Diagram of a human cell showing major organelles
Nucleus
The nucleus is the largest organelle. It contains the cell’s chromosomes and is the place where almost all DNA replication and RNA synthesis occurs.
The nucleus is separated from the cytoplasm by a double membrane called the nuclear envelope. The nuclear envelope isolates and protects the cell’s DNA from various molecules that could accidentally damage its structure or interfere with its processing. Pores in the nuclear membranes regulate the movement of substances between nucleus and cytoplasm. In many cells the outer nuclear membrane is continuous with the rough endoplasmic reticulum.
The nucleus contains a suborganelle called the nucleolus and it is within this suborganelle that most of the cell’s ribosomal RNA is synthesised.
Endoplasmic Reticulum
The endoplasmic reticulum is a network of interconnected internal membranes. There are two varieties: rough and smooth.
The rough endoplasmic reticulum is so called because it has ribosomes attached to its outer surface; the smooth endoplasmic reticulum does not.
The smooth endoplasmic reticulum is the site where synthesis of fatty acids and phospholipids occurs. Many cells have little smooth endoplasmic reticulum but in liver cells it is abundant. Enzymes in the smooth endoplasmic reticulum of liver cells modify and detoxify molecules such as pesticides and carcinogens and exposure to large amounts of such chemicals results in a large increase in endoplasmic reticulum volume in liver cells.
Ribosomes bound to the rough endoplasmic reticulum synthesise organelle and membrane proteins as well as all proteins to be secreted from the cell. Secretory proteins pass through the rough endoplasmic reticulum membrane and accumulate in the inner cavity of the rough endoplasmic reticulum before being transported to their destination. Rough endoplasmic reticulum is particularly abundant in cells that are specialised in the production of secreted proteins such as antibodies (for example plasma cells) or digestive enzymes (for example pancreatic acinar cells).
Most proteins leave the rough endoplasmic reticulum within minutes after their synthesis in small membrane-bound transport vesicles. These vesicles bud off from the rough endoplasmic reticulum and transport the proteins to the lumen of the Golgi complex.
Golgi Complex
The Golgi complex is also surrounded by a membrane. Within different regions of the Golgi complex, different enzymes modify secreted proteins and membrane proteins in different ways depending on the structure and ultimate destination of the proteins.
After modification, the proteins are transported out of the Golgi complex in a second set of transport vesicles. Some vesicles will contain proteins destined for the plasma membrane, while others contain proteins for lysosomes or other organelles.
Mitochondria
Mitochondria are found in nearly all eukaryotic cells, and there are usually several or many in each cell. These organelles burn glucose for fuel in the process of cellular respiration to produce ATP. Essentially, they are the ‘power house’ of the cell.
Mitochondria have a smooth outer membrane and a convoluted inner membrane, which are separated by an intermembrane space. The convolutions of the inner membrane are called cristae and the space inside the inner membrane is the mitochondrial matrix. As glucose is burned for fuel, a mitochondrion shunts various chemicals back and forth across the inner membrane (from the matrix to the intermembrane space and vice versa).
Mitochondria contain their own DNA, which, in contrast to the nuclear DNA, is inherited through the mother.
Lysosomes and Peroxisomes
Lysosomes and peroxisomes can be likened to the ‘disposal system’ of a cell because they remove substances the cell no longer requires, including old organelles.
Lysosomes and peroxisomes are surrounded by a single membrane and are rich in digestive enzymes. For example, lysosomes can contain more than three dozen enzymes for degrading proteins, nucleic acids and polysaccharides. All lysosome enzymes work at acidic pH values; thus, the internal pH value is maintained at around 4.8. The cell would not be able to house such destructive enzymes if they were not contained in a membrane-bound system to protect the rest of the cell.
Many materials degraded by lysosomes are brought into the cell by one of the following processes:
Peroxisomes contain several oxidases that oxidise organic substances. This process generates the toxic molecule hydrogen peroxide (H2O2), which is then converted to O2 and H2O by the enzyme catalase. The peroxisome is the main organelle for oxidising fatty acids, which are precursors for key synthetic pathways. Toxic molecules in the blood are also cleared by peroxisomes, particularly in liver and kidney cells.
Cytosol and Cytoskeleton
The cytosol of the cell does not only contain the cell organelles, it also contains the cytoskeleton. The cytoskeleton is made up of three classes of fibres: microtubules (20 nm diameter), microfilaments (7 nm diameter) and intermediate filaments (10 nm diameter). The cytoskeleton gives the cell rigidity and strength, thereby allowing it to maintain its shape, and changes in its organisation underpin a cell’s ability to migrate.
Finally, the cytosol also contains many particles such as glycogen-containing granules.
Cell signalling
In multicellular organisms, a complex intercellular communication network exists that coordinates metabolism, differentiation, growth and repair. The term cell signalling refers to the communication between cells by means of signalling molecules. Released by the signalling cell or bound to its surface, signalling molecules produce a response in a target cell that has receptors for that particular signalling molecule. The process of converting an extracellular signal into a response in the target cell is known as signal transduction.
Extracellular signalling by means of secreted molecules can be classified as endocrine, paracrine and autocrine, depending on the distance travelled by the signalling molecule:
Endocrine signalling involves the release of hormones from endocrine glands; the hormones are then carried in the bloodstream to a distant target site (Figure 5.2A).
In paracrine signalling the signalling molecule produced by a cell acts only on cells very close to it (Figure 5.2B); neurotransmitters work by paracrine signalling.
In autocrine signalling a cell responds to a signalling molecule that it has released itself (Figure 5.2C); many growth factors operate like this and it is commonly seen in tumour growth.
In a fourth method of extracellular signalling, the signalling molecules are membrane-bound proteins that can interact with receptors on adjacent cells (Figure 5.2D).
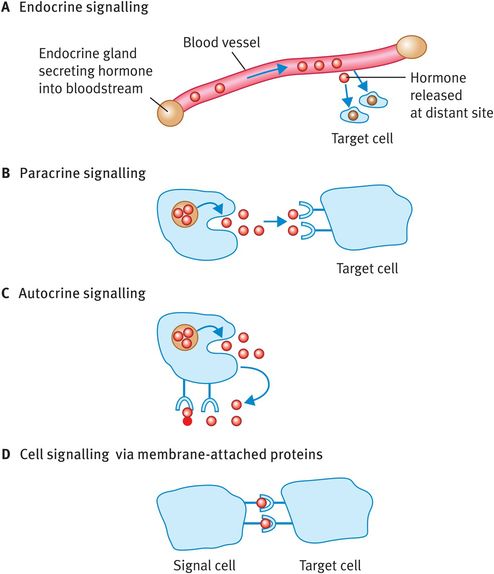
Extracellular signalling methods
Most types of animal cell have a characteristic set of high-affinity receptors allowing them to respond to a range of signalling molecules. The effect of some signalling molecules varies depending on the cell type they encounter. For example, acetylcholine increases the contraction of skeletal muscle but decreases the contraction force of heart muscle and thereby the heart rate. Cells can also modulate their response to a particular ligand by altering the number of cell-surface receptors for that ligand.
Some chemical signalling mechanisms are rapid and transient. Examples include insulin secretion in response to raised blood glucose levels and neurotransmission, a process that is even faster than insulin secretion. Some signalling processes are slow in onset and long-lasting such as estradiol production by the ovaries at the onset of puberty.
Many signalling molecules are required just to allow the cell to survive and further signalling molecules are required to enable the cell to proliferate. When deprived of survival signalling molecules, cells may undergo programmed cell death (apoptosis).
Signalling Molecules
The binding of ligands to receptors leads to increases or decreases in the concentration of second-messenger molecules such as cyclic adenosine monophosphate (cAMP), cyclic guanosine monophosphate (cGMP), diacylglycerol, inositol trisphosphate (IP3) and Ca2+. These in turn modify various cell functions.
Inositol trisphosphate and diacylglycerol
IP3 and diacylglycerol are produced through hydrolysis of inositol phospholipids (phosphoinositides), which are mainly located in the inner half of the plasma membrane. The inositol phospholipid known as phosphatidyl bisphosphate (PIP2) is the most important member of this class of lipids in terms of cell signalling, even though it accounts for less than 10% of the total inositol lipids and less than 1% of all the phospholipids in the cell membrane. The breakdown of PIP2 starts with the binding of a signalling molecule to its receptor in the plasma membrane. The activated receptor stimulates a guanine-nucleotide-binding protein (G protein) known as Gq, which in turn activates an inositide-specific phospholipase C called phospholipase C-β. This enzyme cleaves PIP2 into two products (Figure 5.3):
IP3
diacylglycerol.
These two molecules act as mediators for separate signalling pathways.
IP3 is small and water-soluble. It diffuses into the cytosol, where it binds to and opens IP3-gated Ca2+ release channels in the endoplasmic reticulum (Figure 5.3) or, in muscle cells, ryanodine receptors in the sarcoplasmic reticulum. In fact, in many cell types both forms of Ca2+ receptors are present. To end the Ca2+ response, Ca2+ is pumped back out of the cytosol and IP3 is broken down by phosphatases within the cell.
Some of the IP3 is also phosphorylated to form inositol tetrakisphosphate (IP4), which may promote the refilling of the intracellular Ca2+ stores and/or mediate slower or longer-lived responses within the cell.
Diacylglycerol remains attached to the plasma membrane and has two potential fates. First, it can be cleaved to give arachidonic acid, which can be used as a precursor in the synthesis of eicosanoids. Second, and more importantly, it can act as a second messenger. The initial rise in Ca2+ brought about by IP3 causes an enzyme called protein kinase C (C because it is Ca2+-dependent) to move from the cytosol to the plasma membrane, where it is activated by diacylglycerol (Figure 5.3).
Protein kinase C is a serine/threonine protein kinase that regulates the function of other proteins by phosphorylating their serine and/or threonine residues. In addition, protein kinase C can alter the transcription of specific genes. In one pathway, the enzyme catalyses the phosphorylation of mitogen-activated protein (MAP) kinase, which in turn phosphorylates and activates the transcription factor Elk-1. Together with another protein (the serum response factor), Elk-1 binds to the serum response element, a short DNA sequence in the promoter region of the target gene. This leads to transcription of the gene. In another pathway, activation of protein kinase C results in the release of nuclear factor kappa B, which moves into the nucleus also to activate the transcription of specific genes.
As diacylglycerol is rapidly metabolised, sustained activation of protein kinase C for longer-term responses depends on a second wave of diacylglycerol production. This time, diacylglycerol is released by phospholipase-mediated cleavage of phosphatidylcholine, the major phospholipid in the cell.
Eicosanoids
Eicosanoids are signalling molecules that are continuously made in the plasma membrane of all mammalian tissues. They are synthesised from 20-carbon fatty acid chains (mainly arachidonic acid), which in turn are cleaved from membrane phospholipids by phospholipases. There are four major groups of eicosanoids:
prostaglandins
prostacyclins
thromboxanes
leucotrienes.
The first three are collectively known as prostanoids. The generation of prostanoids is catalysed by the enzyme prostaglandin H2 synthase, also known as cyclooxygenase (COX), whereas formation of leucotrienes occurs via a lipoxygenase pathway.
Detailed information on the prostaglandin synthetic pathway is provided in Chapter 6. In the current context, it is important to note that there are two main forms of COX, known as COX-1 and COX-2. These two enzymes have similar functions but are the products of different genes. In general, COX-1 is found in tissues that produce prostaglandins constantly, such as the stomach mucosa, whereas COX-2 is only expressed at sites of inflammation and so is inducible. A third type of COX, COX-3, is formed by alternative splicing of the gene encoding COX-1.
Prostaglandins and leucotrienes are important stimulators of the myometrium during labour (see Chapter 6 for a discussion of the regulation of myometrial contractility). The increase in prostaglandin levels during labour is thought to be caused by induction of COX-2 in fetal membranes. Bacterial products and proinflammatory cytokines can also increase expression of COX-2 and hence prostaglandin synthesis. In addition, high concentrations of prostanoids have been reported during menstruation, in particular in conditions associated with painful menstruation, such as menorrhagia, dysmenorrhoea and endometriosis.
The synthetic pathways of eicosanoids can be targeted by therapeutic drugs. For example, corticosteroid drugs such as cortisone inhibit phospholipase and are used to treat inflammatory conditions such as arthritis. Non-steroidal anti-inflammatory drugs (NSAIDs), including aspirin and ibuprofen, block the first oxidation step of arachidonic acid that is catalysed by COX.
Older NSAIDs such as indometacin inhibit both COX-1 and COX-2. Newer NSAIDs are selective for COX-2. In general, the more COX-2-selective an NSAID is, the better its adverse effect profile. For example, COX-2-selective inhibitors are just as effective in the treatment of menstrual pain as NSAIDs but have fewer gastrointestinal adverse effects.
Aspirin inhibits both COX-1 and COX-2 (it is actually much more active against COX-1 than COX-2; hence its poor adverse effect profile). Unlike most NSAIDs, which are competitive antagonists, aspirin functions by permanently acetylating the active site of the COX enzyme. It can be used at a low dose to inhibit platelet thromboxane synthesis with little effect upon vascular endothelial prostacyclin synthesis. This may be of value in thromboprophylaxis and in the management of pre-eclampsia.
Low doses of aspirin permanently disable platelet COX as the platelets pass through the hepatic portal system. Since the platelet has no nucleus, it cannot synthesise a new supply of COX and so platelet thromboxane synthesis is permanently inhibited. Most of the aspirin is then inactivated within the liver. The small amount of aspirin that then passes into the general circulation may acetylate vascular endothelial COX but, since these cells have a nucleus, they can synthesise a new supply of COX and maintain prostacyclin synthesis.
Nitric oxide and carbon monoxide
Most signalling molecules are hydrophilic molecules but some are small enough to pass straight into the cell where they can directly exert their effects. One such example is the gas nitric oxide (NO). NO is produced from l-arginine by the enzyme NO synthase in the presence of co-factors and O2; the by-product is l-citrulline. There are three main forms of this enzyme:
endothelial NO synthase (eNOS)
inducible NO synthase (iNOS)
brain NO synthase (bNOS).
These enzyme isoforms are the products of different genes that share about 50–60% sequence homology. Many cells express more than one form of NO synthase.
eNOS was first described in endothelial cells. It is constitutively expressed and is dependent on Ca2+ and calmodulin. NO has a very short half-life (5–10 seconds) and is converted to nitrates and nitrites in the blood. Endothelial cells on blood vessels release NO in response to increased shear stress or agents such as acetylcholine. The released NO diffuses to the underlying smooth muscle, where it reacts with iron in the active site of the enzyme guanylate cyclase to produce the intracellular mediator cGMP. The effects of guanylate cyclase are rapid and result in muscle relaxation. Thus, continual release of NO from blood vessels is one of the main mechanisms for keeping blood pressure at its normal level.
In pregnancy, blood pressure falls and it is thought that the associated vasodilation is partly mediated by increased NO release. NO is also important in the regulation of blood flow within the placenta and eNOS is expressed on the entire syncytiotrophoblast surface, just as it is expressed on blood vessels. Placental eNOS is thought to inhibit the aggregation of neutrophils and platelets present in maternal blood in the intervillous space.
The expression of iNOS is induced in response to inflammatory signals such as bacterial cell-wall products, which include lipopolysaccharides and cytokines such as interferon gamma or tumour necrosis factor alpha. It is induced in activated macrophages and neutrophils. NO released from these cells helps them to kill invading microorganisms. The activity of iNOS is independent of Ca2+ and calmodulin.
bNOS was first described in the brain. Like eNOS, it is constitutively expressed and is dependent on Ca2+ and calmodulin for activity.
NO is released by many types of nerve cell to convey signals to neighbouring cells. For example, NO released by autonomic nerves in the penis causes local blood-vessel dilatation resulting in penile erection. In reproductive biology, NO has also been implicated in the control of myometrial quiescence, in the onset of labour and in cervical ripening, although the evidence for some of these functions is certainly not conclusive.
The effects of NO on blood vessels also explain the efficacy of glyceryl trinitrate in the treatment of angina, for which it has been used for nearly 100 years. Glyceryl trinitrate is converted to NO, which relaxes blood vessels in the heart. Glyceryl trinitrate has also been used in an attempt to prevent preterm labour by relaxing myometrial smooth muscle and to ripen the cervix.
Carbon monoxide (CO) is another gas that stimulates guanylate cyclase. CO is produced by the enzymes haem oxygenase 1 and 2. Haem oxygenase 1 is inducible, while haem oxygenase 2 is constitutively expressed. It is now known that CO, like NO, is important in maintaining vasodilatation in the placenta.
Calcium
Cells maintain low concentrations of free Ca2+ (100 nmol/l) despite much higher concentrations in the extracellular fluid (10 mmol/l) and endoplasmic reticulum. Increases in intracellular Ca2+ concentrations are one way in which extracellular signals are transmitted across the plasma membrane. When Ca2+ channels are transiently opened in the plasma or endoplasmic reticulum membranes, intracellular Ca2+ concentrations rise to about 50 micromoles/l and activate Ca2+-responsive proteins in the cell. Resting Ca2+ concentrations are kept very low by several means. Ca2+-ATPases in the plasma membrane pump Ca2+ out of the cell. Nerve and muscle cells, which use Ca2+ to a greater extent for signalling, have an additional Ca2+ pump (Na+,Ca2+-exchanger) in the plasma membrane, which couples Na+ influx to Ca2+ efflux.
In the endoplasmic reticulum, there is yet another Ca2+-ATPase that takes up Ca2+ from the cytosol. Mitochondria can also pump Ca2+ inside; a low-affinity, high-capacity Ca2+ pump in the inner mitochondrial membrane uses the electrochemical gradient generated across the membrane during electron transport in oxidative phosphorylation. This pump only operates when Ca2+ levels are extremely high, usually as a consequence of cell damage. Ca2+-binding proteins in the cytoplasm and in the endoplasmic reticulum offer additional Ca2+ buffering capacity.
Calmodulin is a Ca2+-binding protein found in all eukaryotic cells. It mediates many Ca2+-regulated pro-cesses and undergoes a conformational change when bound to Ca2+. When this happens, the Ca2+–calmodulin complex can bind to various target proteins and alter their activity. Among the Ca2+–calmodulin targets are various enzymes (such as eNOS and bNOS) and membrane transport proteins. Most effects of Ca2+–calmodulin are, however, indirect and are mediated by Ca2+–calmodulin-dependent protein kinases; an example for such an enzyme is myosin light chain kinase, which activates smooth muscle contraction.
Two pathways of Ca2+ signalling have been well defined. One is used mainly by excitable cells. When nerve-cell membranes are depolarised by an action potential, voltage-gated Ca2+ channels open and Ca2+ enters the cell, leading to the secretion of neurotransmitters. In the other pathway, binding of extracellular signalling molecules to cell-surface receptors ultimately leads to the opening of channels in the endoplasmic reticulum and a rise in the intracellular Ca2+ concentration. The opening of these channels is brought about by the intermediate molecule IP3.
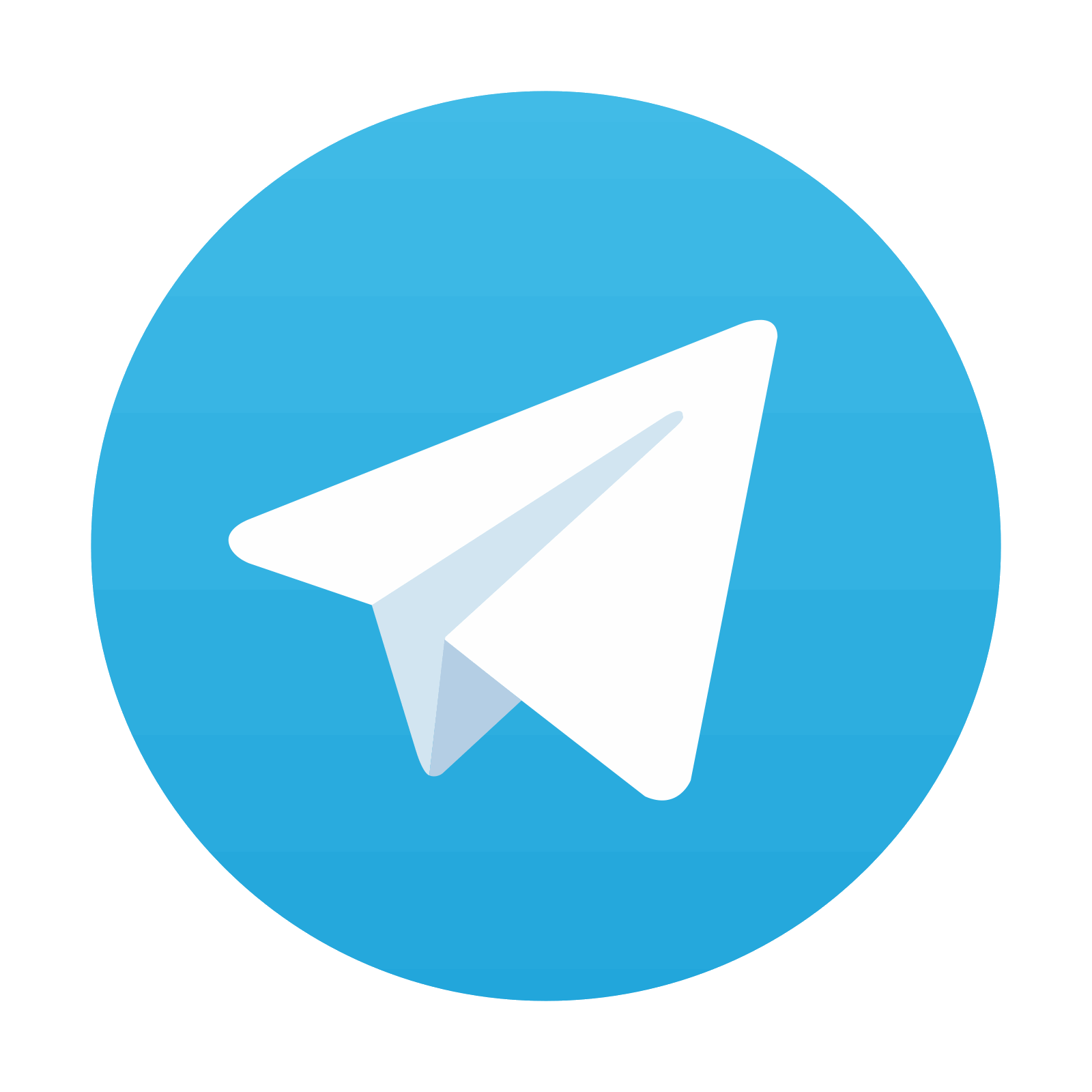
Stay updated, free articles. Join our Telegram channel
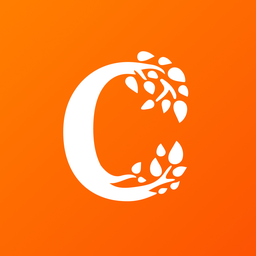
Full access? Get Clinical Tree
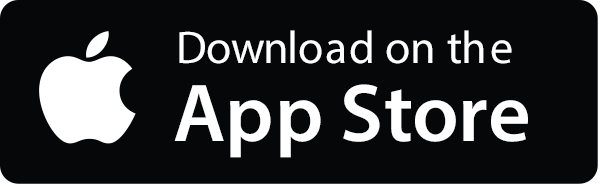
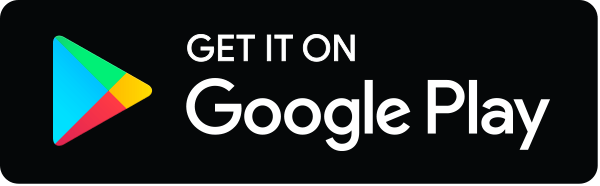