Acknowledgement
The authors acknowledge the contributions of Drs David Durand and Nick Mickas, previous authors of this chapter.
Arterial blood gas measurements are the gold standard by which the adequacy of oxygenation and ventilation is assessed in sick neonates. In modern intensive care, blood gas measurements are used concurrently with continuous noninvasive monitoring devices, particularly pulse oximeters and transcutaneous carbon dioxide monitors, that provide real-time estimates of blood gas values.
The measurement of blood gases is invaluable for management of patients with respiratory difficulty, for such measurements not only enable the diagnosis of respiratory failure by estimation of the magnitude of respiratory illness severity but also provide crucial information required by the clinician to guide adjustments to the mechanical ventilator or other form of respiratory support. In this chapter, we first discuss the physiology of gas transport in the blood, followed by a description of techniques of measurement of blood gases commonly used in the neonatal intensive care unit (NICU). Next, we will describe the technical aspects of blood gas estimation by commercially available devices. Finally, we will discuss the interpretation of blood gases, as well as some perils and pitfalls of blood gas measurement in clinical practice.
Blood Gas Physiology
Gas exchange occurs primarily in lung saccules in extremely preterm infants and in alveoli in more mature preterm and term infants, although some gas exchange occurs through immature skin soon after birth. In the postnatal period after transition, systemic venous blood travels to the right atrium and on to the right ventricle, which pumps the deoxygenated blood to the pulmonary arterial system. This blood travels through the pulmonary vasculature until reaching the capillaries, which are contiguous to alveoli, thereby facilitating gas exchange. Gas exchange occurs in ventilated alveoli, with absorption of oxygen from alveoli into the alveolar capillaries and removal of carbon dioxide from the circulation into the alveoli. In general, the oxygen concentration of arterial blood exiting the left ventricle reflects the matching of ventilation and perfusion. A decrease in oxygen content would occur if perfusion (blood) is not matched with ventilation (aerated alveoli), either because blood passes from the right side of the heart to the left side of the heart without traversing the pulmonary circulation (e.g., extrapulmonary shunt) or because blood is traversing parts of the lung that are atelectatic and/or underventilated (intrapulmonary shunt).
Carbon dioxide (CO 2 ) elimination is primarily dependent upon the magnitude of alveolar ventilation. As alveolar ventilation increases, the partial pressure of carbon dioxide in alveolar gas (P aco 2 ) decreases, and more CO 2 is removed from the blood flowing through the lungs, and the partial pressure of carbon dioxide in blood (Paco 2 ) leaving the left heart decreases. Conversely, a decrease in alveolar ventilation will increase the Paco 2 in systemic arterial blood. An important concept is that while arterial Pao 2 and Paco 2 provide important information about ventilation–perfusion matching and adequacy of alveolar ventilation, they do not provide direct information about the adequacy of oxygen delivery to the systemic vascular bed and peripheral tissues.
Aerobic metabolism of glucose for the production of adenosine triphosphate (ATP) is responsible for the consumption of oxygen and the production of CO 2 . Aerobic metabolism produces approximately 38 ATP molecules for each molecule of glucose consumed, although some of the ATP is consumed in the process, yielding a net of 30 ATP.
During conditions of insufficient oxygen delivery to cells, tissue hypoxia occurs, characterized by anaerobic metabolism of glucose to pyruvate for ATP production. This process is less efficient than aerobic metabolism and yields two molecules of ATP for each molecule of glucose consumed. Pyruvate is metabolized to lactic acid, which increases the base deficit (or decreases base excess) of arterial blood. Base excess is defined as the difference between the actual buffer capacity and the ideal buffer capacity. To more accurately estimate if adequate oxygen has been delivered to tissues, blood returning from the systemic circulation to the heart (mixed venous blood) can be evaluated. Blood sampling from the right atrium, which can be done using an umbilical venous catheter with its tip in the low right atrium, closely approximates mixed venous blood. Monitoring of mixed venous oxygenation is extremely helpful in assessing the adequacy of tissue oxygen delivery and is widely used in adult ICUs and in neonatal extracorporeal membrane oxygenation (ECMO).
Oxygen Transport
Oxygen delivery to tissues depends upon the oxygen content of the blood as well as the cardiac output and its distribution to the tissues. In most neonates, cardiac output ranges from 120 to 150 mL/kg/min, although objective assessment of cardiac output is not easy or accurate, generally depending upon functional echocardiography and Doppler methods or alternative methods such as pulse contour analysis, bioimpedance, or indicator dilution techniques (generally in larger and older infants). The oxygen content of arterial blood (C a O 2 ) consists of oxygen bound to hemoglobin and free dissolved oxygen. Hence,
where C a O 2 is the oxygen content, HbO 2 is the oxygen bound to hemoglobin, and dissolved O 2 is the oxygen in solution.The relationship between the Pao 2 and the hemoglobin saturation is sigmoidal over the physiologic range. Hemoglobin is almost fully saturated at a Pao 2 of 80 to 100 mm Hg ( Fig. 10-1 , curve A ). This sigmoidal oxyhemoglobin dissociation curve (ODC) describes the percent of hemoglobin saturated with oxygen at a given Pao 2 . The concentration of red blood cell diphosphoglycerate (DPG) and the ratio of adult hemoglobin (A) to fetal hemoglobin (F) can shift the position of the dissociation curve. With increasing postnatal age, the concentration of DPG and the proportion of hemoglobin A increase, shifting the curve to the right ( Fig. 10-2 ). DPG has a greater effect on oxygen binding for adult hemoglobin, as compared to fetal hemoglobin. Increases in temperature, Paco 2 , and hydrogen ion concentration (acidosis) also shift the curve to the right. As the curve shifts to the right, hemoglobin can bind less oxygen at a given Pao 2 and therefore releases oxygen more easily to the tissues ( Fig. 10-3 ). The fetus has a lower red blood cell DPG concentration and more hemoglobin F, and the dissociation curve is hence shifted to the left, which helps maintain a higher oxygen saturation at a lower Pao 2 . Conditions such as respiratory alkalosis or therapeutic hypothermia will also shift the dissociation curve to the left, resulting in a higher hemoglobin oxygen saturation for a given Pao 2 .



All gases dissolved in the blood exert pressure proportional to the amount of the gas. The pressure exerted by dissolved oxygen in the blood is represented by the Pao 2 . Normally, only a small amount of oxygen is dissolved in the plasma, unless the Pao 2 is extremely high (e.g., exposure to high FiO 2 or hyperbaric oxygen therapy). At 38° C, 0.3 mL of oxygen is dissolved in 100 mL of plasma (0.003 mL/dL/mm Hg of Pao 2 , at an assumed Pao 2 of 100 mm Hg). This relationship is linear over the entire range of Pao 2 (see Fig. 10-1, curve B ). Because the amount of oxygen that is dissolved in the blood is much less than the amount that is bound to hemoglobin, the oxygen content of blood is approximately equal to the amount of oxygen bound to hemoglobin, as represented by the equation:
The amount of oxygen bound to hemoglobin depends on the hemoglobin concentration, the percentage saturation of the hemoglobin, and the oxygen-carrying capacity of hemoglobin, which can be expressed mathematically as:
O 2 capacity represents the maximum amount of oxygen that can be carried by a gram of hemoglobin that is fully saturated. This value is 1.34 mL of O 2 per gram of 100% saturated hemoglobin.
Assuming a hemoglobin level of 15 g/100 mL blood, and 100% saturation of arterial blood, and ignoring the small amount of dissolved oxygen in blood, the oxygen content of normal arterial blood is approximately:
Using the same assumptions, and with the additional assumption that the normal cardiac output in a newborn is approximately 120 mL/kg/min, the amount of O 2 that can be delivered to the systemic circulation can be calculated as follows:
Oxygen consumption for a neonate is approximately 6 mL/kg/min under normal circumstances. Therefore, the body extracts 6 mL/kg/min of oxygen from the approximately 24 mL/kg/min that is delivered via the systemic circulation. The amount of oxygen delivered is usually much higher than the amount required by tissues, providing a natural reserve. The tissues become hypoxic and switch to anaerobic metabolism only when the oxygen delivery falls below the threshold at which the delivered amount of oxygen is less than the amount required by the tissues. Because only about 25% of the oxygen has been removed from the blood by the time it returns to the heart, the mixed venous blood is approximately 75% saturated. In general, a mixed venous saturation of 70% to 75% represents adequate tissue oxygen delivery. Therefore, mixed venous saturations are usually maintained in the normal physiologic range of 70% to 75% in patients in whom mixed venous saturations can be directly monitored (e.g., patients on ECMO).
Understanding the Oxyhemoglobin Dissociation Curve
One of hemoglobin’s main roles is binding oxygen in the lungs to allow oxygen transport to the body’s tissues. The oxygen is then released from the hemoglobin and enters a dissolved phase in the plasma, which is represented by the partial pressure of oxygen (Pa o 2 ). Without hemoglobin, the cardiac output would have to increase tremendously to meet the body’s metabolic demands as the amount of dissolved oxygen in blood is minimal. The relationship between oxygen saturation and the Pao 2 is described by the sigmoidal ODC. The steep portion of the curve demonstrates that oxygen saturations change quickly during the loading and unloading of hemoglobin with oxygen. This reflects the relaxation of the hemoglobin structure that occurs with oxygen binding. As oxygen binds to heme, the hemoglobin molecule relaxes, thereby exposing further heme molecules, facilitating subsequent binding of oxygen. This process of relaxation is called allosteric modification and is governed by the Haldane effect. The reverse process occurs with oxygen unloading from the hemoglobin molecule. As the oxygen binding sites on the hemoglobin molecule approach full saturation with oxygen, the ODC begins to flatten. Therefore, at higher oxygen saturations it becomes increasingly difficult to predict the Pao 2 from the oxygen saturation. An oxygen saturation of 100% can correspond to a Pao 2 ranging from 80 to over 300 mm Hg.
While the Pao 2 contributes very little to the overall oxygen content of blood, its physiologic importance cannot be overstated as it is the dissolved oxygen in plasma that is available to enter cells. The oxygen that is bound to hemoglobin is not readily available to tissues until it has been released from heme and can dissolve in plasma. Under normal circumstances (e.g., euthermia, normoxemia), 100 mL of blood contains approximately 20 mL of oxygen. The vast majority of this oxygen is bound to hemoglobin with only approximately 0.31 mL of oxygen dissolved in plasma.
The position of the ODC is described by the P50, which represents the Pao 2 at an oxygen saturation of 50%. The position of the ODC, and hence the P50, is dependent on several factors. An increase in body temperature, hydrogen ion concentration (decreased pH), Paco 2 , 2,3-DPG, or adult hemoglobin concentration will each independently shift the ODC to the right, whereas a decrease in any of these factors will shift the curve to the left. Therefore, the Pao 2 for any given oxygen saturation will vary within an individual over time as these factors change. For example, in the presence of a fever, the rightward shift of the curve results in a higher Pao 2 for the P50 value. Both adult hemoglobin concentrations and 2,3-DPG concentrations increase over the first year, causing a shift in the ODC position to the right.
Considerations Regarding Fetal Hemoglobin
As all neonates generally have mostly fetal hemoglobin, it is worth expanding on its importance further. Babies born at <30 weeks’ gestation have nearly 100% fetal hemoglobin. The ratio of fetal to adult hemoglobin gradually diminishes so that by 40 weeks’ gestation, fetal hemoglobin accounts for approximately 70% of all hemoglobin species, with adult hemoglobin accounting for the remaining 30%. This shift to producing adult hemoglobin and away from producing fetal hemoglobin is related to postmenstrual age and not to chronological age. Therefore, premature delivery does not affect the rate of transitioning away from fetal hemoglobin production. A baby born at 24 weeks with nearly 100% fetal hemoglobin would be expected to have approximately 70% fetal hemoglobin at 16 weeks of age (40 weeks’ corrected gestational age), similar to a baby born at 40 weeks’ gestation, although the ratio will change more quickly following transfusion with adult blood.
The first observation that fetal hemoglobin has different oxygen binding properties than adult hemoglobin was reported in 1930. Fetal hemoglobin is composed of two α chains and two γ chains, in contrast to adult hemoglobin, which contains two α and two β chains. This difference results in fetal hemoglobin having a higher oxygen affinity than adult hemoglobin. This promotes the movement of oxygen from the maternal side of the placental circulation to the fetal side because fetal hemoglobin will hold onto oxygen “more tightly” than adult hemoglobin, and this is reflected in the position of the ODC. The P50 observed in a term newborn is approximately 21 mm Hg compared to a P50 of 27 mm Hg observed in adults (see Fig 10-2 ). In preterm babies the P50 may be as low as 18 mm Hg because of the presence of higher fetal hemoglobin and lower 2,3-DPG concentrations.
It is important to consider how therapies can alter the position of the ODC curve. Perhaps most important in this regard is the influence of transfusions of packed red blood cells. Because the packed red blood cells administered to patients are donated by adults, the transfused blood essentially contains 100% adult hemoglobin. The result is a shifting of the ODC to the right after a transfusion. As preterm babies have higher concentrations of fetal hemoglobin, blood transfusions in this population will have an even greater effect in shifting the ODC compared to babies at term. Preterm babies are more likely to receive blood transfusions, which further highlights the importance of this practice in this vulnerable population. There are other important factors to consider regarding the effects of blood transfusions on the ODC, such as the 2,3-DPG concentration, temperature, and type of preservative used in the packed red blood cells. However, a detailed discussion of these factors is beyond the scope of this text.
Is this clinically important? Wimberley and colleagues state that based on the position of the ODC, the oxygen saturation limits for hypoxemia and hyperoxemia can vary between 85% and 94% and between 96% and 98%, respectively. This demonstrates the importance of considering how oxygen saturation targets are related to the Pao 2 as described by the ODC. In practice, clinicians target specific oxygen saturation ranges to help guide oxygen titration and avoid the dangers of both hyperoxemia and hypoxemia. However, oxygen saturation targets are not routinely changed when the position of the ODC shifts, such as following a blood transfusion, which may result in a higher Pao 2 for any given oxygen saturation compared to pretransfusion. Arguably, this is appropriate, because it is the oxyhemoglobin saturation rather than Pao 2 that directly affects oxygen content of blood and tissue delivery of oxygen.
Modern blood gas analyzers have two presets for fetal hemoglobin: 0% and 80%. While not exact, using the preset for 80% fetal hemoglobin is preferable for newborn blood gas testing. For example, consider a blood gas sample corrected to 80% fetal hemoglobin with a P50 of 15 mm Hg and an oxygen saturation of 92% to 97%. The same blood gas sample run on a blood gas analyzer not corrected for fetal hemoglobin would give a P50 of 20 mm Hg, corresponding to an oxygen saturation of 86% to 95%.
On occasion, errors in reporting uncorrected blood gas values are not obvious to the clinician. A blood gas result giving an oxygen saturation of 100% that was corrected for fetal hemoglobin corresponds to an uncorrected oxygen saturation of 105%. However, because blood gas machines do not report oxygen saturations >100%, this would not be apparent to the clinician.
Hypoxemia and Hypoxia
Although hypoxemia and hypoxia often occur together, they are not synonymous. Hypoxemia is generally defined as low arterial blood oxygen content, whereas hypoxia refers to inadequate delivery of oxygen to tissue. Hypoxemia occurs in any situation in which blood enters the systemic circulation without perfusing adequately ventilated alveoli (reduction in ventilation–perfusion matching). Blood can bypass adequately ventilated alveoli by extrapulmonary shunts, by intrapulmonary shunts, or by some combination of the two. With cyanotic congenital heart disease, a structurally abnormal heart leads to some blood entering the aorta without passing through the pulmonary circulation (extrapulmonary shunt). Similarly, infants with persistent pulmonary hypertension of the newborn can also have extrapulmonary shunting through the foramen ovale and/or ductus arteriosus. A right-to-left shunt across the ductus arteriosus can often be detected by comparing the Pao 2 or oxygen saturation of preductal and postductal blood ( Fig. 10-4 ). If the saturation of the preductal blood is significantly higher (≥5% to 10% difference) than the saturation of the postductal blood, a clinically significant right-to-left shunt exists. However, equal pre- and postductal saturations do not exclude the possibility of pulmonary hypertension with a shunt through the foramen ovale. Hypoxemia associated with lung diseases that are characterized by atelectasis (e.g., respiratory distress syndrome, pneumonia) are caused primarily by intrapulmonary shunting. Whenever alveoli are inadequately ventilated, the blood flowing to those alveoli may not become fully saturated. Thus, the greater the degree of atelectasis, the greater the intrapulmonary shunt, and the greater the degree of hypoxemia.

Tissue hypoxia results when the amount of oxygen delivered to the tissues decreases below the critical threshold of oxygen consumption. Hypoxia can occur despite adequate Pao 2 . It is helpful to conceptualize hypoxia in terms of imbalances in supply, delivery and demand for oxygen. Decreased ‘supply’ of oxygen is observed at high altitude due to a lower partial pressure of oxygen in the atmosphere. ‘Delivery’ of oxygen is impaired in the settings of inadequate tissue perfusion (e.g., reduced cardiac output), anemia and abnormal hemoglobin species (e.g., methemoglobin) where the unloading of oxygen to the tissues is negatively affected. The ‘demand’ for oxygen will increase as tissue oxygen requirements rise in response to illness such as fever or sepsis.
Carbon Dioxide Transport
Carbon dioxide transport is significantly less complicated than oxygen transport. Carbon dioxide is produced in tissues during the aerobic metabolism of glucose and is transported in the blood to the lungs, where it is exhaled. Eighty-five percent of the carbon dioxide in blood is transported as carbonic acid, 10% is carried by hemoglobin as carbamate, and 5% is transported as either dissolved gas or carbonic acid. Owing to the equilibrium between dissolved carbon dioxide and the bicarbonate ion, the relationship between the partial pressure of carbon dioxide in the blood (Pa co 2 ) and the total CO 2 content of blood is essentially linear over the physiologic range ( Fig. 10-5 ).
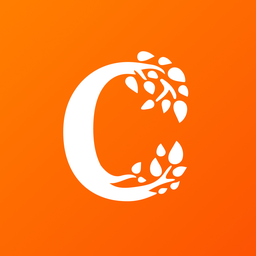
Full access? Get Clinical Tree
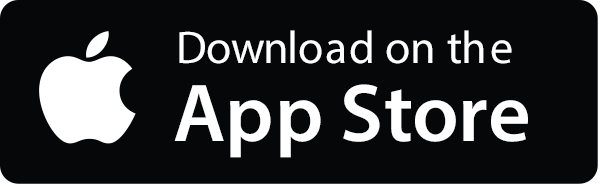
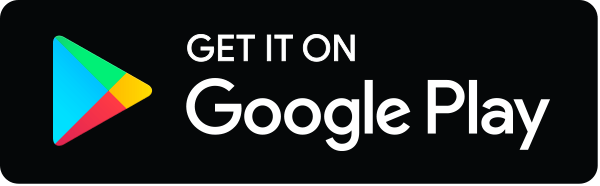