Fetal heart rate modulators
The FHR decreases with advancing gestation, a reflection of the maturing parasympathetic nervous system. The parasympathetic control also influences beat-to-beat variability via its interaction with the sympathetic system (a constant ‘push and pull’) and also changing vagal impulses. As these FHR patterns are controlled by the para-sympathetic and sympathetic nervous systems, the FHR is a reflection of the status of the fetal brain stem’s medulla oblongata. Pathological events, such as fetal hypoxia, that affect these nerve fibre impulses can therefore be observed in FHR patterns. Other influences, such as the fetal sleep and wake states and drugs may also have effects.
Baseline FHR
After the early first trimester, the normal baseline FHR decreases with gestational age. At term, the normal FHR is 110–150 beats/minute.
Fetal tachycardia (at least 160 beats per minute for 10 minutes) can be normal at earlier gestation. Depending on the cause, it is usually mediated via catecholamine release, sympathetic nervous system stimulation or lack of parasympathetic stimulation. Common causes are fetal hypoxia (where it is accompanied by an evolving picture of loss of beat-to-beat variability and late decelerations), maternal pyrexia, chorioamnionitis, tocolysis using beta-sympathomimetic drugs or fetal heart rhythm abnormalities.
Bradycardia is defined as a heart rate of less than 110 beats/minute lasting 10 minutes or longer. Occasionally this can be the normal heart rate of the fetus, when it is an isolated abnormality with normal beat-to-beat variability and accelerations. If the bradycardia occurs with previously normal heart rate, it is usually the response to fetal hypoxia, in particular if the heart rate is below 100 beats/minute. Possible causes include cord compression, placental abruption, maternal hypotension or uterine hyperstimulation. Fetal heart block is a rare cause of persistent fetal bradycardia.
Beat-to-beat variability
The interval between successive fetal heart beats varies in normal circumstances. This beat-to-beat variability is defined as fluctuation in the FHR of at least two cycles per minute and it increases with gestational age. Beat-to-beat variability is divided into long-term variability (LTV) and short-term variability (STV). In clinical interpretation these two are usually reported together, but computerised cardiotocography (CTG) and fetal electrocardiogram (ECG) can measure LTV and STV separately. The LTV measures the oscillations or fluctuations of the heart rate within its baseline range (excluding accelerations or decelerations) and it is measured in cycles/minute. STV is measured in milliseconds and measures the R–R interval between two consecutive QRS complexes on the fetal ECG. Although modern external ultrasound devices use standard CTG to give a close approximation, STV can only be correctly measured using a scalp electrode. The STV therefore reflects the change in the FHR from one beat to the next and is the cause of the rapidly changing display in FHR when using fetal scalp electrode monitoring.
In the absence of any input from the autonomic nervous system, the fetal heart will beat at 110–150 beats/minute without variability. It is the constant modulation of this basal heart rate by the autonomic nervous system that produces the characteristic pattern of beat-to-beat variability. This is usually ascribed to the opposing actions of the sympathetic nervous system causing an increase and the parasympathetic causing a decrease in the FHR, but it may also be caused by repeated, short-lived parasympathetic impulses. Absent or reduced variability may simply be due to preterm gestation, but other causes include the fetal sleep cycle, fetal metabolic acidosis, drugs (central nervous system depressants such as magnesium sulphate, morphine or alcohol) or pre-existing neurologic abnormality.
Fetal heart accelerations
Fetal heart accelerations are defined as an increase of 15 beats/minute for at least 15 seconds. Accelerations are rarely seen in the presence of fetal hypoxia. Conversely, the absence of accelerations for more than 80 minutes correlates with increased neonatal morbidity. Accelerations are usually caused by fetal movements or stimulation (for example, vibroacoustic). Accelerations are thought to occur from direct sympathetic stimulation; repeated accelerations during uterine contractions may be due to mild umbilical vein compression leading to systemic hypotension, which triggers acceleration of FHR.
Fetal heart decelerations
Fetal heart decelerations are defined as a decrease of 15 beats/minute for at least 15 seconds. Decelerations are subclassified into early, variable and late decelerations. Early decelerations occur during uterine contractions. Fetal head compression leads to a transient reduction in fetal cerebral blood flow, hypoxia, hypercapna and hypertension. Triggering of baroreceptors causes parasympathetic stimulation, resulting in a reduction in heart rate.
Variable decelerations occur without a regular pattern in terms of relation with uterine contractions, duration and depth. They are thought to be caused by umbilical cord compression or stretching. The differential compression of umbilical vein and artery may be responsible for the variable appearance of the decelerations. Umbilical vein compression causes a transient reduction of venous return to the heart and reduction in blood pressure. Carotid body baroreceptors are triggered and stimulation of the sympathetic nervous system causes fetal tachycardia. Umbilical artery compression causes an increase in systemic vascular resistance and a rise in blood pressure, and baroreceptor-mediated parasympathetic stimulation causes fetal bradycardia. The frequently observed picture of an FHR deceleration with acceleration before and after its occurrence can be explained by the gradual compression of umbilical vein and then umbilical vein and artery, followed by a gradual release in reverse order. As the depth of variable decelerations is a reflection of baroreceptor stimulation rather than hypoxaemia, fetal wellbeing should be assessed based on the baseline rate, variability and presence of accelerations.
Late decelerations usually begin before the peak of the contraction and are defined as decelerations where the nadir of the deceleration is after the peak of the contraction. They usually have a slow recovery phase. During uterine contractions, the blood supply in the placental intervillous spaces temporarily stops. This means that gas exchange also ceases temporarily and, if there is reduced fetal reserve or uterine overactivity, this leads to a reduction in oxygen absorption (leading to hypoxaemia) and accumulation of lactic acid and carbon dioxide (and therefore acidosis). Hypoxaemia causes chemoreceptor stimulation and results in parasympathetic slowing of FHR. In addition, fetal myocardial hypoxia leads to inability of the myocardium to respond and to further bradycardia. This inability of the fetal heart to respond to autonomic nervous system stimulation often leads to a concomitant reduction in variability.
Transitional Events at Birth
After birth, there is loss of the placental circulation and shunts between the pulmonary and systemic circulations. The cardiac output can then be measured as in the adult. There is a rapid change in the function of the myocardium, with an increase in myocardial contractility. During the first weeks, there is a preferential increase in left rather than right ventricular mass. In the myocardium, there is a switch from lactate and carbohydrate metabolism to using free fatty acids as the preferred fuel.
Fetal circulation
An important prerequisite for the understanding of fetal cardiovascular function is the fetal circulation and the differences with postnatal function. Unlike the adult heart, the four chambers of the fetal heart are arranged as a parallel system where the output of the right and left ventricles mix. This is due to shunts that normally close at birth. There are three such shunts, which are illustrated in Figures 32.2 and 32.3:
ductus venosus: directs blood to the inferior vena cava
foramen ovale: allows blood to pass from the right to the left atrium
ductus arteriosus: connects the pulmonary artery to the aorta. It carries the output of the right ventricle owing to the higher pressure of the pulmonary compared with the systemic circulation.
Before birth, the pulmonary circuit is at high resistance, mainly becasue of compression of the pulmonary capillaries by the collapsed lung, the smooth muscle layer of the pulmonary arteries and the vasoconstrictive effects of low fetal partial pressure of oxygen (PO2). At the same time, the systemic circulation is at low resistance to blood flow, owing to the large placental bed. The presence of shunts, high pulmonary resistance and low systemic resistance allows blood to be diverted from the lungs to the placenta.
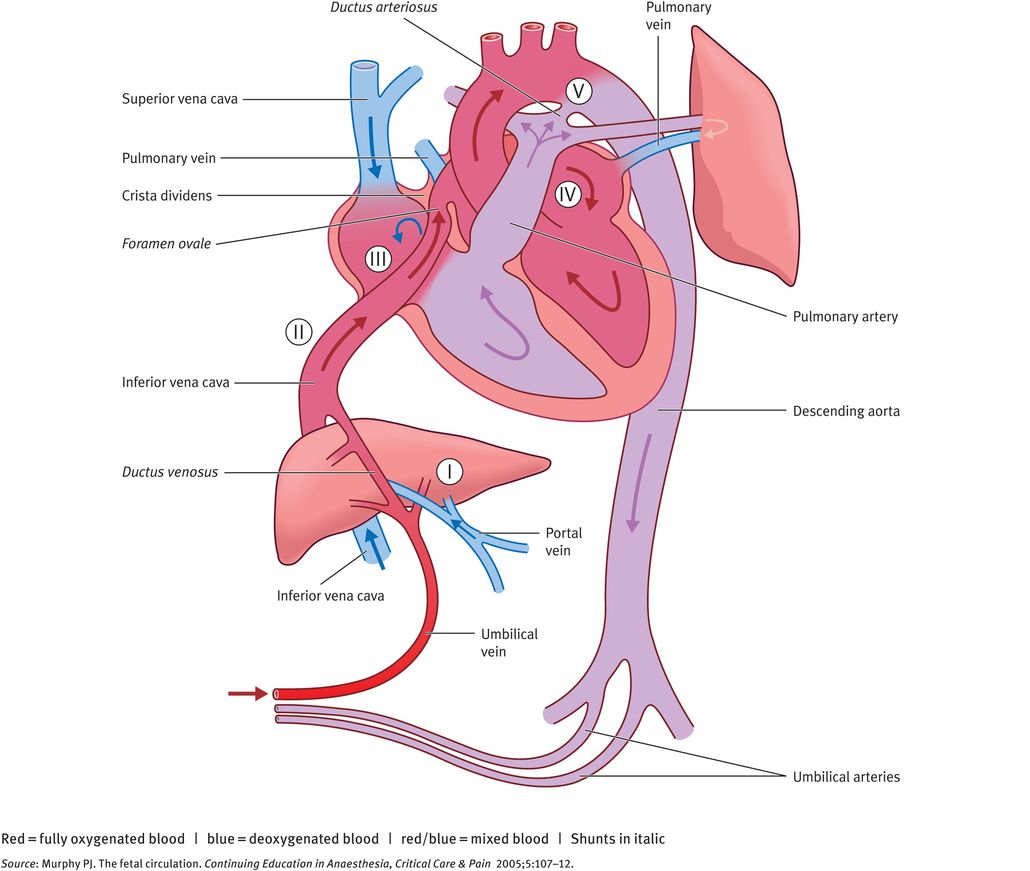
Main characteristics of the fetal circulation showing the fetal shunts
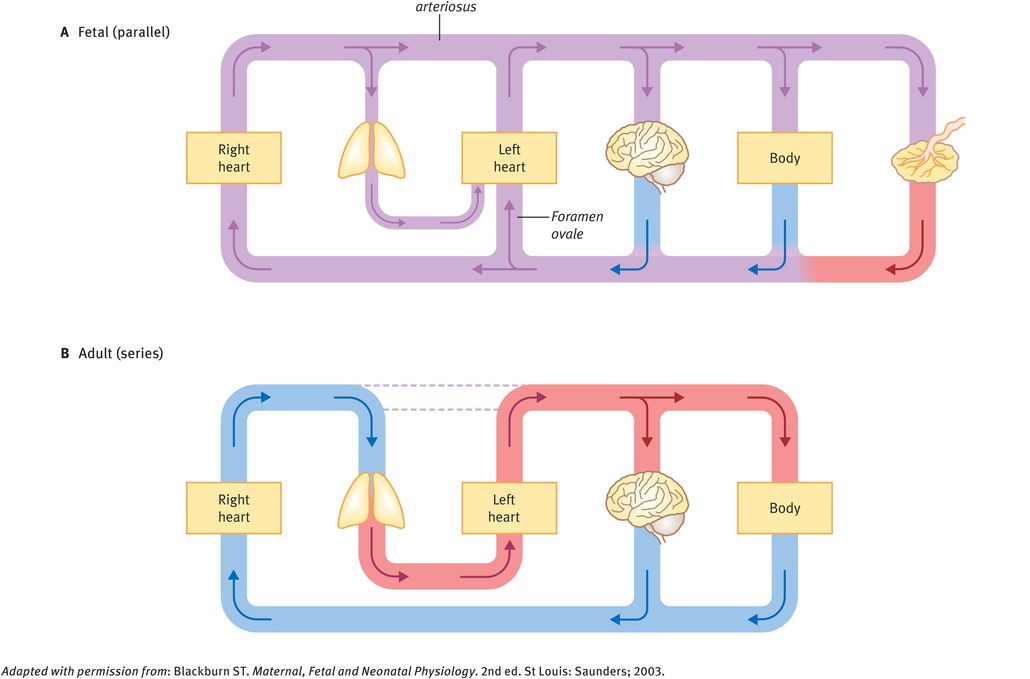
Comparison of fetal and adult circulatory systems
An important feature of the fetal circulation is that, although there is mixing of oxygenated and deoxygenated blood, this does not result in homogenous semioxygenated blood. Rather, preferential streaming of oxygenated blood occurs from the umbilical vein via the ductus venosus and foramen ovale into the left ventricle and proximal aorta, allowing highly oxygenated blood to reach the coronary and carotid arteries. Deoxygenated blood enters the right atrium from the inferior and superior vena cava, through the tricuspid valve into the right ventricle, pulmonary trunk and ductus arteriosus, entering the descending aorta and the umbilical arteries. The physiology of the three fetal shunts allows this preferential streaming of blood.
Ductus Venosus
The ductus venosus connects the umbilical vein to the inferior vena cava (IVC) at the inlet to the heart. The ductus venosus has a small diameter (0.5 mm at mid-gestation to about 2 mm at later gestation) and this narrowing causes an acceleration in blood velocity. This high-velocity blood flow allows preferential streaming of the oxygenated blood coming from the ductus venosus and via the IVC by exerting pressure on the flap-valve of the foramen ovale. About 30% of the umbilical blood is shunted through the ductus venosus at midgestation and this drops to about 20% at 30–40 weeks. This is likely to be because of the development of the fetal liver at later gestations requiring a larger proportion of the umbilical venous blood.
Foramen Ovale
The foramen ovale is a communication between right and left atria and is formed by the overlap of the septum secundum over the septum primum, producing a flap-valve. The higher pressure in the right atrium ensures that the valve is maintained open and allows a right-to-left flow of blood. Blood enters the right atrium from the IVC. Within the IVC, blood flow is not uniform, with the highly oxygenated blood that originated from the umbilical vein flowing anteriorly and to the left within the IVC. As blood enters the atrium from the IVC, it is divided into two streams by the free edge of the atrial septum (the crista dividens). The high-velocity oxygenated blood is shunted towards the left, through the foramen ovale and into the left atrium. The lower velocity, less oxygenated blood is shunted towards the right, mixing with blood from the superior vena cava and coronary sinus.
The net result of this is that blood in the left ventricle is more highly oxygenated than in the right ventricle. The highly oxygenated blood in the left ventricle is pumped into the ascending aorta and 90% of it flows into the coronary arteries, left carotid and subclavian arteries; the remaining 10% flows via the aortic arch and into the descending aorta, mixing with blood from the ductus arteriosus.
Ductus Arteriosus
The ductus arteriosus connects the pulmonary trunk to the descending aorta. This allows blood to bypass the immature lungs. After right ventricular contraction, blood flows mainly through this vessel and into the descending aorta, with about 13% of the combined cardiac output entering the pulmonary circulation, to support lung development. After 30 weeks, the proportion of blood flow to the lungs increases to about 20% of the combined cardiac output. The patency of the ductus arteriosus is maintained by the vasodilator effects of prostaglandins (PGE1 and PGE2) and prostacyclin (PGI2) and reduced fetal oxygen tension.
Fetoplacental Circulation
Blood flow from the fetus to the placenta is via the umbilical arteries and this represents about 33% of the combined ventricular output at 20 weeks of gestation and about 20% after 32 weeks. With increasing fetal growth, the absolute volume of umbilical blood flow increases throughout gestation, but it is lowest at 41 weeks if normalised for fetal weight. The resistance to blood flow through the placental vascular bed has no neural regulation and catecholamines have little effect.
Control of Fetal Circulation
The control of the fetal circulation is complex and poorly understood. The peripheral circulation of the fetus is under a tonic adrenergic influence (predominantly vasoconstriction), probably mediated by circulating catecholamines and in particular by norepinephrine. Other factors such as arginine vasopressin, the renin–angiotensin system and prostaglandins also have a role. Baroreceptors in the aortic arch and carotid arteries are sensitive to changes in arterial blood pressure. Chemo-receptors that are sensitive to changes in the oxygen content ensure that, during hypoxaemia, the blood flow increases to the brain, myocardium and adrenals and decreases to the lungs and lower body. This phenomenon of arterial redistribution is used in clinical practice in the monitoring of fetal growth restriction.
Meeting Fetal Oxygen Needs
The mixing of oxygenated and deoxygenated blood in the fetal circulation means that oxygen content is lower in the fetus than in postnatal life. The mechanisms to ensure adequate oxygen delivery are:
preferential delivery of oxygen-rich blood to the myocardium and brain by presence of shunts
preferential shunting of oxygen-poor blood to the placenta for oxygenation
high heart rate
fetal haemoglobin, which results in oxygen binding even at a low PO2, which results in high saturation, even at low oxygen tensions.
Transitional Events at Birth
Shortly after birth, the low-resistance placental circulation is lost. This loss of blood flow through the placenta means that the systemic resistance is approximately doubled and pressures in the aorta, left ventricle and left atrium increase. At the same time, with the first breath of air there is lung expansion, vasodilation in the pulmonary vascular bed due to higher oxygen tension and a fall in the pulmonary vascular resistance.
The changes in the relative pressures between pulmonary and systemic systems mean that there is a change from the parallel (fetal) system with placental respiration to a neonatal circulatory system in series with pulmonary respiration.
The fall in pulmonary and rise in systemic pressures cause a massive reduction in blood flow through the ductus arteriosus. The ductus arteriosus then closes spontaneously, on average 2 days after birth, most likely because of the increase in oxygen tension. Failure of the closure of the ductus arteriosus can lead to the common problem of ‘patent ductus arteriosus’ in the postnatal period and this is more common in premature infants or those with low oxygen tensions from continuing hypoxia. The ductus arteriosus is sensitive to the influence of prostaglandin E2, which maintains the patency of the vessel. Administration of prostaglandin synthase inhibitors (such as indometacin) can be used postnatally in cases of patent ductus arteriosus for therapeutic purposes, but can cause severe constriction of the ductus arteriosus antenatally if administered during the third trimester.
The ductus venosus usually closes 1–3 weeks after birth in term infants. Unlike closure of the ductus arteriosus, it is thought that in the ductus venosus this is mechanical. Finally, there is functional closure of the flap-like opening of the foramen ovale due to the increase in left atrial pressure. Anatomical closure of the septum primum and septum secundum occurs in the majority of cases by the age of one year. Persistent patent foramen ovale can lead to paradoxical embolic events in later life.
Fetal respiratory physiology
Normal fetal lung development depends on normal anatomical development, fetal breathing movements, absorption of lung fluid at birth and surfactant production.
Anatomical Development
Lung development occurs in five stages, which are described below and in Table 32.1.
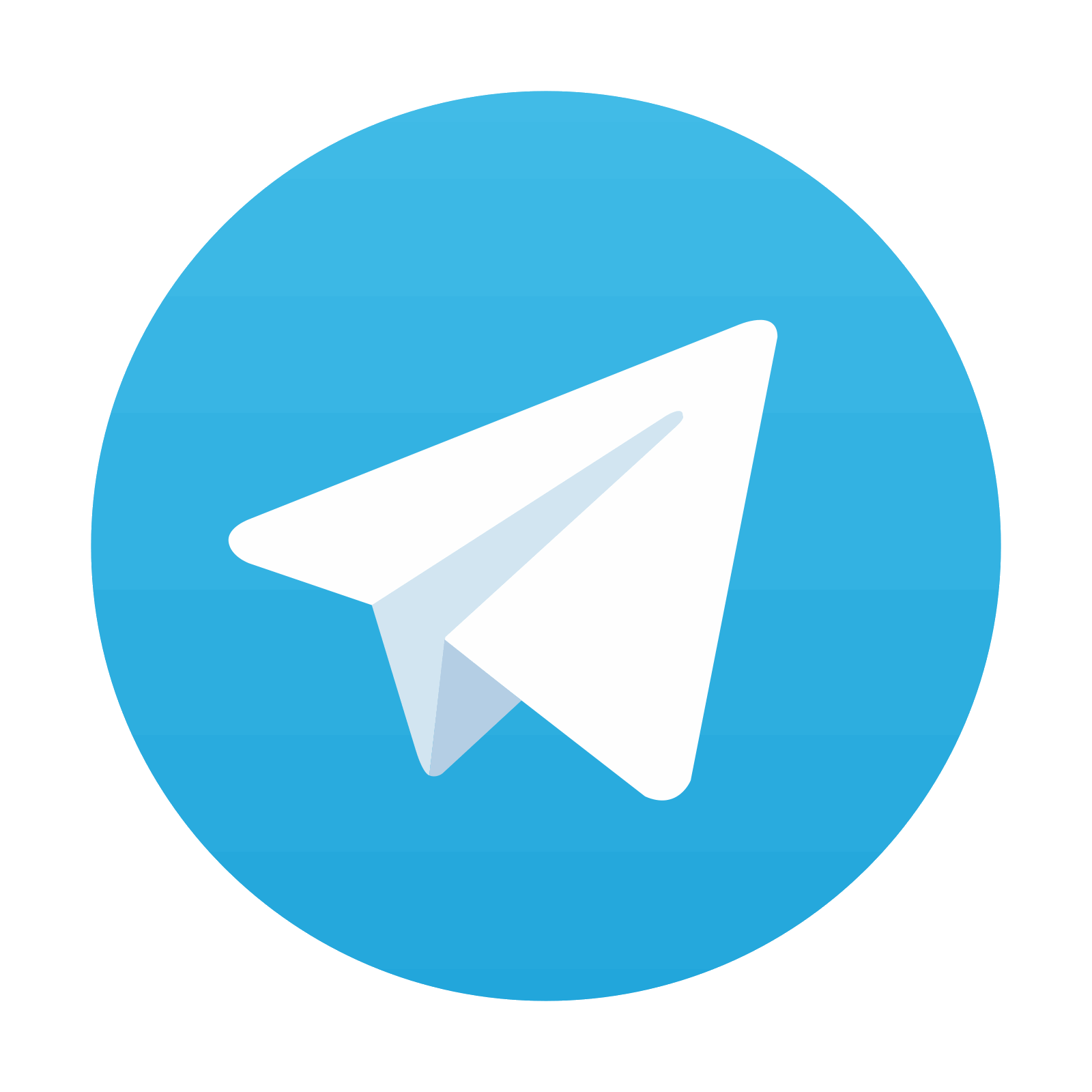
Stay updated, free articles. Join our Telegram channel
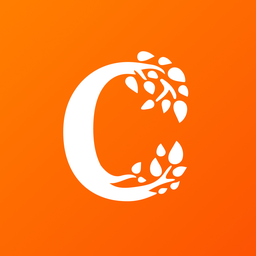
Full access? Get Clinical Tree
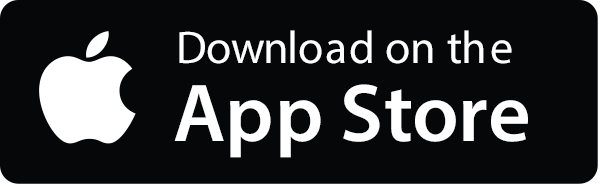
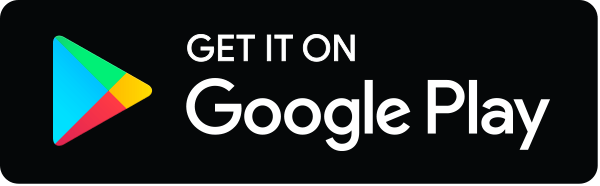