Important advances that enhance our understanding of the regulation of neuronal excitability in epilepsy and other brain functions have been made in molecular neurobiology. This research has typically involved antiepileptic drugs (AEDs) and other pharmacologic probes that have defined different mechanisms affecting neuronal excitability. The investigation of AEDs in these systems has also shed new light on the molecular basis of epilepsy and on the mechanisms of AED action (1–5).
This chapter provides the scientific background to assist the clinician in keeping abreast of several rapidly advancing fields. It highlights issues that influence neuronal excitability and seizures with emphasis on the mechanisms of sustained repetitive firing (SRF), sodium channels, the gamma-aminobutyric acid (GABA)–chloride channel complex, potassium channels, excitatory transmission, regulation of metabotropic receptors, carbonic anhydrase inhibition, neuromodulators, calcium-mediated regulation of neuronal function, and inhibition of epileptogenesis. In considering these quite different mechanisms, this chapter brings together many of the concepts considered in Chapters 4 and 40.
SUSTAINED REPETITIVE FIRING (SRF)
Sustained high-frequency repetitive firing is an important property of vertebrate and invertebrate neurons that correlates with the excitability state of the neuron (6–10). Many central nervous system (CNS) neurons exhibit SRF. Although no direct evidence has demonstrated the link between SRF and epilepsy, information from in vitro studies on isolated neurons may have some bearing on altered neuronal excitability and anticonvulsant action.
SRF is a nonsynaptic property of neurons. The relevance of limitation of SRF to AED action is strengthened by several important observations. The therapeutic efficacy of each AED in controlling seizures in animals and humans is similar to that for controlling SRF in isolated, cultured neurons. These results indicate (7) that therapeutic levels of AEDs in cerebrospinal fluid (CSF) correlate with the concentrations that are most effective against SRF. The effects of anticonvulsants in limiting SRF have been shown in a variety of neurons from different regions of the mammalian CNS maintained in culture. This argues that this effect is not unique to specific regions or cell types. Anticonvulsants prevent bursting in the epileptic focus and restrict the spread of epileptic activity from the focus to normal surrounding tissue. Thus, suppression of SRF by anticonvulsants may inhibit excitability by inhibiting the spread of seizure activity. Membrane properties of nonepileptic neurons may be important in understanding drug mechanisms of action and may have a special relationship to the properties of SRF. SRF is an important model for studying the excitability of isolated neurons.
SUSTAINED REPETITIVE FIRING AND SODIUM CHANNEL REGULATION
In studying SRF, several properties of neuronal excitability and seizure phenomenon have been recognized. Voltage-gated sodium channels are responsible for the rising phase of neuronal action potentials. Upon neuronal depolarization to action potential threshold, the sodium channel undergoes a conformational change that results in the channel opening for a few milliseconds from its closed (resting), nonconducting state to permit sodium flux. The channel inactivates within a few milliseconds to terminate sodium ion influx. The membrane must repolarize before it can be activated again by a subsequent depolarization. Brain sodium channels can rapidly cycle through the conducting and nonconducting stages, allowing neurons to fire tonic high-frequency action potentials, as is required for both normal brain function and for the expression of epileptic activity characterized by SRF. Several agents block sodium channels to produce an antiepileptic effect. They include phenytoin, lamotrigine, carbamazepine, zonisamide, felbamate, topiramate, and valproate. The AEDs that block SRF have several properties in common. Blocking of SRF is voltage-dependent, and the anticonvulsant effect is use-dependent. Research on the role of the sodium channel in regulating SRF indicates that the drug effects on SRF are mediated through a use-dependent blockage of the sodium channel. Thus, the block accumulates with SRF, inhibiting only the pathologic high-frequency discharges but not the excitatory or inhibitory synaptic processes essential for normal brain functioning (11). Phenytoin and carbamazepine can reduce the amplitude of the sodium-dependent action potential in a use-dependent fashion (12). Phenytoin is thought to induce a nonconducting state of the sodium channel that is similar to channel inactivation (11, 13). These studies indicate that modulation of sodium channels by anticonvulsants, and possibly endogenous anticonvulsant-like molecules, may play an important role in the regulation of neuronal excitability in seizure discharge. Newer compounds such as safinamide, which is in clinical trials, are thought to produce an anticonvulsant effect, at least in part, through inhibiting sodium and calcium channels, stabilizing membrane excitability, and inhibiting transmitter release (14). Another novel anticonvulsant, YKP-3089, is currently in phase-II clinical trials (NCT01866111). It acts as a selective blocker for the inactivated state of the sodium channel, preferentially blocks persistent sodium current, and is also thought to facilitate presynaptic GABA release. Together, these actions result in a potent inhibition of synaptic neurotransmission (15). Cannabidiol, the nonpsychotropic constituent of Cannabis sativa, has been reported to exhibit potent anticonvulsant action. It has been shown to preferentially suppress resurgent sodium channels (NaV 1.6)—a genetic mutation present in select pediatric refractory epilepsies (16).
Voltage-gated sodium channels activate (open) upon membrane depolarization and deactivate (close) upon repolarization. However, following continual depolarization, the channels enter an “inactivation” state where the channel is refractory and is unable to open for a prolonged period. This inactivation can be of two types: the fast inactivation (lasts for milliseconds) or the slow inactivation (lasts for seconds). These processes are critical to membrane excitability and, therefore, have an important role to play in seizures. Indeed, many human neuronal epileptic channelopathies have been reported in genes encoding voltage-gated sodium channels, particularly modification of inactivation kinetics. Inactivation processes are affected by chemical agents. For example, the antiepileptic drug lacosamide does not affect the fast inactivation of voltage-gated sodium channels but it targets the slow inactivation processes and enhances the maximal fraction of channels that are in the slow inactivated state (17). In this aspect, surprisingly, eslicarbazepine’s mechanism of action resembles lacosamide rather than carbamazepine (18). This unexpected finding that eslicarbazepine targets the slow inactivation of sodium channels over the expected carbamazepine-based inhibition of fast inactivation of sodium channels further elaborates the point that investigating the heterogeneity of sodium channels and their relationship to altered neuronal excitability is an area of important research.
BENZODIAZEPINE RECEPTORS AND MEMBRANE EXCITABILITY
In the 1970s, use of radioactively labeled benzodiazepine derivatives allowed the detection of specific nanomolar benzodiazepine receptor sites in brain membrane (19–21). These sites have a very high affinity for the benzodiazepines, binding in low (nanomolar) concentration ranges. Binding to these receptors is reversible, saturable, and stereospecific. Nanomolar benzodiazepine receptors have now been identified in the human brain, where they are widely distributed. The specific membrane protein that accounts for the majority of nanomolar benzodiazepine binding has a molecular weight of approximately 50,000 daltons and has been purified from animal and human brain (22).
High-affinity benzodiazepine binding has also been observed in peripheral, nonneuronal tissue (23). A different class of benzodiazepine receptor molecules causes this binding, because both the potency and the tissue distribution of this binding are different from that at the central-type receptor. This second class of high-affinity benzodiazepine binding sites was designated “peripheral type receptor” (23), but it was subsequently shown that the peripheral-type benzodiazepine receptor is also present in neuronal tissue. Thus, both peripheral and central high-affinity benzodiazepine receptors exist in the brain.
High nanomolar and low micromolar benzodiazepine binding sites have been identified more recently in brain membranes (24,25). In addition, another benzodiazepine receptor that binds in the high nanomolar range has been identified in brain cytosol (25). These novel benzodiazepine-binding sites are stereospecific and have potencies for benzodiazepine binding that correlate with the ability of these compounds to inhibit maximal electric shock-induced seizures. Benzodiazepine bound to micromolar benzodiazepine receptors is displaced by phenytoin. These results indicate that high nanomolar and low-micromolar-affinity benzodiazepine receptors may represent important anticonvulsant binding sites in the brain membrane that mediate some of the effects of benzodiazepines in high concentration in curtailing status epilepticus, generalized tonic–clonic seizures, and maximal electric shock-induced seizures.
Benzodiazepines are effective in nanomolar concentrations in blocking pentylenetetrazol-induced seizures in animals and in treating absence seizures. In addition, benzodiazepines in high nanomolar and low micromolar ranges inhibit maximal electric shock-induced seizures in animals. Furthermore, benzodiazepines are effective in humans in stopping generalized tonic–clonic seizures and status epilepticus when given in intravenous doses that produce low micromolar serum concentrations. The potency of the benzodiazepines in blocking maximal electric shock-induced seizures, however, correlates in neither time nor consequence with their ability to inhibit pentylenetetrazol-induced seizures or bind to the nanomolar central benzodiazepine receptor (24). Thus, other mechanisms appear to underlie this generalized anticonvulsant property of the benzodiazepines in high concentration ranges. Lower-affinity benzodiazepine binding sites in the high nanomolar and low micromolar ranges have also been described (24,26). These lower-affinity receptor sites come into play in the concentration ranges in which benzodiazepines produce effects on maximal electric shock-induced seizures in animals, on generalized tonic–clonic seizures in man, and on SRF in cultured neurons.
Diazepam and clonazepam reduce SRF in high nanomolar and low micromolar concentrations (7). These concentrations are above therapeutic free-serum concentrations achieved in ambulatory patients treated with benzodiazepines but are within the ranges of free-serum concentrations achieved in patients treated for status epilepticus or acutely for generalized tonic–clonic seizures. In addition to the discrepancy in concentration ranges, the potency of benzodiazepines for suppressing SRF does not correlate with benzodiazepine binding to the nanomolar central receptor or with their ability to inhibit pentylenetetrazol-induced seizures but does correlate with binding to the lower-affinity benzodiazepine binding system.
THE GABA SYSTEM, NEURONAL EXCITABILITY, AND SEIZURE ACTIVITY
Gamma-aminobutyric acid is the major inhibitory neurotransmitter in the brain. It has been extensively characterized and plays a major role in regulating neuronal excitability by controlling chloride permeability (27–29). Specific binding sites for GABA molecules have been identified in the neuronal membrane. Although not all GABA receptors are linked to the chloride channel, a large proportion of these receptors are directly involved in regulating chloride channel function. The major inhibitory effect of GABA on the nervous system is mediated through its ability to regulate chloride channel permeability. GABA binding to the GABA receptor potentiates the opening of the chloride channel, allowing chloride ions to flow more easily into the cell. This causes cellular hyperpolarization and inhibits neuronal firing, because chloride ions increase the internal electrical negativity of the cell. This is believed to be the major mechanism by which GABA produces its inhibitory effect on neurons.
Functions of GABA in neuronal systems have been closely linked to the effects of the benzodiazepines (27–29). Significant portions of the nanomolar benzodiazepine receptors in the brain are associated with the GABA-binding sites and are, thereby, functionally linked to the chloride channel in neuronal membranes. The GABA–nanomolar benzodiazepine receptor/chloride ionophore macromolecular complex is an important example of the interrelationship between membrane receptors and neuronal excitability (29). The ability of AEDs and the benzodiazepines to modulate chloride conductance is a major molecular mechanism regulating neuronal excitability.
The GABA–Chloride Channel Complex Regulates Seizure Discharge
The chloride channel is surrounded by a GABA receptor, a nanomolar central benzodiazepine receptor, and a receptor site that binds picrotoxin and related convulsants as well as barbiturates and related depressants. This channel and its properties, related to the benzodiazepines, GABA, and convulsant and barbiturate molecules, have been characterized in detail (30). Picrotoxin binds to the proposed site and modulates benzodiazepine and GABA receptor binding in a way that inhibits chloride channel permeability, thereby making the cell more excitable. Barbiturate binding potentiates benzodiazepine receptor binding and thus indirectly potentiates the GABA effect on opening the chloride channel and enhancing neuronal inhibition. This complex interaction between GABA, benzodiazepines, picrotoxin and related convulsants, and the barbiturates and related depressants is a prime example of how pharmacologic agents modulate the function of ion channels through specific receptor binding.
Benzodiazepines are an important class of compounds that have antianxiety or anxiolytic effects and sedative, muscle relaxant, and anticonvulsant properties (19,27–29,31). As anticonvulsants, these compounds are effective in blocking both pentylenetetrazol-induced seizures in animals and, at higher concentrations, maximal electric shock-induced seizures. Benzodiazepines are the most commonly prescribed drugs for the initial treatment of generalized tonic–clonic seizures and status epilepticus in hospital emergency rooms. The anticonvulsant properties of the benzodiazepines, therefore, are not only of academic importance but also have widespread clinical use. The research characterizing this benzodiazepine receptor (20–23,32,33) demonstrates AED receptor-mediated regulation of neuronal excitability. Benzodiazepine binding to the nanomolar receptor site potentiates GABA effects on neuronal inhibition, providing one clear mechanism by which these compounds regulate neuronal excitability. Correlative neuropharmacologic studies have indicated that the anxiolytic and antipentylenetetrazol-induced anticonvulsant activity of these compounds are mediated through the high-affinity benzodiazepine nanomolar receptors. The effects of benzodiazepines on maximal electric shock-induced seizures and on generalized convulsions, however, cannot be clearly explained by these nanomolar actions.
Stiripentol is a novel antiepileptic compound that is structurally unrelated to any of the other currently marketed antiepileptic drugs (34). Studies in animal models have demonstrated that it produces anticonvulsant effect by increasing GABAergic transmission by enhancing the duration of the opening of GABAA receptor channels (35). However, its clinical development was halted because of its inhibitory effect on cytochrome P450 enzymes. Despite this, it has been found to be beneficial for the treatment of early childhood epilepsy, and clinical trials are under way to further characterize its beneficial effect in pediatric patients (34).
Recently two types of GABAA receptor-mediated inhibition have come to the forefront: phasic and tonic. Synaptically released GABA, acting on postsynaptic GABAA receptors, produces “phasic” inhibition, whereas ambient GABA, which is continually present in the extracellular space, produces persistent activation of extrasynaptic GABAA receptors, resulting in “tonic” inhibition (36). Elegant studies by Istvan Mody’s group strongly indicate that distinct GABAA receptor subtypes mediate these tonic and phasic receptor-mediated inhibitions (36–38). They found that the alpha-4 GABAA receptor subtypes were extrasynaptically located and were responsible for tonic inhibition (38). These results suggested that alpha 4/beta/delta and alpha 4/beta/gamma subunits containing GABAA receptors may be present exclusively extrasynaptically, have a higher affinity for GABA, and do not desensitize on the prolonged presence of the agonist (39). On the other hand, alpha 1/beta/gamma 2 or alpha 2/beta/gamma 2 receptors are thought to mediate phasic inhibition, since the synaptic currents are known to be sensitive to benzodiazepines (40,41). These studies provide another mechanism for GABAA regulation of synaptic activity.
Neuroactive steroids are positive allosteric modulators of GABAA receptors (42). They exhibit both phasic and tonic aspects of GABAergic transmission neuromodulation (43). Allopregnenolone is being developed for the treatment of refractory status epilepticus (44,45), while the synthetic neurosteroid ganaoxolone is currently in clinical trials for the treatment of adult partial onset seizures (46).
GABAA– and GABAB-Mediated Neuronal Inhibition
As described previously, the best-characterized inhibitory effect of GABA on the nervous system is to open chloride channels and hyperpolarize the membrane, an effect that is mediated through GABAA receptors. In addition to GABAA receptors, scientists have characterized GABAB receptors that primarily open potassium channels in the membrane either pre-or postsynaptically. Any alteration or decrease in GABA-mediated inhibition through GABAA or GABAB receptors can lead to seizures. Thus, GABAB knockout mice exhibit spontaneous seizures (47). These data clearly indicate that GABA receptors in the regulation of chloride and potassium channels can regulate seizure discharge.
Potassium Channels
Potassium channels play a major role in regulating neuronal excitability. Although more than 20 types of potassium channels have been identified by biophysical studies, there are four major groups: calcium-activated, voltage-gated, sodium-activated, and inwardly rectifying potassium channels. These different types of potassium channels are regulated by neuromodulators, ions, and second messenger systems. The opening of potassium channels has the effect of hyperpolarizing neurons or reversing depolarizing actions that exist during the transmission of the action potential or the neuroexcitatory input. Following depolarization, several calcium-activated potassium channels play a major role in the after-hyperpolarization that occurs to restore the resting potential of a neuron (48). Electrographic studies on hippocampal neurons from patients undergoing temporal lobectomies have demonstrated alterations in potassium channels, particularly the delayed rectifier, Kv1.1 type in epileptic tissue (49). In addition, compounds that can block potassium channels and prevent the effect of these channels on hyperpolarization are potent convulsants. The possibility of developing new classes of compounds that can activate or potentiate potassium channel activity may play an important role in increasing hyperpolarization following excessive excitatory activity and may serve to reverse the decreased levels of some potassium channels observed in patients with epilepsy.
Some anticonvulsant compounds may also play a role as potassium channel openers. Carbamazepine has been found to enhance potassium conductance in neurons (50). Other potential anticonvulsant compounds that may also potentiate potassium channel activation are being evaluated. Investigation of anticonvulsant drugs regulating potassium channels is a major frontier in the development of new anticonvulsant compounds. The small conductance Ca2+-activated K+(SK) channels inhibit epileptiform bursting in hippocampal CA3 neurons. Compounds activating or inhibiting voltage-gated potassium channels formed by KCNQ2, KCNQ3, and KCNQ5 assembly (M-channels) are undergoing clinical trials for epilepsy, stroke, and Alzheimer disease (51). One such compound, Ezogabine (retigabine), was approved as add-on treatment of partial seizures in adults by the U.S. FDA in June 2010 (52). Mutation in KCNQ2/3 causing mild reduction of M-channel activity is thought to enhance neuronal excitability associated with benign neonatal seizures. On the other hand, M-channel openers decrease the hyperexcitability responsible for epileptic seizures and migraine. Indeed, retigabine is thought to produce an antiepileptic effect by opening the KNCQ2/3 channels (53,54). This is a promising area for the development of new anticonvulsant drugs (55).
It is only in the recent past that scientists have begun to decipher the role of the hyperpolarization-activated cation (HCN) channel or h-channel (Ih) in regulating neuronal excitability and modulating seizures. The HCN family of genes consists of four subtypes and encodes HCN or Ih (56). HCN1 and HCN2 are the most prevalent subtypes in the cortex and hippocampus, and HCN2 and HCN4 predominate in the thalamus. HCN3 is modestly expressed in the brain (57). HCN has the unique capacity of being able to produce opposite biophysical effects. Thus, it can be either excitatory or inhibitory with respect to its influence on action potential firing. For example, Ih can diminish the effect of excitatory inputs, or it can set the normal resting membrane potential, depolarizing the membrane from the K+reversal potential toward the firing threshold, thereby producing an excitatory effect (58). There is a growing body of evidence implicating HCN in epileptogenesis (58–61). For example, Ih– and GABA-mediated inhibition are increased in febrile seizures (62,63). Moreover, genetic deletion of HCN2 resulted in a mouse phenotype that exhibited spontaneous absence seizures as well as cardiac sinus arrhythmias, indicating that the absence of HCN2 is proconvulsive (64). There is conflicting pharmacologic evidence for the role of HCN in epilepsy (58). Certain AEDs, such as lamotrigine and gabapentin, act beyond their normal target—the Na+ channels—and upregulate Ih, and they reduce action potential firing that is initiated from dendritic depolarization and not from somatic depolarization (59,65). On the other hand, other Na+ channel blockers, such as carbamazepine or phenytoin, did not lower dendritic excitability (59). Thus, the action of lamotrigine on HCN may constitute an important, novel anticonvulsant mechanism. The role of the HCN channel in epilepsy is an important area for further investigation.
EXCITATORY TRANSMISSION
Glutamate is the major excitatory neurotransmitter in the brain. Understanding the role of excitatory transmission and its over-activation in epilepsy is an important area for anticonvulsant drug development. Only since the early 1980s has the role of glutamate, aspartate, and other compounds that serve as excitatory transmitters been clearly identified. Several important receptors, which respond to glutamate and other excitatory neurotransmitters, have been identified in the brain (66). The major glutamate receptors that regulate ion channels include the N-methyl-D-aspartate (NMDA), quisqualate or alpha-amino-5-methyl-3-hydroxy-4-isoxazolepropionic acid (AMPA), and kainate channels. In addition, there are other, more recently identified subcategories of excitatory amino acid ion channels regulated by glutamate (67).
NMDA receptor-regulated ion channels are an important class of excitatory amino acid coupled channels in the brain that not only play a role in neuronal excitability but also have long-term effects on long-term plasticity changes in the brain. NMDA receptor-activated channels are permeable to sodium, potassium, and calcium. They can be voltage-blocked by magnesium, which indicates that they can be in an operative form only when the cell is partly depolarized by other excitatory receptor activation. This type of modulation allows for complex regulation of NMDA receptor activity. For an NMDA channel to be activated, it is necessary for either the excitatory amino acid transmitter glutamate or aspartate to bind to the NMDA receptor site and for the molecule glycine or D-serine to bind at the neuromodulatory site on the receptor. Antagonists of either glutamate or glycine at their respective recognition sites can block this channel. Regulation of NMDA receptors may play an important role in the development of anticonvulsant drugs. In addition, long-term changes in NMDA channel expression may accompany epileptogenesis. Properties of NMDA receptor channels are altered in neurons from kindled rats (68). Furthermore, NMDA receptor antagonists are anticonvulsants in several models of epilepsy (69). Glutamate (70) and glycine site competitive antagonists (71,72) also have anticonvulsant activity. Noncompetitive channel blockers that work when the channel is open can also inhibit NMDA channels. These drugs include dizocilpine (MK-801) and phencyclidine. Although both of these compounds are good anticonvulsants, both have major side effects when used in anticonvulsant doses (73). Nevertheless, the possible role of glutamate/NMDA-channel regulation is an important area for the development of anticonvulsant compounds and those that may prevent epileptogenesis. Although there is a plethora of evidence from preclinical in vivo and in vitro models of epilepsy regarding the AED potential for glutamate receptor blockers, use of selective NMDA agents as AEDs has been rather disappointing in the clinical setting. However, there is evidence that some of the clinically used AEDs, such as felbamate, can block NMDA receptors at therapeutic concentrations (74).
AMPA receptors are major excitatory amino acid receptors that play an important role in the fast depolarizing actions of glutamate, contributing to the fast excitatory transmission in the CNS. They are thought to be essential for seizure spread and are thus attractive targets for development of novel AEDs. During epilepsy, the AMPA receptors are responsible for the early component of the discharges involved in spike discharges and paroxysmal depolarizing shifts. The later elements of these phenomena are primarily related to NMDA receptor activation. Quinoxalinediones (75) were one of the first AMPA receptor antagonists that were found to have anticonvulsant properties in several models of epilepsy (70,76). Several classes of AMPA channel inhibitors, which have anticonvulsant activity, have also been developed. Both indirect AMPA antagonists and noncompetitive allosteric inhibitors of AMPA receptors have anticonvulsant property in several animal models (76). The AMPA receptor has several molecular subtypes that are differentially expressed in different neurons (77). Receptors containing the GluR2 subunit have a much lower permeability to calcium and show a component of their activation that has a linear rectifying current–voltage relationship. Receptors lacking the GluR2 subunit are different and show significant calcium permeability and an inwardly rectifying property (78). Evidence indicates that differential expression of the GluR2 subunit may play an important role in epileptogenesis and altered neuronal excitability. Thus, the ability to selectively modify AMPA receptors containing GluR2 subunits may play an important role in the development of anticonvulsant compounds. Selective AMPA receptor antagonists following intense investigation as potential AEDs were recently approved for the treatment of partial onset seizures (79,80).
Kainate channels also play an important role in fast depolarizing actions of glutamate in the CNS. However, only a few drugs have been identified as primary kainate antagonists, although some of the AMPA antagonists also inhibit kainic acid channels (81). At the present time it is not clear whether selective inhibition of kainate channels has any advantage in terms of anticonvulsant development over the development of AMPA channel inhibitors. Sodium ion-permeable kainate receptors not only contribute to the postsynaptic excitation, but the presynaptically located kainate receptors can modulate release of glutamate from excitatory terminals and, interestingly, can also prevent release of GABA from inhibitory interneurons (82). Kainate receptor antagonists have been demonstrated to block seizures in the in vivo and in vitro epilepsy models (83). In fact, among topiramate’s multiple actions that contribute toward its being a potent AED, kainate receptor blockade is a participating mechanism of action (84,85). The possible role of kainate glutamate receptors in modulating seizure discharge and anticonvulsant drug development is an important frontier for further research.
METABOTROPIC RECEPTORS
Metabotropic receptors are glutamate- or excitatory amino acid-activated receptors that are not coupled to ion channels. These excitatory amino acid receptors are coupled to second messenger systems in the membrane that have an important role in regulating cellular metabolism and function (86). Metabotropic receptors are coupled to G proteins and enzymes that regulate adenylate cyclase or guanylate cyclase. Regulating these enzymes by metabotropic receptor activation changes levels of cyclic adenosine monophosphate (cAMP) or cyclic guanosine monophosphate (cGMP). Metabotropic glutamate receptors are classified into eight different types (mGluR1–mGluR8). These mGluRs are further classified according to the second messenger systems to which they are linked. Class I mGluRs, consisting of mGluR1 and mGluR5, are most potently activated by quisqualate and are coupled to phospholipase C (PLC) activation. Class II mGluRs, consisting of mGluR2 and mGluR3, are activated by 2R,4R-4-aminopyrrolidine-2–4-dicarboxylate (APDC), and inhibit adenylate cyclase activity. Class III mGluRs (mGluR4 and mGluR6–mGluR8) are most potently activated by l-amino-4-phosphonobutyrate and inhibit adenylate cyclase activity, but to a lesser extent. Metabotropic glutamate receptors are found both on the presynaptic and postsynaptic membranes. Presynaptic mGluRs decrease neurotransmitter release, while mGluRs on the postsynaptic membrane regulate the function of ligand-gated ion channels, including all three subtypes of ionotropic glutamate receptors, as well as inhibit the function of voltage-gated Ca2+ channels (VGCCs) and some potassium channels. Thus, metabotropic glutamate receptors can act to modulate synaptic transmission in the CNS (87). Modulating the activation or inhibition of metabotropic receptor activation by excitatory transmission plays an important role in modifying neuronal function over both short- and long-term periods relative to seizure activity. Metabotropic receptors play an important role in producing sustained changes in neuronal excitability that may have implications in epileptogenesis and the development of seizure discharges. In patients with temporal lobe epilepsy (88) or focal cortical dysplasia (89), upregulation of mGluR5 immunoreactivity has been described. In the pilocarpine-induced epilepsy model, mouse strains with differential susceptibility exhibit different expressions of mGluR4 receptors, with up-regulation in the dentate gyrus of mice with attenuated seizure phenotype and downregulation in mice with enhanced seizure susceptibility (90). Evidence has accumulated for a possible role of glutamate metabotropic receptors in the development of epileptogenesis (91). Inhibition of metabotropic receptor activation during epileptogenesis in several models has blocked the development of long-term epilepsy. Competitive antagonists of group I mGluRs have shown efficacy against seizures induced experimentally in several epilepsy models. Metabotropic receptor inhibitors, therefore, are possible future areas for the development of novel AEDs (90,92,93).
CARBONIC ANHYDRASE INHIBITION
Carbonic anhydrase (CA) is a major enzyme system that has been found to regulate GABA-mediated inhibitory potentials and therefore has important anticonvulsant or antiepileptic effects (94). GABA receptor ion channels are permeable to both chloride and bicarbonate ions. Bicarbonate ions normally move outward through the GABA receptor, producing a depolarizing effect that is normally smaller than the hyperpolarizing action of the inward movement of chloride ions. The movement of bicarbonate ions out of the neuron, following entry of chloride ions, can produce a potential depolarizing effect that can be mediated by the GABA-receptor channel. Inhibitors of CA play a role in blocking this effect. Thus, blocking the depolarization effects mediated by the GABA receptor through bicarbonate ion movement may be an important role of CA inhibitors in increasing the hyperpolarizing effect of the GABA receptor in the neuron. There may be other actions of CA inhibitors that go through other mechanisms, but this is another area of research for the development of anticonvulsant drugs. CA inhibitors such as sulthiame and acetazolamide (AZM) have previously been shown to lower neuronal intracellular pH (pHi), which effectively reduced epileptiform activity in epilepsy model systems in vitro (95). Topiramate (96) is shown to produce carbonic anhydrase inhibition that reduces the steady-state pHi of cornu ammonis field 3 (CA3) neurons in slices, thereby inhibiting epileptiform activity.
NEUROMODULATORS
There are many classes of neuromodulators, but two major classes have been widely studied that have significant implications in regulating seizure activity: adenosine and the monoamines. Both adenosine and monoamines influence seizures and epileptogenesis in several models of epilepsy. Adenosine is released during seizure activity and acts as an endogenous anticonvulsant (97,98). One form of the adenosine receptor (A1 receptor) is coupled to the G protein system in a similar mechanism, as is the metabotropic receptor. Activation of the A1 adenosine receptor stabilizes the resting membrane potential presynaptically and blocks glutamate release but not GABA release (99). Several analogs of adenosine acting at the A1 receptor exhibit good anticonvulsant effects in several models of epilepsy (100,101). These compounds, however, have significant adverse cardiovascular effects, and their potential role as anticonvulsants needs further investigation. A ketogenic diet (KD) has been used with variable success in pediatric and therapy-resistant epilepsy (102). This alternative metabolism-based epilepsy treatment has multiple probable mechanisms of action (103). One such mechanism of action for KD is thought to be enhanced adenosinergic tone following A1 receptor activation. Adenosine is synthesized by ATP hydrolysis, and increased ATP levels following KD is thought to underlie the antiepileptic effect of KD (104). Another major type of adenosine receptor is the A3 receptor. Selected antagonists of the A3 receptor have also been recently shown to have anticonvulsant activity (105).
Regulation of adenosine receptors, both chronically and during seizure activity, may play important roles in developing new anticonvulsant drugs. The major challenge in this area, however, is to develop compounds that do not have complicating side effects, such as cardiovascular alterations or effects on other aspects of the nervous system.
Monoamines are important classes of neurotransmitters that play important roles in modulating both excitatory and inhibitory neurotransmission. Abnormalities in monoaminergic transmission in several animal models and in human epileptic foci taken from patients following epilepsy surgery have indicated that there may be a role for monoamine receptor activation in chronic epilepsy. Pharmacologic manipulation of monoaminergic neurotransmission plays a role in the development of several seizure syndromes, such as the reflex epilepsies (106). Noradrenaline agonists, such as alpha2 agonists, are protective against seizure activity, and antagonists act as convulsant compounds. These effects have been well studied in amygdala kindling in rats and kittens (107,108). In contrast, alpha2 agonists have been reported to exacerbate spike–wave discharges in both GAERS as well as WAG/Rij absence seizure rat models (109,110). Dopamine antagonists have also been shown to be protective in photosensitive epilepsy in animals and humans (111,112). Monoamine oxidase B inhibitors play a role as anticonvulsants in several seizure models.
There is a growing body of evidence implicating the serotonergic system in the etiology of seizures. For example, depletion of brain 5-hydroxytryptamine (5-HT, serotonin) levels by p-chlorophenylalanine (113) and the serotonergic neurotoxin 5,7-dihydroxytrpytamine (114) appears to exacerbate seizures, indicating that experimental manipulations that attenuate serotonergic neurotransmission can induce and/or augment epileptic seizures. In contrast, experimental manipulations that enhance serotonergic function, such as treatment with 5-HTP (115), fluoxetine (116), or stimulation of dorsal raphe nuclei (117–119) has been shown to inhibit seizures. In light of these findings, the role of 5-HT receptor subtypes is being actively investigated in epilepsy. Some clinicians consider vagus nerve stimulation (VNS) as an alternative in drug-resistant epilepsy. It is only in the recent past that we have begun to understand the mechanisms underlying VNS-mediated seizure suppression. One of the best indications regarding VNS’s mechanisms of action has come from Krahl et al (120). They chemically lesioned the locus coeruleus (LC) to chronically deplete rats of norepinephrine or acutely inactivated LC with lidocaine. Under both circumstances, VNS-induced seizure suppression was attenuated when tested in the maximal electroshock (MES) model. These studies indicate that the LC was necessary for the VNS-mediated anticonvulsant effects. Their studies also suggest that noradrenergic agonists could enhance VNS-induced seizure suppression. Thus, the possible role of monoamines in relation to monoamine receptors in epilepsy is another potential area for development of future anticonvulsants.
CALCIUM REGULATION OF NEURONAL FUNCTION
Calcium plays a major role in modulating the normal activity and function of the nervous system (121,122). One of its most widely recognized roles is modulating synaptic neurotransmission. A host of studies have demonstrated the importance of calcium in stimulus-secretion coupling (123). In addition to its important effects on neurotransmission, calcium plays a major role as a second messenger in neuronal and nonneuronal tissues.
Several lines of evidence indicate calcium’s importance in regulating neuronal excitability and producing anticonvulsant effects. Because of calcium’s importance as a second messenger, it is reasonable to assume that alterations in the normal function of calcium-regulated processes may underlie some of the abnormalities of neuronal excitability seen in seizure disorders.
Accumulating evidence suggests that abnormalities in major calcium-regulated enzymatic processes or ion channels underlie alterations in neuronal excitability and result in seizure activity (124). The role of calcium in antiepileptic drug action and in regulating seizure excitability has been recently reviewed (124,125). Certain anticonvulsants have been shown to regulate the entry of calcium into cells through both voltage-regulated and transmitter-regulated calcium channels. For example, anticonvulsants like gabapentin and pregabalin bind to alpha-2-delta (2) subunit of the voltage-dependent calcium channels to modify the calcium conductance (126,127). In addition, anticonvulsants inhibit important calcium-mediated enzyme systems that play important roles in cell function and neuronal excitability. These mechanisms may also be significant for some anticonvulsant effects.
Calcium Channels and Neuronal Excitability
The entry of calcium into a cell triggers many biochemical and biophysical actions (128,129). This major second-messenger effect of calcium has been clearly linked to the regulation of neuronal excitability and cell metabolism (98,129,130). Thus, controlling calcium entry into the cell is the first major step in regulating the effect of calcium as a second messenger.
Depolarization-dependent action potentials are typically mediated by large sodium currents into the cell. Calcium simultaneously enters the cell during depolarization. Recently, the importance of this calcium entry during action potential generation has been more clearly understood. Accumulation of increased concentrations of calcium within a neuron is related to SRF of neurons, which can occur in vitro or during epileptic activity. Calcium entry is also regulated by specific excitatory amino acid receptors. This type of calcium channel is opened or closed in response to binding of excitatory amino acids (EAA) to specific calcium channel–linked receptors. The ability of these channels to produce tonic, long-lasting excitability changes in hippocampal neurons and in other cortical neurons has implications for long-term potentiation, memory, and excitability.
In conceptualizing the role of calcium in neuronal excitability and anticonvulsant drug action, one must consider both voltage-regulated and excitatory amino acid-modulated calcium channels. The regulation of calcium channels, like the regulation of the chloride channel, by the benzodiazepines, barbiturates, and convulsant drugs may play an important role in modifying neuronal excitability.
Voltage-Gated Calcium Channels
Voltage-regulated or gated calcium channels affect neuronal excitability. As an action potential arrives at a nerve terminal, depolarization of the nerve terminal membrane causes entry of calcium through voltage-gated calcium channels with subsequent release of neurotransmitters. This is the classic paradigm for calcium-mediated neurotransmitter release and was the initial observation that demonstrated the importance of calcium in neuronal excitability, but little was understood about the specific mechanisms of calcium channels in the brain.
Early insight into the neuropharmacology of calcium channels came from studies of smooth muscle and cardiac cells during the 1970s. The dihydropyridine type of calcium channel blocker and related molecules were shown to be effective in blocking calcium channels in peripheral tissue (125,131,132). These compounds were classified as “organic calcium channel blockers” and represented a major advance in pharmacology. Numerous analogues were developed, and specific binding sites for the dihydropyridines and other analogs were identified and characterized. This led to the first molecular characterization of calcium channels and their regulation by specific receptor sites. A major controversy developed based on the observation that the organic calcium blockers, effective in inhibiting calcium entry into nonneuronal tissue, seemed to be ineffective in blocking voltage-gated calcium entry into neurons (133). Numerous investigators demonstrated that calcium entry as a result of neuronal activity was not significantly inhibited by therapeutic or relevant concentrations of the organic calcium channel blockers (125). Because there was at that time no clear evidence for more than one type of calcium channel, this dichotomy was not well understood and was attributed to the unusual properties of the neuronal membrane and to specific differences in drug penetration into the nervous system. More recent studies using benzodiazepines and phenothiazines (134–137) demonstrated that these compounds in fact could significantly block voltage-gated calcium channels in neurons. These results indicated that calcium channels in the brain were distinct from calcium channels in peripheral tissue.
With the development of patch and voltage clamp technology, more sophisticated characterization of calcium channels has been possible. It is now clear that there are at least three, and possibly more, types of calcium channels in neurons (133,138,139). One type of voltage-gated calcium channel is insensitive to dihydropyridines, while a second type of calcium channel is sensitive to these compounds. A third type of brain membrane calcium channel has also been postulated that is different from the first two types of channels. Although a set terminology has not been developed for these different channels, initial classification by Tsien (139) is currently in use and describes these channels as the T-, N-, and L-type channels, respectively.
The heterogeneity of calcium channels provides a major insight into different mechanisms for regulating calcium excitability in the nervous system. These observations also explain the fact that the major voltage-gated calcium channels in the brain that were insensitive to dihydropyridines were a different class of channels from those found in peripheral tissues. In certain regions of the nervous system and at certain sites on the cell body, however, there are calcium channels that are sensitive to dihydropyridines and are similar to those calcium channels in nonneuronal tissue. The different types of calcium channels are distributed in characteristic patterns over the surface of a neuron. Some channels may be localized at the synapse, while others may be present at a higher density at the cell body. The heterogeneity and individual functions of specific types of calcium channels are important areas for further investigation, such as the development of specific drugs to regulate each type of channel.
Several anticonvulsant compounds block or alter calcium entry through voltage-gated calcium channels. Ferrendelli and coworkers (140,141) described the ability of phenytoin, phenobarbital, carbamazepine, but not ethosuximide, to block voltage-dependent calcium entry into isolated nerve terminal preparations. Subsequent studies have demonstrated that benzodiazepines, as well as phenytoin and barbiturates, can regulate calcium entry into isolated neurons. However, the concentrations of antiepileptic drugs that are required to block calcium entry are in the low micromolar range, concentrations that are approximately one order of magnitude higher than the therapeutic levels of these drugs achieved in spinal fluid. Although these concentrations may be relevant to anticonvulsant actions, they are more likely related to toxic side effects.
Thalamic relay neurons fire in two different modes: burst and tonic. T-type Ca2+channels underlie the burst firings. When activated, T-type channels produce low-threshold Ca2+ currents that lead to the generation of a burst of action potentials (142). Burst firing of thalamic neurons have been implicated to play an important role in the pathogenesis of absence epilepsy (143). Ethosuximide, a known antiepileptic drug, is known to block T-type Ca2+ channels in thalamic relay neurons (144). Analysis of knockout mouse for the alpha1G gene coding for a subtype of T-type Ca2+ channels demonstrated absence of low-threshold, T-type, Ca2+ currents and an inability to induce burst firing in the thalamic relay neurons (145,146). Alpha1G knockout mice were resistant to GABAB receptor agonists, baclofen- and gamma-hydroxybutyrate (GHB)-induced seizures (147). T-type Ca2+ channels represent a novel pharmacologic target for development of drugs for the treatment of epilepsy.
Excitatory Amino Acid Receptors and Calcium Channels
L-glutamate was proposed as an excitatory neurotransmitter over 30 years ago (148). Recently, excitatory amino acids (EAA) have been found to play important roles in epilepsy, neuronal excitability, and learning (148–150). The two main excitatory neurotransmitters currently known are glutamate and aspartate (151). Many pathways in the brain use these neurotransmitters, including hippocampal afferents and major cortical output tracts that are widely activated during convulsions.
Excitatory amino acids bind to specific membrane receptors (66,152). Currently, four major types of EAA receptors have been characterized. One major EAA receptor is characterized by binding of the EAA analog NMDA. The NMDA receptor has now been well studied and represents a specific type of excitatory amino acid receptor. Three types of non-NMDA receptors that bind other analogs of EAAs but have different properties from the NMDA receptor have also been identified. The non-NMDA receptors bind EAAs but not NMDA. The non-NMDA receptors include binding sites for kainic acid, quisqualate, and 2-amino-4-phosphonobutyrate (2-APB).
Excitatory transmission regulated by EAA receptors plays a major role in synaptic transmission in the CNS. Excitatory amino acids regulate specific ion channels that allow calcium and sodium to enter the cell when the channel is activated by the EAA receptor (66,152). These specific channels are actually opened or gated by the EAA. The currents activated by EAA receptors are both rapid and long lasting. The postsynaptic localization of these receptors, as well as their presence over the cell body, have been implicated in explaining how EAAs alter neuronal excitability, causing rapid depolarization in some situations and long-lasting neuronal changes in other cells. Thus, this type of calcium entry is activated by specific EAAs and has been implicated in many neuromodulating effects in the brain. Important convulsants, such as kainic acid, alter neuronal excitability by binding to these receptor sites and activating calcium and sodium channels. Compounds that bind to EAA receptors but do not activate the channel can inhibit EAA effects on these receptors. Several of these compounds have potent anticonvulsant actions and are very effective in maximal electric shock-induced seizure models (66).
Because of their potential importance to epileptogenesis, EAA receptors and the calcium channels that they regulate have been the focus of extensive research (149,153). These receptor sites are responsible for mediating some of the effects of kainic acid in producing convulsant discharge in the brain. NMDA receptors have been implicated in the phenomenon of long-term potentiation and in long-term alterations of neuronal excitability. The EAA analog 2-amino-7-phosphonoheptanoate (APH, AP7) is a potent anticonvulsant in various seizure models. These and other investigations provide strong evidence that EAA receptors and their regulation of calcium channels are important mechanisms in regulating neuronal excitability and, potentially, in AED actions. It is anticipated that several new anticonvulsant compounds will be developed that act specifically through this mechanism.
Modulating the Calcium Signal in Controlling Neuronal Excitability
The discovery of a calcium-binding protein, calmodulin (CaM), was the first major breakthrough in understanding the molecular mechanisms that mediate calcium second messenger effects (154,155). Evidence now suggests that many of the effects of calcium on cell function are mediated by calmodulin (135,136,156–158). Several important calcium-regulated processes are mediated by calmodulin and by a major calmodulin target enzyme system, calmodulin kinase II (159–161). Evidence from several laboratories has now confirmed the original calmodulin hypothesis of neurotransmission and substantiated the role of calmodulin in mediating some of the effects of calcium on neuronal excitability. Antiepileptic drugs, including phenytoin, carbamazepine, and the benzodiazepines, antagonize calcium-mediated effects and inhibit calmodulin activation of calmodulin kinase II (158–162). Concentrations required to inhibit CaM kinase II are in the low micromolar concentration ranges for antiepileptic drug effects on the protein kinase. This enzyme plays an important role in mediating calcium-dependent protein phosphorylation of membrane and soluble proteins.
CaM kinase II has been implicated by several investigators to be a major molecular mechanism mediating some of the second-messenger effects of calcium in the cell. Thus, control of this important calcium-mediated event by phenytoin, carbamazepine, and diazepam may be a major action of these drugs. The precise relationship of this particular effect to clinically useful anticonvulsant activity remains to be elucidated.
The importance of calmodulin kinase II in regulating neuronal excitability is widely recognized. Injection of CaM kinase II into invertebrate neurons regulates potassium and calcium currents (163). In addition, CaM kinase II levels in hippocampal neurons are chronically altered during the long-term alteration of neuronal excitability that occurs in kindling (164). CaM kinase II activity is inhibited by specific anticonvulsants (124,136), and the subunits of CaM kinase II are a major protein component of the postsynaptic density, localizing this important enzyme system directly at the synapse (165,166). Further understanding of the role of CaM kinase II in the pathophysiology of epilepsy and in controlling neuronal excitability is clearly important.
Another major molecular mechanism regulating the effects of calcium on neuronal excitability and cell function is the major enzyme system protein kinase C. Protein kinase C has been implicated in many of the effects of calcium on cell function and has been implicated in some of the effects of calcium in regulating specific ion conductance (121). Although no direct studies have been performed to investigate the effects of anticonvulsant drugs on the C-kinase system, this too is an important area for investigation. Modulation of these calcium target enzyme systems by anticonvulsant drugs is a potential area for new drug development.
Calcium is a ubiquitous signaling molecule with significant regulatory function in neurons (167,168). As a result, even the slightest changes in [Ca2+]i could sustain alterations in Ca2+-dependent enzyme systems that could contribute to the generation of epileptiform activity. It has been demonstrated that the levels of protein kinase C (alpha) isozyme are decreased after kindling epileptogenesis (169), and the activity of the Ca2+/calmodulin-dependent phosphatase calcineurin is increased after pilocarpine-induced status epilepticus (SE) (170,171). Further, the activity of CaM kinase II is depressed in human epileptic tissue (172) and in various in vivo models of epilepsy (173–177). Epileptic neurons in culture also manifest some of the biochemical, electrophysiologic, and molecular changes observed in animal models. Thus, decreased CaM kinase II activity and its alpha subunit expression are observed in the low-Mg2+ hippocampal neuronal culture model of epilepsy (178). As in epileptogenesis, these changes are dependent on activation of the NMDA receptor (177,178). Further, mice that lack active CaM-KII manifest epileptiform activity after subconvulsive stimulation (179), implicating decreased CaMKII activity in epileptogenesis. In addition, hippocampal neuronal cultures, treated with antisense oligonucleotides to the CaMKII alpha subunit, express decreased levels of CaMKII and exhibit epileptiform discharges similar to the low-Mg2+ and glutamate injury-induced epileptogenesis models (180,181).
Anticonvulsants That Inhibit Vesicular Neurotransmitter Release
SV2A, a synaptic vesicle protein, has recently been identified as a likely target for antiepileptic action. SV2A is a protein component of synaptic vesicles that is structurally similar to 12-transmembrane-domain transporters. SV2A is not essential for synaptic transmission, but mice, in which the protein has been deleted by gene targeting, exhibit seizures (182). This finding led to a search for compounds acting on SV2A as probable anticonvulsant agents. Levetiracetam (Keppra®) is thought to bind with SV2A and disrupt synaptic release mechanisms to produce an anticonvulsant effect (183). It is also speculated that levetiracetam binding enhances a function of SV2A that inhibits abnormal bursting in epileptic circuits, a function whose loss in the SV2A knockout models results in seizures (183). It is also suggested that a reduction of potassium currents may contribute to the antiepileptic effect(s) of levetiracetam (184). A newer analog of levetiracetam, brivaracetam, is currently being investigated for probable anticonvulsant actions (185). Levetiracetam, at clinically relevant concentrations, is also reported to inhibit Na+-dependent CWHCO3 exchange, lower neuronal pH, and thereby contribute to its anticonvulsive activity (95). Brivaracetam, currently in phase-III clinical trials, has approximately 20-fold higher affinity for SV2A than levetiracetam. It has a faster onset of action due to its greater CNS permeability (186–188).
Anticonvulsants That Target the Endocannabinoid System
Recently the endocannabinoid system—an inhibitory neurotransmitter system—has generated considerable interest as a novel locus of anticonvulsant activity in the brain (189–192). This unique system consists of at least two cannabinoid receptors (CB1 and CB2), the endogenous agonists (endocannabinoids: anandamide and 2-Arachidonylglycerol (2-AG)), and the protein machinery for their synthesis, transport, and degradation (193). Cannabinoids such as those naturally occurring in marijuana (Cannabis sativa) possess anticonvulsant properties (194,195) and have been used since antiquity for the treatment of seizures (196). Recently synthetic cannabinoids such as WIN 55,212–2 have been demonstrated to produce an anticonvulsant effect in a CB1 receptor-dependent manner (197,198). Emerging evidence has indicated that the endocannabinoid system controls seizure threshold, duration, and seizure frequency (189,199).
Endocannabinoids regulate neuronal excitability via depolarization-induced suppression of excitation (DSE) (200) or inhibition (DSI) (201,202). In response to Ca2+-mediated depolarization of the postsynaptic membrane, endocannabinoids are synthesized “on demand,” and they diffuse in a retrograde fashion and activate presynaptic CB1 receptors to inhibit neurotransmitter release and thus modulate neuronal excitability (203). Intense synaptic activity caused by seizure discharges stimulates 2-AG synthesis (189). Such an “on-demand” synthesis of endocannabinoids is thought to produce anticonvulsant effects, ultimately terminate seizures, and regulate seizure duration and frequency in the pilocarpine model of epilepsy. There is also evidence that the endocannabinoids, such as anandamide and 2-AG, dampen epileptiform activity in hippocampal brain slice preparations (204). Cannabinoids and endocannabinoids have also been demonstrated to block seizure spread via a CB1 receptor-dependent mechanism in the MES model of short-term seizure and the rat pilocarpine model of temporal lobe epilepsy (189,197,199). This plethora of evidence indicates that an active endogenous cannabinoid tone maintains chronic activation of presynaptic CB1 receptors that play an important role in dampening persistent excitation in epilepsy and may prevent the transition of a single seizure into SE. In the pilocarpine model of acquired epilepsy, inhibiting endogenous cannabinoid tone using a CB1 receptor antagonist caused a marked increase in seizure frequency and duration and produced SE-like activity (189), suggesting that the endocannabinoid system was playing an important role in preventing the development of SE. Thus, the endocannabinoid system appears to be the brain’s endogenous mechanism that guards against persistent epileptic neuroexcitation and prevents the transformation of a single seizure into SE.
Recently, a lot of interest has been generated in the use of medicinal marijuana for the treatment of seizures (205–207). The marijuana plant, cannabis sativa, has been used for centuries for recreational and medicinal purposes. Over 400 chemicals are found in this plant. Of these, two compounds are of particular significance: delta-9-tetrahydrocannabidiol (THC), the main psychotropic chemical, and cannabidiol (CBD), the nonpsychotropic constituent. Self-medicating with cannabis sativa (medical marijuana) by some epileptic patients has anecdotally indicated some beneficial outcomes for the control of seizures, although to date, no reliable conclusions for the use of medical marijuana can be made. Experimental studies and preliminary clinical findings indicate a therapeutic potential for cannabinoid preparations that are high in CBD and low in THC. Such a composition of cannabinoid-based medication is believed to exert a beneficial anticonvulsant action with minimal side effects. Cannabidiol (CBD) lacks CB1 agonistic activity and is nonpsychotropic. It has been found to exert potent anticonvulsant activity in experimental seizure models. Recent media events have also spurred interest in CBD and clinical trials are underway to test the anticonvulsant efficacy of this compound (208). A liquid preparation of cannabidiol (Epidiolex, GW Pharmaceuticals) is currently under clinical trials for treatment of certain pediatric seizure disorders. Results obtained so far are found to be promising. Twelve weeks of CBD treatment produced approximately 50% seizure reduction in patients with Dravet and Lennox-Gastaut syndromes (209). Further research is needed to delineate psychotropic effects, abuse potential, and development of tolerance to cannabinoid-based medications when used for treating seizure disorders.
New Frontiers in Epilepsy Treatment: Inhibition of Epileptogenesis
More than half of the epilepsies are described as symptomatic or acquired. These types of epilepsy are acquired through environmental stress or injuries to the nervous system that result in the permanent alteration of neuronal function, resulting in epilepsy (210). Conditions such as stroke, head trauma, metabolic disease, prolonged seizures, tumors, or other neurologic insults can permanently alter the brain and trigger mechanisms of neuronal plasticity that eventually result in the development of epilepsy (211). Because of the diversity of the causes of epilepsy, epilepsy is not considered a disease but rather a condition. In addition to the acquired causes as described previously, other idiopathic forms of epilepsy may have a genetic basis (212).
The process of epileptogenesis means the development of spontaneous recurrent seizures in a previously normal brain (187). Symptomatic epilepsy, representing a significant number of patients who develop epilepsy, occurs through the process of epileptogenesis. This is an important area to consider for anticonvulsant drug development. Compounds that prevent the development of epileptogenesis may have important clinical ramifications. If specific antiepileptogenic drugs can be administered following a brain injury, symptomatic epilepsy may be prevented. Although these compounds may not be true anticonvulsant drugs, they would be the ultimate AEDs by preventing the development of epilepsy. This is a new and important area for future research.
Molecular genetics has set the stage for major advances in studying neurologic diseases, and initial advances have been made in understanding specific mutations that are associated with epilepsy and other neurologic conditions (213). In addition to specific mutations, permanent alterations in brain function and diminished expression are associated with epileptogenesis. In symptomatic epilepsy, normal brain tissue is permanently altered and develops spontaneous recurrent seizures. These changes entail long-lasting changes in gene expression at both transcriptional and posttranscriptional levels in association with the induction of epileptogenesis.
Understanding the effects of severe environmental stresses on the multiple sites of transcriptional and posttranscriptional regulation of gene expression is likely to provide important insights into how altered neuronal function develops and point to novel strategies that will prevent epileptogenesis. This important area for future research represents a frontier in the development of AEDs.
REFERENCES
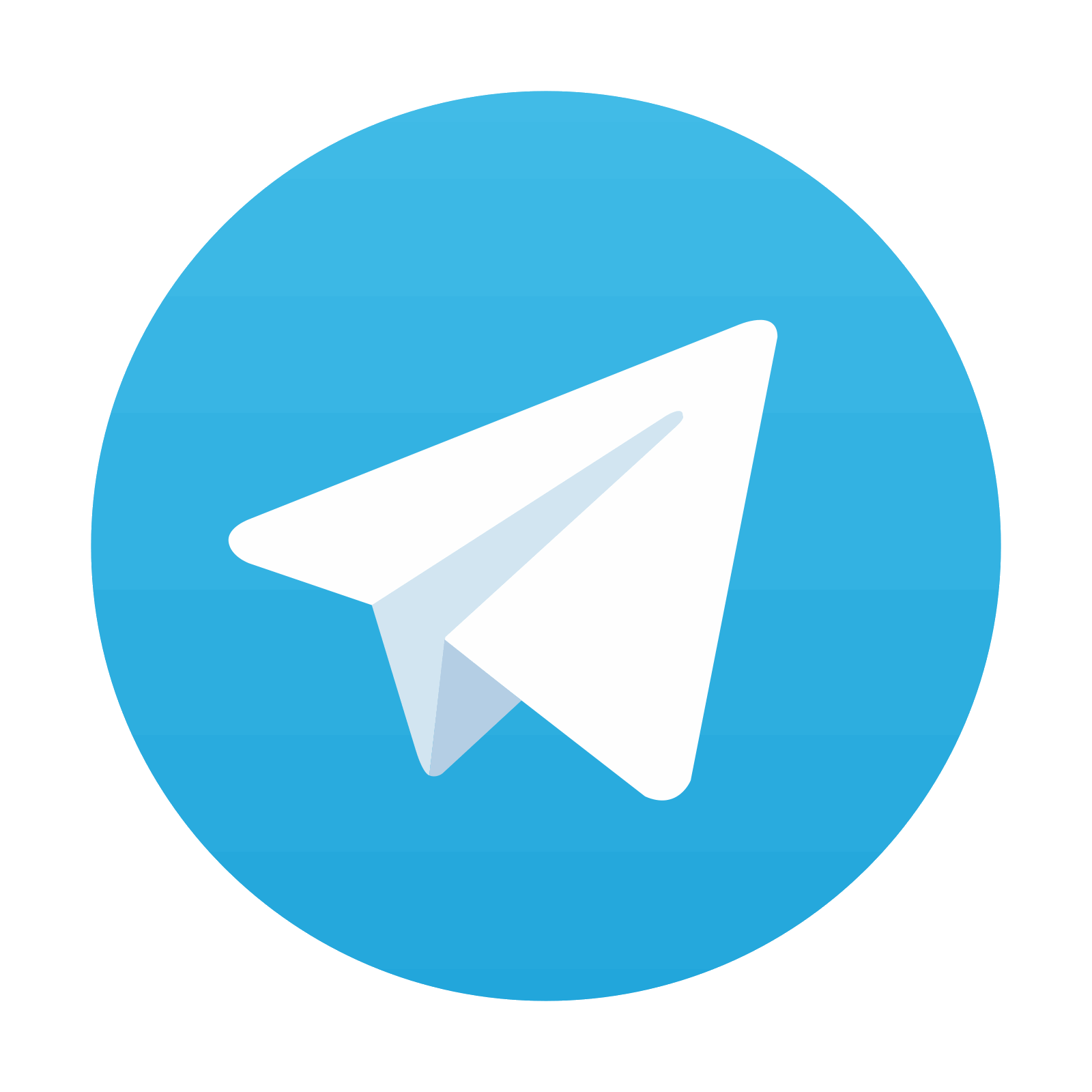
Stay updated, free articles. Join our Telegram channel
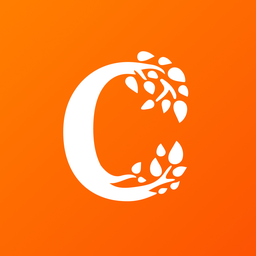
Full access? Get Clinical Tree
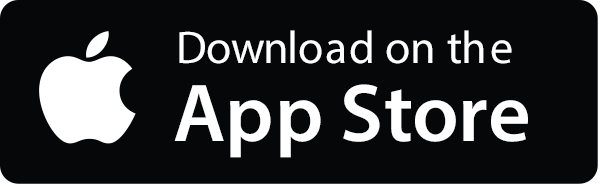
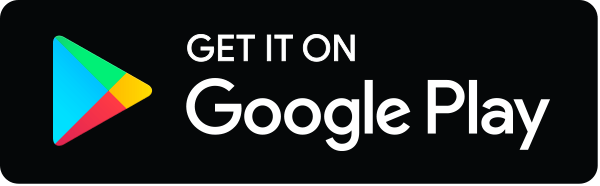