Basic Life Support
Constance M. McAneney
Introduction
Basic life support (BLS) encompasses early recognition and intervention for respiratory and cardiopulmonary arrest. The goal of BLS is to artificially supply oxygen to the body, most importantly to the brain and heart, through the use of rescue breathing and chest compressions. BLS can represent either a definitive intervention (since spontaneous respirations and normal cardiac activity will sometimes resume) or a temporizing measure until advanced life support (ALS) can be administered (the usual case). BLS is a crucial component of the overall emergency medical system for children, which includes bystander cardiopulmonary resuscitation (CPR), rapid response by appropriately trained prehospital personnel, and transport to a hospital emergency department (ED). Prompt initiation of BLS is critical for a good outcome. Consequently, all individuals responsible for the care of children—including parents and other caregivers, emergency medical personnel, nurses, and physicians—should have training in this procedure.
Cardiopulmonary arrest in the pediatric population is an uncommon event. In fact, only 10% to 15% of all arrest victims are younger than 19 years of age (1). The age range most commonly affected is children under 1 year, representing approximately half of all pediatric arrests. Among the remaining patients, children between the ages of 1 and 4 years account for 15% to 30% of pediatric arrests, school-age children (5 to 12 years) 15% to 20%, and teenagers 10% to 15% (1,2,3,4,5). With children younger than 4 years of age, the causative event is most likely to occur in or around the home, including apparent life-threatening events (formerly called “near-miss sudden infant death syndrome”), household injuries, respiratory illnesses, drownings, and neurologic disorders. For school-age children, the streets and recreational areas are more common sites, with injuries and drownings representing the most common causes (2,6,7,8).
Asystole is by far the most common arrest rhythm seen among pediatric patients, accounting for 75% to 90% of documented cases (1,5,7,9,10). However, ventricular fibrillation (VF) also occurs. Two studies suggest that from 6% to 11% of children and young adults who experience a pulseless arrest have VF (11,12). Pediatric patients presenting with VF are likely to have a specific insult to the heart, such as accidental ingestion of a cardiotonic medication, metabolic abnormalities, or underlying cardiac disease (2,7,9,10). Unlike in the case of adults, coronary artery insufficiency is a rare cause of pediatric arrest. As a result, whereas adult patients usually suffer a sudden primary cardiac arrest, cardiopulmonary arrest in children generally represents a “final common pathway” after progressively worsening compromise as a result of other illnesses. Indeed, respiratory etiologies of arrest are far more likely among pediatric patients than are cardiac etiologies.
Most studies of out-of-hospital normothermic cardiac arrest in children report survival averages of 10%, although most of these victims suffer permanent neurologic impairment (4,5,8,13,14). Not surprisingly, the highest rate of intact survival is achieved with witnessed arrests when there has been prompt institution of BLS and ALS procedures (2,3,6,9). In a study of out-of-hospital arrest in children, Hickey et al. found an overall 27% survival rate; all but one of the survivors had return of spontaneous circulation prior to arrival in the ED (15). Conversely, the intact survival rate for respiratory arrest alone may exceed 50% with rapid intervention (4,10,13). This relatively high rate of survival underscores the importance of early recognition and resuscitation for pediatric patients who suffer a primary respiratory arrest.
This chapter focuses exclusively on the CPR component of BLS for children. Although traditionally included with BLS procedures, the initial management of upper airway foreign bodies (back blows, Heimlich maneuver, etc.) is not discussed in this chapter. A complete description of both the basic and advanced techniques for managing upper airway foreign bodies is found in Chapter 51.
Anatomy and Physiology
Airway/Breathing
Various techniques for attempted resuscitation of the dead or near-dead have been described since ancient times (16). Proposed methods for reviving victims have included rolling on a barrel, bouncing on a galloping horse, and using a bellows to blow air into the lungs. Manual methods of ventilation using either back pressure arm-lift or chest pressure arm-lift date back almost 150 years (17,18). The Schafer “prone pressure method” was taught by the American Red Cross until the late 1950s, when mouth-to-mouth resuscitation was recognized as being more effective.
References to mouth-to-mouth ventilation date as far back as biblical times. In 1771, Tossach described inflating a person’s lungs using the rescuer’s expired air (19). Yet it was the pivotal studies by Safar and Gordon published in the 1950s that demonstrated that mouth-to-mouth ventilation was superior to other methods for all age groups in providing rescue breathing (20,21,22). These reports, along with the landmark publication by Kouwenhoven et al. describing closed chest compression (23), began the modern era of CPR.
A number of important differences exist between pediatric patients and adults that are relevant to airway control and rescue breathing (24,25) (Fig. 12.1). For one, the occiput of an infant is more prominent, which may cause a flexed airway when the infant is lying in the supine position. In addition, when compared with adults, infants and younger children have a larger tongue relative to the size of the hypopharynx and greater laxity of the pharyngeal soft tissues. These characteristics contribute to the fact that pediatric patients are prone to develop airway obstruction simply from lying supine. Moreover, the neck is short and chubby, making appropriate positioning more difficult. The tracheal cartilage is soft and easily compressible; as a result, hyperextension of the infant’s head and neck can produce airway obstruction. There is also greater airway resistance caused by the narrower luminal diameter of the pediatric airway. According to Poiseuille’s law, resistance to gas flow is inversely proportional to the fourth power of the radius (25). A pediatric airway one-half the diameter of an adult airway will therefore have a 16-fold greater resistance to flow. For this reason, even small changes in airway diameter (e.g., due to inflammation, edema, or improper positioning) may cause severe respiratory compromise in a younger patient. Further discussion of this concept and other aspects of pediatric airway anatomy can be found in Chapters 14 and 16.
Many aspects of the mechanics of breathing are also unique to infants and children. Because the pediatric rib cage is very pliable and more horizontally oriented, it does not provide the same degree of support as with an adult, and therefore the residual capacity of the lungs is decreased. If there is diminished air entry resulting from intrinsic lung disease or upper airway obstruction, the negative pressure created during inspiration causes paradoxical movement of the chest. In addition, infants and younger children are more dependent on diaphragmatic excursion for ventilation. Any conditions that hamper movement of the diaphragm (abdominal distension, masses, etc.) can therefore significantly impair gas exchange. Children also develop hypoxemia in response to respiratory insults more rapidly than adults due in part to differences in the work of breathing and alveolar surface area. Efficient respiratory effort takes place when a minimum of energy is expended to provide an adequate respiratory rate and tidal volume. Increased airway resistance and greater chest wall compliance cause children to achieve efficient breathing at higher relative rates than adults, disproportionately increasing the metabolic demands of respiration (25). Furthermore, the smaller alveolar surface area of a pediatric patient results in a diminished reserve for gas exchange. As a result, processes that cause mismatching of ventilation and perfusion in the lungs are more likely to produce hypoxemia in children.
As mentioned previously, respiratory failure is the primary cause of cardiopulmonary arrest among pediatric patients. Respiratory failure results from inadequate oxygenation of blood and/or inadequate ventilation. Inadequate oxygenation, which is usually caused by either intrinsic lung disease or upper airway obstruction, leads to a succession of adverse effects, including tissue hypoxia, lactic acid production, and metabolic acidosis. Initially the patient attempts to compensate by increasing the respiratory effort and rate; when this can no longer be sustained, the patient develops progressively worsening hypoxemia and acidosis, resulting eventually in full cardiopulmonary failure. By contrast, ventilatory dysfunction impairs excretion of CO2 (hypercarbia), producing a respiratory acidosis. This is generally a more gradual process and results from conditions that inhibit the normal functioning of the “respiratory pump” (i.e., those systems of the body responsible for producing effective ventilation) (see also Chapter 16). With progressively increasing levels of CO2 in the blood, fatigue and mental status depression cause respiratory effort to wane and finally cease altogether. The patient develops worsening hypoxemia as well as hypercarbia, profound acidosis, and ultimately cardiac arrest.
Circulation
Although adults typically have abrupt cardiovascular collapse caused by arrhythmia as a prelude to arrest, pediatric patients often experience a more gradual worsening of circulatory compromise and shock. Simply stated, the shock state is characterized by delivery of oxygen and substrate to tissues that is insufficient to meet metabolic demands. This outcome depends on the circulating blood volume, cardiac output, and vasomotor tone, which are continually regulated to maintain tissue perfusion. Significant changes in any one of these variables result in altered function of the others as the body attempts to maintain an adequate blood pressure. For example, a drop in vasomotor tone (e.g., septic shock) is accompanied by an elevated cardiac output through increased heart rate and stroke volume; significant blood loss or dehydration causes both increased vasomotor tone and increased cardiac output. In addition, compensatory mechanisms, such as the release of endogenous catecholamines, also operate to maintain an adequate blood pressure despite compromise to one or more of these physiologic mechanisms. As shock progresses, however, these compensatory mechanisms become inadequate, and the patient develops global deficits in oxygen and substrate delivery to vital organs, resulting in multiple organ system failure and eventually cardiopulmonary arrest.
For purposes of discussion, shock is divided into three general categories: hypovolemic, distributive, and cardiogenic. Hypovolemic shock, the most common type in the pediatric age group, is characterized by inadequate intravascular volume, resulting in a diminished stroke volume and cardiac output. Dehydration and hemorrhage are by far the most common causes, but “third-spacing” of fluids (i.e., sequestering fluids within the body but outside the cardiovascular system) can also cause hypovolemic shock. Distributive shock is characterized by peripheral vasodilatation, resulting in a drop in systemic blood pressure. The cardiac output may be normal or even elevated, but the maldistribution of blood flow causes inadequate perfusion of vital organs. This type of shock is seen in anaphylaxis, certain drug ingestions, and early sepsis. As septic shock progresses, significant intravascular fluid losses occur as well as myocardial dysfunction, and thus in the later phases of septic shock, a low cardiac output state also develops. Cardiogenic shock is characterized by myocardial dysfunction. Even though the intravascular volume and heart rate may be adequate or even increased, poor myocardial contractility results in a diminished stroke volume and diminished cardiac output. Cardiogenic shock is usually seen in patients with underlying heart disease (congenital or acquired) and metabolic derangements such as acidosis, hypoxia, hypoglycemia, and hypocalcemia.
The first scientific reports of cardiac compression were published in the late 19th and early 20th centuries. The impetus for this work was the occurrence of sudden, unexpected death of patients receiving chloroform anesthesia. Open cardiac massage was initially advocated for treating arrest patients (26,27), but this proved to be fraught with complications and required the expertise of highly trained physicians. The method of closed chest compression was initially described by Boehm in 1878 using laboratory animals (28). Further reports on this technique appeared sporadically over the next 70 years, but it was not until the work of Kouwenhoven et al., published in 1960, that closed chest cardiac compression was considered a potential intervention for arrest patients (23).
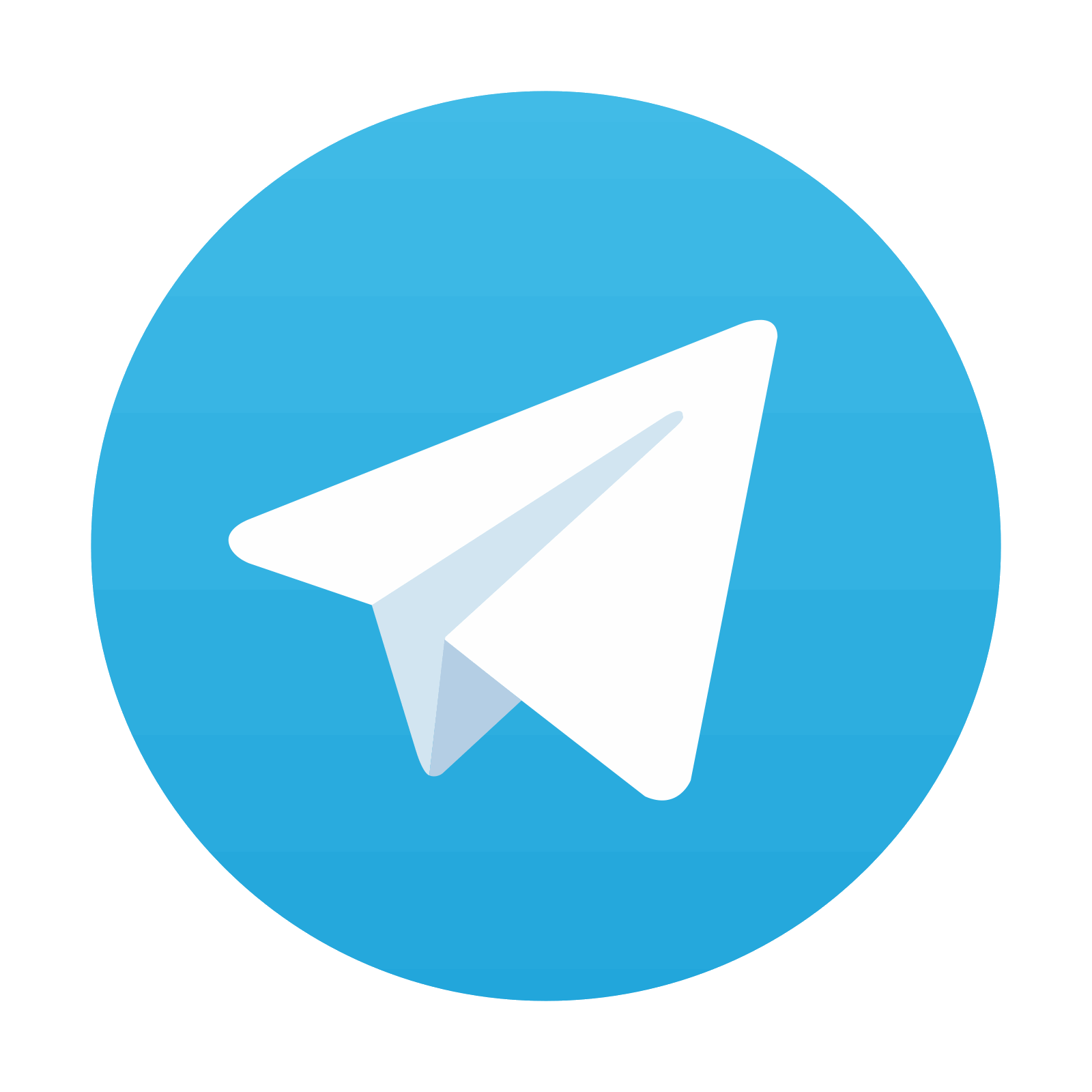
Stay updated, free articles. Join our Telegram channel
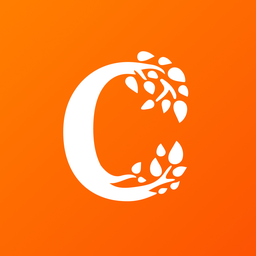
Full access? Get Clinical Tree
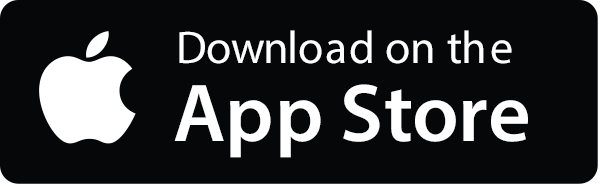
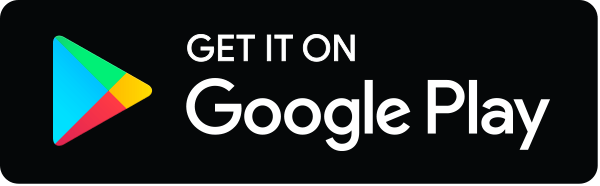