlinear; thus, even average indoor room light intensity can produce phase shifting.28,29
The duration of light exposure also determines the amount of phase shifting. The original PRCs to light were constructed with multiple-hour light exposure (e.g., six consecutive hours). A 2012 study has demonstrated that even an hour of light exposure can result in significant phase shifting.30 Similar results have been reported with intermittent light exposure31,32 (alternating periods of bright and dim light exposure). These observations support the conclusion that a nonlinear relationship also exists between duration of light exposure and the amount of phase shift.33
Early research on photic circadian shifting relied upon full-spectrum artificial lighting, approximating natural sunlight. Subsequent research has found that the circadian system is maximally sensitive to visible blue light.34 Current investigations are measuring the amount of circadian phase shifting caused by exposure to commonly encountered light-emitting devices with significant blue light spectrum, such as computer monitors, laptop monitors, and handheld computer devices. The results of these studies should improve our understanding of how some of the circadian rhythm sleep disorders develop and are maintained, explain the existence of barriers to treatment, and help us design optimal treatment protocols.
The central element of the circadian system is the suprachiasmatic nucleus (SCN), a bilaterally represented structure in the hypothalamus.35,36 The SCN is sometimes referred to as the circadian clock. As noted above, the circadian system requires periodic input from the light–dark cycle to maintain optimal phase alignment with a nighttime sleep episode and a daytime wake episode. Environmental light is transduced to neuronal signals at the level of specialized retinal ganglion cells.37 This circadian vision system connects to the SCN via the retinohypothalamic tract.38 The SCN has many output pathways to influence other brain structures. One that has been well studied is a multi-synaptic pathway connecting the SCN to the pineal gland, the most important source of melatonin in the CNS.39
Sleep Homeostatic System
Although this is a chapter on chronobiology and specifically on human circadian rhythms, it would be incomplete to discuss the circadian system without discussing its equally important counterpart. The second major modulatory process affecting sleep and wakefulness is the sleep homeostatic process. Put simply, it is as if the CNS keeps track of each hour of continuous wakefulness, with ever-building sleepiness and sleep propensity. Though not directly measureable in humans, a proxy for the accumulation of sleep homeostatic drive can be measured via spectral composition of the waking EEG, such as spectral power in the delta band in frontal leads.40 Similarly, the dissipation of sleep homeostatic drive during sleep episodes can be estimated from measurement of spectral power of slow-wave activity (SWA).41–44 Following partial sleep restriction, there is an enhancement in SWA in recovery sleep.42,45
For many decades there have been hypnotoxin theories suggesting that across the duration of an episode of wakefulness, a substance or substances build up in the brain and/or cerebrospinal fluid (CSF) that have a negative impact on alert brain functioning, thereby increasing the likelihood of sleep. Several candidate substances have been identified to support this process, including early findings for delta sleep-inducing peptide and sleep factor S. More recent investigation has pointed to the importance of adenosine as a sleep-promoting substance that may be a key element of the sleep homeostatic process.46,47
The Two-Process Model
The two-process model of sleep–wake regulation is a useful way of considering the simultaneous impact of the sleep homeostatic process and the circadian regulatory system on sleep and wakefulness.48 Use of the forced desynchrony protocol over the past few decades has allowed for estimation of the independent influence of each of the two processes and, perhaps more importantly, their nonlinear interaction.5,6 An example of this nonlinear interaction is that the amplitude of circadian modulation of alertness is quite small when measured with a concurrent low level of sleep homeostatic drive, and systematically larger with increasing extent of sleep homeostatic drive.6,49 One of the important findings of these forced desynchrony studies has been demonstration of the difficulty knowing the extent to which certain phenomena – when they occur at a certain time of day – are caused by the homeostatic system, the circadian system, or a combination of both. For example, the depth and duration of the midafternoon trough of alertness is related both to the circadian and to the homeostatic systems. It is unfortunate that many published reports describing circadian rhythms of certain events or biological processes fail to measure or even estimate or consider the effects of circadian phase and sleep homeostatic pressure on the rhythms studied.
Another important outcome of forced desynchrony (and some earlier free-run) studies is the finding that circadian systems are crucial to the maintenance of sustained bouts of wakefulness during the day and sustained periods of sleep at night.5 If the homeostatic system were the only one present, alertness would decline across the waking day, reaching critical levels of impairment in the second half of the daytime; sustained wakefulness across the daytime would be extremely difficult if not impossible. However, the circadian system actively promotes alertness across the waking day, opposing the build-up of sleep homeostatic drive. The circadian system reaches its maximal drive for alertness a few hours prior to habitual bedtime (e.g., approximately 7 p.m. for a child routinely sleeping from 9 p.m. to 6 a.m.), a period known as the forbidden zone for sleep12 or as the wake maintenance zone.50 Similarly, sleep homeostatic drive decreases across the nighttime sleep episode. If only a sleep homeostatic system were present, sleep would become progressively fragmented with intrusions of wakefulness in the second half of the night. Fortunately, the circadian system has a sleep-promoting function during the night, with increasingly greater sleep drive across the nocturnal sleep episode. Curiously, the circadian drive for sleep reaches its maximal level at normal wake time and for an additional 2–3 hours (e.g., approximately 6 a.m. to 9 a.m. for a child routinely sleeping from 9 p.m. to 6 a.m.). The time window of maximal sleep promotion has been called the circadian sleep maintenance zone.49,51
Development of Circadian Rhythms – Infancy Through Adulthood
It has been demonstrated that late in fetal development there is the differentiation between active and quiet sleep.52 Confirmatory evidence comes from observations of infants born prematurely.53 This is only to say that there is early development of sleep state regulation occurring prior to birth. Similarly, observations from nonhuman primates suggest the consolidation of circadian rhythms starts during fetal development.54 In humans, there have been reports of variations in fetal heart rate synchronized to the mother’s circadian rhythms,55 as well as an observed 24-hour periodicity in cortisol secretion in the full-term fetus.56 Observations such as these suggest the functioning of at least a rudimentary circadian system occurs prior to full-term birth. However, much of the animal literature on entrainment or phase shifting of fetal and/or neonatal circadian rhythms may not translate to humans and hence will not be reviewed here, given that many of the non-photic zeitgebers that can shift rhythms in animals (e.g., timing of feeding or activity) are weak or inactive as zeitgebers in humans. For a comprehensive review of the animal research findings in fetal and early-life development of the circadian system, see Sumova et al.57
In the human newborn, a circadian pattern of core body temperature oscillation develops by approximately 2 to 3 months of age,58 or perhaps even earlier as suggested in a case report.59 A circadian rhythm of salivary cortisol is established as early as the first 2 months of infancy, but different methods of data analysis produced results suggesting that this rhythm is not established until months later, and high individual differences were noted.60 An early report described a melatonin rhythm in infants aged 6–9 months; in this study estimations were made from measurements of a urinary metabolite of melatonin collected from the diaper across a 60-hour interval.61 Individual differences were also emphasized in this report, but there were intriguing findings, such as of a correlation between higher levels of a melatonin metabolite in the evening hours, and earlier hours of sleep onset at night. There has also been a partial validation of a salivary melatonin collection protocol (commonly used in children and adults) for newborns, showing a reasonable correlation with blood sample melatonin levels.62 This procedure could be utilized to sample melatonin more frequently, such as is done during the dim light melatonin onset (DLMO) protocol63 in children and adults (as described below in the circadian modulation section).
Much of the literature on circadian rhythms in young children is focused on those with neurodevelopmental disorders, and hence there is a gap in knowledge for typical early childhood development of the circadian system. For example, it has been reported that 2- to 10-year-old children with autism may exhibit fragmented sleep with delayed timing relative to the sleep–wake schedule desired by the parents, and these patterns are responsive to exogenous melatonin administration.64 A blunted or absent circadian rhythm of melatonin has also been reported in autism,65,66 but it is unclear if this is evidence of a primary problem with the circadian pacemaker itself, with the pineal gland itself, or with the circadian regulation of pineal synthesis of melatonin. Similar sleep delay and disruption, and melatonin treatment responsiveness, has been reported for children with Angelman syndrome.67 Other circadian research in pre-teenage children has focused on chronotype (see next section).
The most comprehensive study of circadian rhythms in children has been on youngsters in the years of pubertal development. Carskadon’s research laboratories at Stanford University and Brown University have been the sites of important studies on adolescents in this age range. Much of the early studies focused on sleep and sleep deprivation, but more recent protocols have included detailed circadian assessments. A fundamental finding has been the measurement of circadian period, which is slightly longer in adolescents than in adults (approximately 24.3 hours as noted above).18 Another well-documented finding is that of a delay of the timing of the nocturnal sleep episode during the teenage years68 thought to result from a combination of social, behavioral, and biological factors (see Chapter 6). Implications are clear for the development of delayed sleep phase disorder (see Chapter 5), which often begins or worsens in the teenage years.
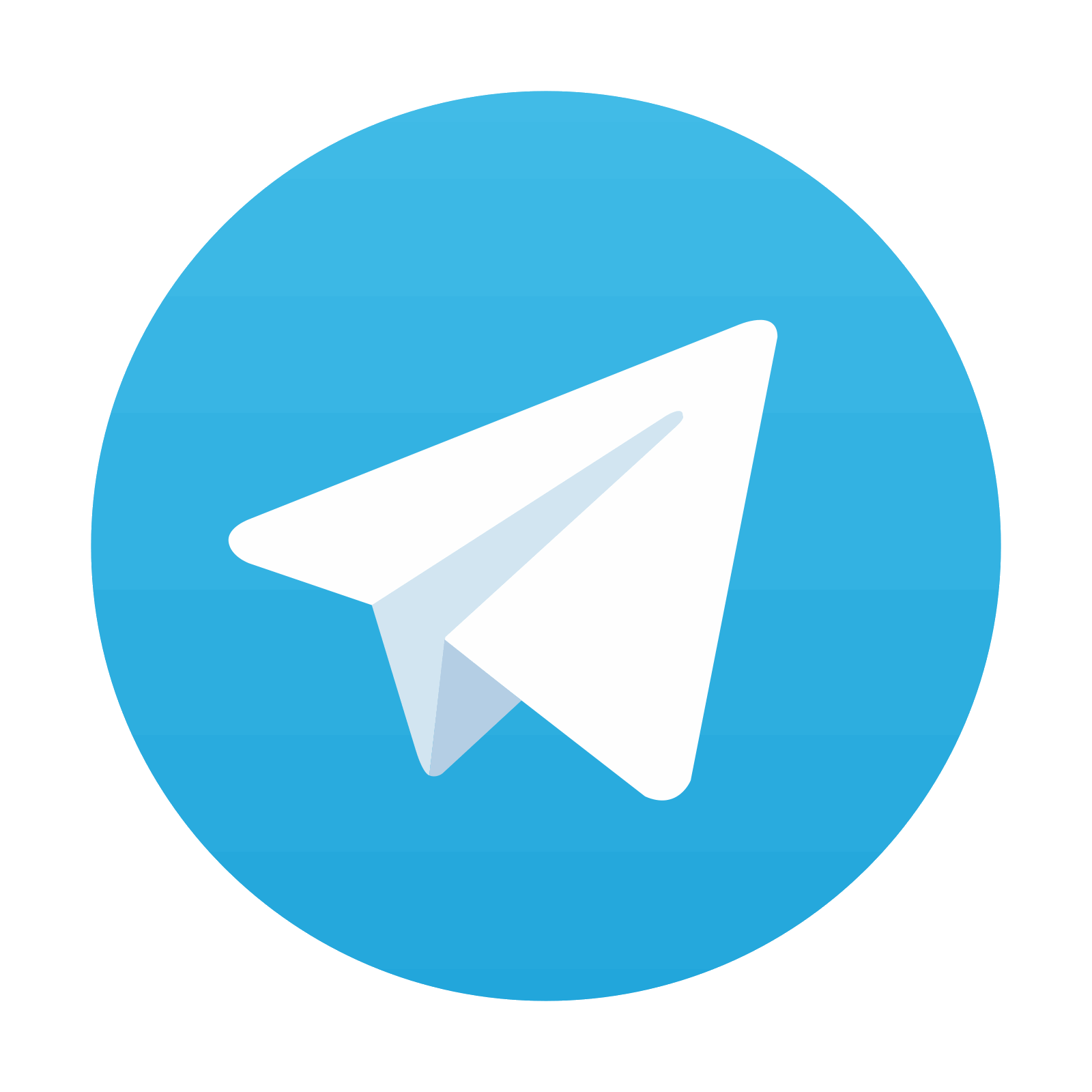