Abstract
Fertility, pregnancy and childbirth are connected and inseparable realities that ultimately need to combine to result in a “baby at home”; each one of these phenomena possesses their own suite of challenges and limitations. From a biological viewpoint, the factors contributing to a successful “baby at home” are male factors (mainly sperm quality) and female factors (mainly oocyte quality and endometrial receptivity). Any failure or disability in one of these competences shall likely contribute to the reduced probability of having a “baby at home”. However, in modern times a new factor is playing a crucial role in this scenario, the so called “Artificial Reproduction Techniques” (ART). Thus, it is becoming increasingly possible for reproductive biologists and clinicians to apply a range of technologies to transform a biologically infertile couple to an assisted fertile one. To this purpose, a series of novel technologies, some of them with ethical implications at the time of their use on a routine basis, have and will continue to have a remarkable potential impact on fertility treatments [1].
22.1 Introduction
Fertility, pregnancy and childbirth are connected and inseparable realities that ultimately need to combine to result in a “baby at home”; each one of these phenomena possesses their own suite of challenges and limitations. From a biological viewpoint, the factors contributing to a successful “baby at home” are male factors (mainly sperm quality) and female factors (mainly oocyte quality and endometrial receptivity). Any failure or disability in one of these competences shall likely contribute to the reduced probability of having a “baby at home”. However, in modern times a new factor is playing a crucial role in this scenario, the so called “Artificial Reproduction Techniques” (ART). Thus, it is becoming increasingly possible for reproductive biologists and clinicians to apply a range of technologies to transform a biologically infertile couple to an assisted fertile one. To this purpose, a series of novel technologies, some of them with ethical implications at the time of their use on a routine basis, have and will continue to have a remarkable potential impact on fertility treatments [1].
Infertility associated with a male factor has been estimated to affect approximately 50 percent of infertile couples [2, 3], but when semen parameters are in the normal range, as defined by the World Health Organization (WHO), a reliable diagnosis of infertility associated to male factor remains elusive. Given this issue, any new technique that is able to shed some light to elucidate what might be described as a “third factor of infertility”, would be of substantive value to helping couples achieve a successful pregnancy. This is one of the reasons why Sperm DNA Fragmentation (SDF) is considered a promising diagnostic tool for the management and diagnosis of male infertility with both research and clinical applications [4].
High levels of SDF in the ejaculate are associated with an increased time to conception, impaired fertilization, decreased early embryo development, higher miscarriage rates and recurrent pregnancy loss after ART [4, 5, 6, 7, 8]. Thus, the detection of anomalous levels of SDF can serve as an additional marker of fertility potential and a predictor of the success of pregnancy. A significant proportion of men with normal semen profiles are still considered infertile [3], suggesting that other semen parameters also contribute to understanding unexplained infertility; clearly sperm DNA damage and associated DNA modifications are important potential sperm parameters that have a role here [9].
Since the proposal by Evenson that flow cytometry be used as a valuable methodology to assess SDF [5], a series of alternative techniques have subsequently emerged. These include the TUNEL assay, the Comet assay (with different variants) and the Sperm Chromatin Dispersion (SCD) test. All of these techniques are considered reliable methodologies providing consistent results on the sperm DNA quality of donors and patients. All of them offer the possibility to assess DNA quality in fresh, processed or cryopreserved samples. In this paper, we review some aspects of the SCD test and derived results of its application in clinical practice, including the rationale of the technique and implications in different fields of ART.
22.2 Methodological Basis of the Sperm Chromatin Dispersion Test
The SCD test was serendipitously discovered when using the DNA Breakage Detection-Fluorescence In Situ Hybridization (DBD-FISH), a technique that is a very sensitive assay in determining DNA breaks. From a conceptual viewpoint, DBD-FISH is a straightforward methodology. Unfixed cells, which are enclosed in an inert thin microgel on a slide, are incubated with an alkaline DNA denaturing solution and with lysing solutions to remove proteins. The alkaline solution is a main step in the DNA unwinding assays and classically employed to evaluate ionizing radiation induced DNA breaks. High pH conditions denatures the DNA starting from the end of the DNA breaks (5´–3´ free ends), producing stretches of single-stranded DNA that may be quantified by different approaches.
Nuclear proteins pack the DNA molecules in the nuclear volume, while the lysing solutions remove proteins and membranes from the cell and the nucleus. Subsequently, after extensive nuclear protein removal, DNA is unpackaged producing a peripheral halo of chromatin loops that spread from the central remnant core. These are remnant cores known as nucleoids and they remain retained within the microgel after the whole process. Single-stranded DNA motifs produced by the alkaline unwinding treatment from the DNA breaks may then be detected under fluorescence microscopy by hybridization of fluorescent labeled DNA probes. The higher the number of DNA breaks, the higher the amount of single-stranded DNA motifs produced by the denaturing solution, the higher the amount of hybridized probe and the visualized fluorescence (Figure 22.1A,B). The hybridized DNA probe can be quantified by digital image analysis software (Figure 22.1C–F). The system is sensitive enough to assess DNA breaks in the whole genome or within specific DNA sequence domains such as repetitive DNA sequences or single gene copies [10]; (Figure 22.1G,H). DBD-FISH evaluates possible differences in sensitivity to DNA damaging agents in different regions of the genome.
Figure 22.1 DNA Breakage Detection-Fluorescence In Situ Hybridization (DBD-FISH) in somatic cells. A) DBD-FISH using a whole DNA genomic probe on a somatic cell in absence of DNA damage. B,C) Flat (B) and 3D planimetry (C) of Figure 21.1A. D) DBD-FISH using a whole DNA genomic probe on a somatic cell in absence of DNA. E,F) Flat (E) and 3D planimetry (F) of Figure 22.1D. DBD-FISH using restrictive human centromeric DNA probes satellite 1 (red) and satellite 2 (green) of chromosome 1 in untreated (G) and irradiated (H) cells. Note the difference in amount of hybridized DNA probe according with the expected level of DNA damage.
When the DBD-FISH protocol was applied to native human sperm cells, without exposure to any damaging agent, it was observed that a certain and variable proportion of spermatozoa, depending on the ejaculate used, appeared strongly labeled when using a whole genome DNA probe, showing an intense fluorescence. These sperm were interpreted and presenting with a very high amount of DNA breakage, i.e. DNA fragmentation; the rest of the sperm showed a slight labeling, mainly within specific regions of the nucleoid. Counterstaining of the DNA revealed that the nucleoids from sperm with non-fragmented DNA presented the typical central core and peripheral halo of spread DNA loops (Figure 22.2A). Surprisingly, those sperm strongly labeled after the DBD-FISH protocol, i.e. containing fragmented DNA, did not show peripheral halos or these were small by comparison but they presented a high level of hybridized DNA probe (Figure 22.2B).
Figure 22.2 Visualization of different sized haloes depending on the level of DNA damage observed after DNA denaturation and protein depletion. A) Spermatozoa stained for fluorescence microscopy. Red arrows indicate those spermatozoa without DNA damage. B) Same image as Figure 22.2A but after DBD-FISH. Note that spermatozoa with large haloes are free of hybridization (white arrows) while those with small haloes in Figure 22.2A show large signals of hybridization. C) SCD test but visualized under bright filed microscopy.
As a direct correlation between the presence of halo and DNA-labeling intensity can be established, the long hybridization step could then be omitted resulting in a more efficient procedure. The rationale of the DBD-FISH was simplified and sperm enclosed in the microgel are then simply incubated with an unwinding solution to denature DNA presenting free ends and later with lysing solutions to remove proteins. Finally, the resulting nucleoids can be stained and visualized even using bright field microscopy (Figure 22.2C). Spermatozoa exhibiting small halo or no halo correspond to sperm with fragmented DNA while those presenting a large halo of dispersed chromatin were considered as those presenting non-fragmented DNA molecules. This simplified assay was coined the “Sperm Chromatin Dispersion test” [11].
The reality is that sperm DNA damage affects every cell continuously and it is expected that different levels of damage are therefore likely to present differentially. Within the context of interpreting results of the SCD test, these differences are characterized with the visualization of a series of different sized haloes after staining (Figure 22.3A,B) and with the help of digital image analysis, different cell subpopulations can be discriminated [12]; (Figure 22.3C).
Figure 22.3 Different sized haloes in spermatozoa processed with the SCD test. A) 1–2: large haloes = no fragmented sperm; 3: medium-sized halo = no fragmented sperm; 4: small halo = fragmented sperm; 5 and 6: no halo and degraded sperm respectively = fragmented sperm. B) Color planimetry to show density differences in the different nucleoids. C) Multidimensional plot obtained using morphometric variables (area, perimeter and associated variances) to show the differential clustering obtained. Color code in Figure 22.3C corresponds to color number in Figure 22.3B.
Following this initial development, the procedure was refined, designing an exclusive lysing solution, specific to human sperm, that removes protamines while keeping the sperm tails visible. This was possible by exchanging the alkaline solution with an acidic solution. The main advantage of using acid denaturation is that it is less strong than the alkaline one to denature from DNA ends on the DNA damaged molecule. Spermatozoa with fragmented DNA produce single-stranded DNA stretches that after protein removal give rise to small or no haloes. Spermatozoa without DNA fragmentation practically do not denature the DNA and after protein depletion, the peripheral haloes are larger than those observed after using the stronger alkaline solution. The use of a controlled acid denaturation improves the contrast between sperm with and without fragmented DNA; furthermore, the technique allows for better nucleoid staining not only by fluorochromes but also by standard dyes like Wright or Diff-Quik staining procedures, allowing the use of the basic bright-field microscope for visualization of the haloes [13]. The actual SCD protocol, including the scoring of 300–500 sperm, may be accomplished in less than an hour. The assay has now been standardized as a commercialized kit (Halosperm®; Halotech DNA SL, Madrid, Spain), being user-friendly, not technically demanding and easy to be implemented at any laboratory to obtain reliable and consistent results.
Different approaches validate the ability of the SCD test to determine SDF. First at all, the sequential DBD-FISH technique performed in each specific sperm (Figure 22.2), confirms that those spermatozoa without haloes or with small haloes contain massive DNA breakage. Moreover, the SCD-processed sperm may also be sequentially incubated for enzymatic labeling of DNA breaks, either with the terminal deoxynucleotidyl transferase (TdT; TUNEL assay) or the Klenow fragment of the DNA polymerase (Klenow-end labeling) or just using the DNA polymerase (ISNT: In Situ Nick Translation). As is the case with the DBD-FISH procedure, DNA breakage labeling by the different DNA polymerases is only observed in the sperm nucleoids without haloes or with a small halo. Other important proof of evidence is the incubation of sperm with DNA damaging agents. Treatment with DNase I or with free radicals such as hydrogen peroxide or sodium nitroprusside (SNP), results in the sperm displaying small or no haloes [14]. Finally, the SCD test strongly correlates with the results of other procedures to determine SDF, like the Sperm Chromatin Structure Assay (SCSA), TUNEL assay and the single-cell gel electrophoresis (SCGE)/Comet assay [15, 16, 17].
22.3 Advanced Applications of the Sperm Chromatin Dispersion Test
From a technical viewpoint, the SCD test may also be coupled with other techniques of interest for basic and clinical research. Staining with a mix of fluorochromes with different affinities and emission wavelengths may reveal different DNA characteristics of SCD-processed sperm. For example, DNA may be stained with a DNA interacting fluorochrome such as propidium iodide or Gel-Red, whereas proteins/protein remnants may be stained by 2,7-dibrom-4-hydroxy-mercury-fluorescein. Using this fluorochrome combination and a dual-band excitation-emission block filter, the nucleoids (mainly DNA) fluoresce red, while sperm tails (mainly proteins) fluoresce green (Figure 22.4A). This staining facilitates that somatic cells, like leukocytes, which may be present in the semen sample, can be easily discriminated from surrounding spermatozoa. Somatic cells show large round nucleoids without a prominent halo of dispersed chromatin. These cells exhibit a mixture of red and green fluorescence emissions due to the presence of DNA and histones retained in the nucleus. Under these staining conditions, somatic cells can be easily differentiated from the sperm heads (Figure 22.4B).
Figure 22.4 The SCD test used for different purposes. A) Simultaneous visualization of the sperm head and flagellum in a normal ejaculate. B) Testicular sperm. Discrimination between somatic (yellow cells without flagellum) and spermatozoa (red cells with flagellum). C–F) Use of SCD to assess sperm DNA damage in individualized spermatozoa. C) Intra-cytoplasmic morphologically selected sperm injection (IMSI) selected sperm showing normal head morphology and absence of DNA damage (D). E) Two selected spermatozoa showing head abnormalities. F) Same spermatozoa as in 22.4B but showing DNA damage. G) SCD visualizing different spermatozoa and presence of bacteria associated to a leukocyte. H) Detailed enlargement of Figure 22.4G.
This approach provides some advantages in certain clinical situations such as patients with leukocytospermia [18] or identification of testicular sperm where the number of somatic cells is high because of the invasive characteristics of sperm retrieval. Testicular samples often contain debris and other cell types like spermatogonia, spermatids or Sertoli cells making the discrimination from spermatozoa problematic. This situation can be extreme in some patients such as those presenting with Kartagener’s syndrome or varicocele [19, 20], but even in these patients, somatic cells can be easily distinguished using a combination of fluorochromes targeting DNA and proteins with different emission spectra.
Sperm DNA Fragmentation assessment in severe oligozoospermic samples is not possible with procedures based on flow cytometry, which require a relatively high amount of cells to be processed. The SCD procedure is also very effective when studying testicular sperm from biopsies or puncture-aspiration, spermatozoa isolated with restrictive selection systems such as MACS, PICSI or microfluidic based devices. The SCD can also be used to assess SDF in just one selected sperm, as in the case of IMSI [12]; (Figure 22.4C–F) or very few selected spermatozoa such us those captured after PICSI or MACS [21, 22].
Evaluation of modified DNA bases on SCD processed slides may be accomplished through incubation with specific antibodies. When SCD-processed sperm are incubated with antibodies binding to 8-oxoguanosine (8-oxoG), the presence of DNA fragmentation and the presence of oxidative damage can be simultaneously determined [23]. Additionally, the DNA methylation level, using an antibody that binds to 5-methylcytosine, can also be assessed in different spermatozoa after being processed with the SCD test (figure 10 in [24]).
Specific DNA sequences may be identified in SCD-processed nucleoids by standard FISH [25, 26]. To this purpose, dehydrated nucleoids are carefully denatured, trying to avoid loss of DNA from the haloes, and hybridized with specific DNA probes. Counterstained nucleoids reveal presence or absence of DNA fragmentation through evaluation of halo size, whereas the enumeration of signals from chromosome specific hybridization of DNA sequences indicates the presence or absence of aneuploidies. This strategy allows identification of DNA damage and presence of aneuploidies in the same sperm.
It is interesting to highlight that bacteria, when they are present at the ejaculate, are only partially affected by the lysing solutions used in the SCD protocol. Because of this chromatin relaxation, they remain more perceptible and produce a much more efficient fluorochrome-DNA interaction. The final result is that bacteria can be identified when they are present at the ejaculate whether they are free or endosymbiotic (Figure 22.4G,H); [27].
One peculiarity of the SCD test is the ability to distinguish a special kind of sperm with fragmented DNA, the “degraded” subtype. This is a sperm without halo but presenting an irregular or faint stained nucleoid and corresponds to a strongly damaged sperm containing both massive single- and double-strand DNA breaks [28]. In semen samples from men with varicocele, this “degraded” type has been found to be increased in proportion with respect to the whole population of sperm with fragmented DNA (sperm degradation index: SDI; [20]). A degradation index (considering this as the proportion of degraded sperm within the fragmented population) of >0.33 may predict the presence of varicocele, even when these are subclinical, with an accuracy of 94 percent [20].
22.4 Sperm DNA Fragmentation and Reproductive Outcome
A value of 30 percent SDF is regarded as one of the most accepted cut-off values of SDF that discriminates low from high levels of damaged DNA. Evenson and colleagues have suggested this value in different papers using the SCSA technique [6, 29]. The SCD technique was adapted to produce near equivalent results to those obtained with the SCSA technique [13]. However, discrepancies exist among the cut-off values when compared with other techniques such as TUNEL (lower values than 30 percent) or the alkaline comet assay (higher values than 30 percent). Probably, this is mostly related to the resolution capacity of each technique to capture the entire DNA damage present in the sperm, rather than with the results of reproductive outcome obtained. What is important is that all the techniques show good correlation at the time of determining variable levels of SDF existing among different patients [16, 30].
Numerous studies have reported a close correlation between SDF and clinical outcomes using different strategies for fertilization such as intrauterine insemination, in vitro fertilization and intracytoplasmic sperm injection [7, 31, 32, 33, 34, 35, 36]. While in general, a negative correlation has been found when SDF values are plotted against rates of fertilization, embryo quality and development, implantation rates, and pregnancy, this association is not perfect and some investigations point out to a quasi-neutral role of SDF in reproductive outcome [37, 38, 39]. One of the most inclusive studies to correlate SDF and pregnancy outcome was a multicenter survey including 622 couples [40]; these type of studies, at least, eliminate confounding factors such as variability of the technique to assess SDF, or inclusion criteria. Vélez de la Calle et al. [40] reported that the correlation between pregnancy and SDF was not as high as that reported in other studies, although they found a significant positive correlation for SDF and reduced rate of oocyte fertilization, embryo quality, and rate of blastocyst implantation.
One of the possible explanations for these discrepancies is that the assessment of SDF is usually performed in neat sperm and this is not the actual sample that will be used for fertilization purposes. In fact, the samples that will be used for fertilization are those obtained after performing different sperm selection techniques on the ejaculated sample. Each technique to select sperm presents different levels of skill and efficiency to remove damaged sperm [41]. Additionally, the ineludible negative impact of iatrogenic damage is rarely considered as an important modulator of SDF with respect to time. Another confounding factor is the quality of the embryo selected to be transferred. When different oocytes are fertilized but only one is transferred, this is a form of selection and this can mask the net effect of SDF on reproductive outcome. Then, the question would be: if a selected embryo fails to implant or develop, is this reflective of the other fertilized but not-transferred oocytes?
Understanding the actual role of SDF on reproductive outcome requires a consistent experimental model that is able to minimize confounding factors. The use of donated oocytes to be fertilized with the semen sample of the partner minimizes the role of female factor. This can be a good experimental strategy to understand the true role of male factor in reproductive outcome; nevertheless, even under these conditions, confounding factors cannot be discarded. For example, the receptive female is contributing to these factors with respect to her endometrial receptivity or other physiological characteristics that may be compromised, especially taking into consideration that the majority of these females are attending an ovodonation program for different abnormal physiological reasons. Using this experimental model, we performed a prospective study where each male partner was controled at different levels; SDF was assessed both at 30 minutes after ejaculation and at the time of fertilization. All the samples were processed with swim-up for sperm selection and used for ICSI. Fixing these conditions, receiver operator characteristics (ROC) showed that SDF cut-off values for pregnancy were 24.8 percent for neat sperm, while for swim-up processed samples these values decrease to 17.5 percent. Interestingly, prediction of pregnancy from ejaculated samples or swim-up processed samples were quite similar showing a sensitivity and specificity of 77 percent and 73 percent, respectively. These results show that while increased levels of SDF have a negative impact in reproductive outcome, the predictive values of SDF can be different if we only use as reference fresh ejaculated samples or samples that have been processed [12].
22.5 Assessing Sperm DNA Longevity with the Sperm Chromatin Dispersion Test
In those cases where ICSI, IVF or IUI are used for the purpose of fertilization, the sperm must be kept alive in in vitro conditions until the oocyte is reached. This unpretentious statement has crucial implications if we want to achieve a successful fertilization. The ejaculate is maintained in vitro for liquefaction, sperm capacitation, sperm selection and sperm-oocyte interaction. Altogether, these steps may impair the environment where the sperm is maintained free of non-desirable modifications at different levels. For many years now, it has been well known that sperm quality declines over time following ejaculation and semen characteristic such as membrane quality and sperm motility decrease after ejaculation, even when using the best sperm preservation environments; this produces a serious decline in the abilities of sperm to achieve reproductive outcome. We know now that DNA quality does not escape this general rule and DNA quality declines after ejaculation. This decline is different when comparing the sperm of individuals within one species or when different species are compared [42, 43, 44], or when cryopreserved samples are compared with fresh ones [45].
One important aspect of the dynamic assessment of DNA fragmentation for ART is taking into account the negative impact of iatrogenic damage caused by exposure of sperm to a panoply of changing ex vivo conditions. If the time elapsed between SDF assessment and fertilization is not considered, then it is not surprising that there is often not a strong correlation of these variables and we see mixed conclusions about the predictability of SDF and infertility. In a recent paper, and using a highly sensitivity technique to assess DNA damage as the two-tail Comet assay, our group has called attention to this particular issue. Cryopreserved human samples increase in single-strand DNA breaks after only 30 minutes post-thawing [47], and in most cases, we would generally not consider this potential DNA damage as a possible effector of pregnancy loss; perhaps we have underestimated this problem, especially if the post-thaw sperm are left longer before use in ART procedures.
Sperm DNA longevity can be conceptualized and partially modeled in the laboratory imitating what goes on in the female reproductive tract. The physiological temperature in the female reproductive tract is around 36°C, so if we examine changing values of SDF along a determined period of incubation at this temperature, we may detect genomes predisposed to assume higher levels of DNA damage than others do over time. With the level of resolution of the SCD technique it can be determined that SDF shows changing values after only two hours of incubation [46]. The rate of sperm DNA fragmentation (increase of SDF per time unit) is variable among individuals but is also highly dependent on the storage conditions or environmental influence. When thawed-cryopreserved samples are tested, an increase of SDF of about 8 percent in some individuals is appreciated after 24 hours of incubation. However, in other cases, this increase reaches 80 percent of the spermatozoa [46]. If the semen sample is to be used for IVF or IUI, the baseline level of SDF as diagnosed in the patient probably will change during co-incubation of intrauterine inoculation. In routine IVF, oocytes are co-incubated with sperm overnight. Some aspects about the negative impact of iatrogenic DNA damage were reported some years ago [48] using sperm samples that were delivered between different ART clinics. The main conclusion of that paper was that miscarriage improved after co-incubation of sperm-oocyte for short periods of time in those cases where sperm was delivered between different centers. It has been reported that this period of co-incubation may cause problems in normal embryo development. To avoid this trouble, short periods of co-incubation may be recommended to achieve better rates of fertilization in IVF conditions [48, 49].
While in humans, problems for embryo development related with an increased incidence of SDF occurring during co-incubation need to be demonstrated, we do have evidence of this situation in animal models. In the case of rabbits using the same females to be inseminated with males presenting high or low rates of SDF, it was found that the rate of stillborn pups was significantly higher in females inseminated by males with a high rate of SDF when compared to those with low rate of SDF. Moreover, the risk of stillborn animals was low when males with low rates of SDF were used. This risk increased dramatically after inseminating the same females with animals presenting with a high rate of SDF [50]. It is interesting to highlight, that using zebra-fish as an experimental model, when sperm DNA damage is controled under in vitro conditions and used for fertilization purposes, fertilization rates are less affected than the embryo viability rates [28]; this finding agrees with the high correlation of embryo loss associated with high levels of SDF reported in humans.
The concept of sperm DNA longevity can also be applied to donor sperm banks in order to select those individuals exhibiting the highest level of DNA stability. In a prospective study, it was found that donors exhibited a sperm DNA longevity 2.5 times higher than that observed in a cohort of patients. Static values of SDF after thawing indicated that a level of SDF of about 11 percent identify donors within a general population with 71 percent sensitivity and 84 percent specificity. On the other hand, a dynamic SDF increase of 2.3 units per hour might identify the donors with 70 percent sensitivity and 66 percent specificity [51].
22.6 Improving Sperm DNA Quality for Assisted Reproductive Technologies
The mere detection of increased levels of SDF is not paramount in solving the infertility problem, but once abnormal levels are detected, we may use different approaches to reduce these altered levels of SDF. These procedures open a new space in ART that are linked to the concept of personalized medicine since they not only try to identify the problem, but also to test and fix the most efficient methodology to obtain the most competitive sperm for fertilization associated to each patient. In a previous review, we have summarized some of the available strategies to reduce high levels of sperm DNA damage when observed in the neat ejaculate [52]. Some of these strategies are extremely useful because they are not invasive or based on supplements administration, but try to interpret and mimic what nature is doing at the time of ejaculation.
22.6.1 Recurrent Ejaculations
Ejaculatory abstinence duration is one of the non-invasive strategies that may allow us to decrease high levels of SDF. At the epididymis, there is a potential ROS capacity that may produce DNA damage; equally, active mechanisms of DNA repair are not available to the spermatozoon. Ejaculation abstinence for long periods before collecting sperm may seem like a way of increasing the proportion of good quality sperm, but this is likely to be a misleading strategy. In fact, we have shown that recurrent ejaculations every 24 hours for 72 hours resulted in a significant decrease in SDF [24]; other studies have observed an equivalent response of the ejaculate [53]. In these cases, DNA quality improved even further when these same samples were processed using density gradient centrifugation. This is most likely linked to the fact, that recurrent ejaculation produces an increase in immature sperm that are most efficiently selected using DGC because the cell surface area (including the cytoplasmic droplet) presented to gradient media is higher than that offered by compact mature sperm cells. As a general pattern, patients with a high level of SDF should undergo recurrent ejaculation every 24 hours for 3–4 days with a final period of abstinence of 12 hours. This can be combined with sperm selection using DGC and fertilization with ICSI. This combination results in a significant increase in pregnancy rate [54, 55].
22.6.2 Ejaculate Fractionation
Fractionation of the ejaculate is also a relatively simple strategy that may decrease the level of SDF observed at the whole ejaculate. In most clinics, the ejaculate to be processed for fertilization is not fractionated. However, a whole ejaculation consists of different semen fractions that are successively released during natural intercourse and they present different characteristics in composition with respect to their biological role [56, 57]. In those mammalian species so far studied, it is evident that the level of SDF is consistently lower in the first fraction when compared with that of the second fraction or in the whole collected ejaculate [58, 59]. Consequently, in cases where a high level of SDF is observed in a routine spermiogram, the use of the first ejaculated fraction, followed by the standard sperm selection techniques, is likely to produce a reduction in the levels of SDF.
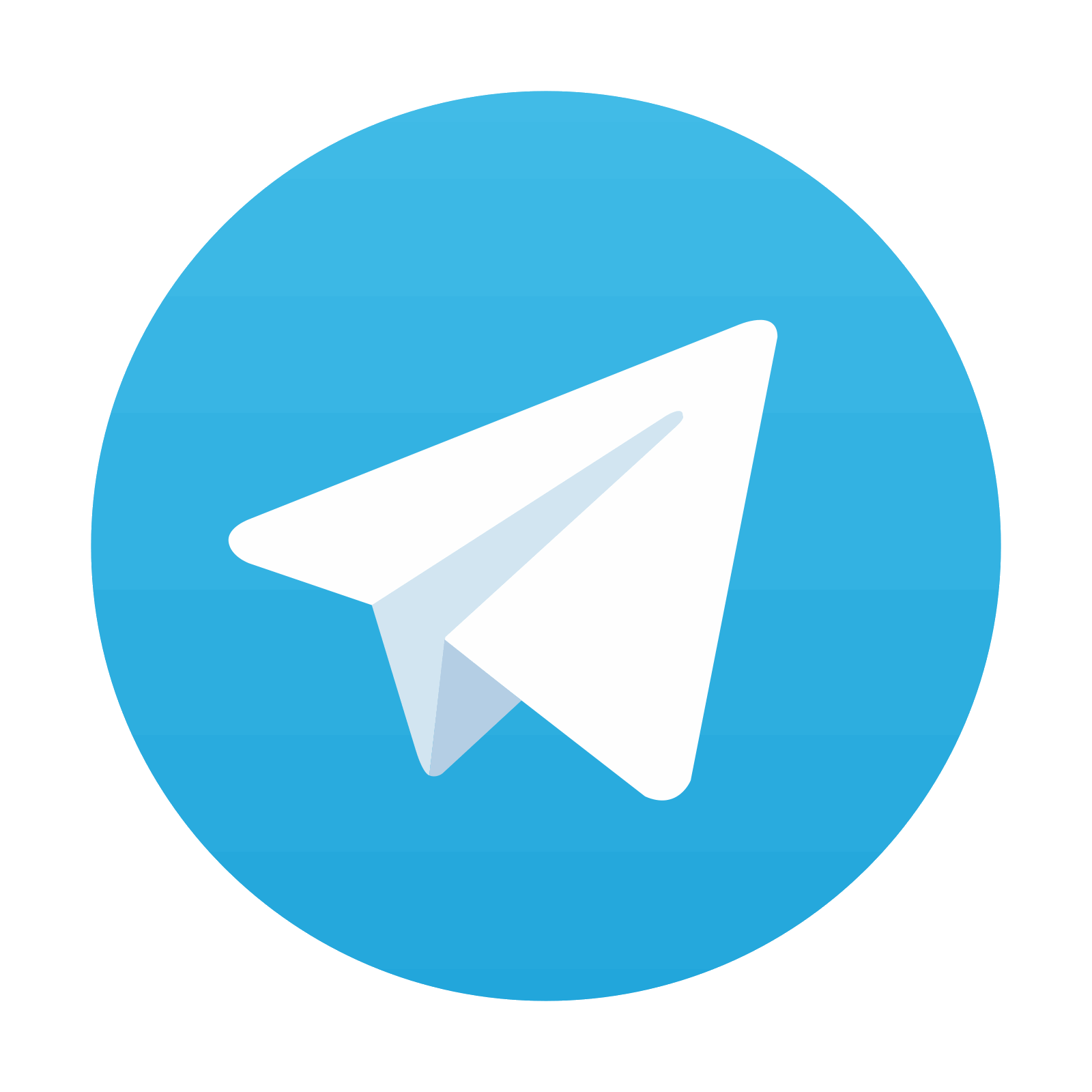
Stay updated, free articles. Join our Telegram channel
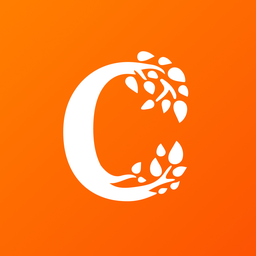
Full access? Get Clinical Tree
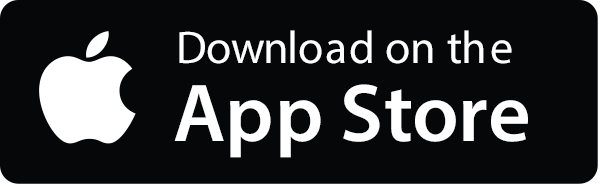
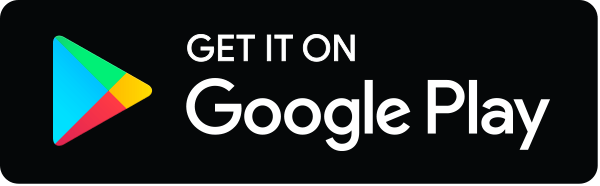