Assessment of Neonatal Pulmonary Function
Most neonates requiring intensive care present with respiratory symptoms. Although standard techniques for assessing pulmonary function can be applied in a healthy infant, special limitations and problems are encountered in very small or sick neonates. Methods have been developed to evaluate pulmonary function in neonates with suspected abnormalities of the cardiopulmonary system. This section presents a practical and clinical approach to disordered cardiopulmonary function and attempts to help distinguish between heart and lung disease.
Clinical Observations
Five common physical signs relay indirect information regarding pulmonary function: respiratory rate, retractions, nasal flaring, grunting, and cyanosis.
Respiratory Rate
Precise monitoring of respiratory rate is invaluable, as deviations from the normal respiratory patterns have been observed with mechanical pulmonary dysfunction, acid-base imbalances, and arterial blood gas abnormalities. During spontaneous breathing, infants can only achieve successful gas exchange within a limited range of respiratory rates. At low rates, decreased alveolar minute ventilation may occur, while at high rates and corresponding low tidal volumes (Vt), a large proportion of the minute ventilation is wasted in ventilating dead space.
Infants may also adjust the respiratory rate to minimize work of breathing. The total work of breathing consists of elastic and resistive components. The elastic component represents the work required to stretch the lungs and chest wall during a tidal inspiration, and the resistive component is the work required to overcome friction caused by lung tissue movement and gas flow through the airways. Healthy infants rely on tidal volumes of 6 to 7 mL/kg and approximate respiratory rates of 40 to 60 breaths per minute. However, during the first hour after birth respiratory rates may be higher, with approximately 50% of healthy newborns having respiratory rates greater than 60 breaths per minute.41 Newborns with stiff lungs, such as those with respiratory distress syndrome (RDS), attempt to compensate for the increased workload with rapid shallow breathing, whereas patients with increased resistance (e.g., subglottic stenosis) usually exhibit slower and deeper breathing (Figure 71-1).
Retractions
The neonatal chest wall is extremely compliant, and substernal, subcostal, and intercostal retractions are readily observable even with a relatively minor derangement in lung mechanics. Retractions are caused by negative intrapleural pressure-generated by the contraction of the diaphragm and other respiratory muscles and the mechanical properties of the lungs and chest wall. In neonates with respiratory distress, retractions become more apparent as the lungs become stiffer.
Apart from their characteristic appearance in RDS and other pulmonary diseases, severe retractions can signal complications of respiratory disease such as airway obstruction, misplacement of an endotracheal tube, pneumothorax, or atelectasis (see Chapter 72). Decreased retractions in the presence of adequate inspiratory effort suggest that lung compliance and/or airway caliber is improving.
Nasal Flaring
Nasal flaring is another sign of respiratory distress often observed in infants. The dilation of nostrils produced by contraction of the alae nasi muscles results in a marked reduction in nasal resistance. Because newborns are preferential nose breathers and because nasal resistance contributes substantially to total lung resistance, nasal flaring markedly decreases the work of breathing. Nasal flaring is occasionally observed in the absence of other signs of respiratory distress, particularly during feeding and active sleep.
Activation of other respiratory muscles, such as the genioglossus (tongue) and laryngeal muscles, is also important for optimal function of the upper airway. The genioglossus muscle protrudes the tongue and, in part, maintains pharyngeal patency, whereas the laryngeal muscles move the vocal cords and regulate airflow, particularly during expiration.
Grunting
With normal breathing, the vocal cords abduct to enhance inspiratory flow. In some respiratory disorders and with initiation of air breathing after birth, neonates attempt to close (adduct) their vocal cords during the initial phase of expiration, holding gas in the lungs and producing an elevated transpulmonary pressure in the absence of airflow. The elevated pressure and corresponding increased lung volume result in enhancement of the ventilation-perfusion ratio (). During the last part of the expiratory phase, gas is expelled from the lungs against partially closed vocal cords, causing an audible grunt.
Grunting may be either intermittent or continuous, depending on the severity of lung disease. Grunting can maintain functional residual capacity (FRC) and partial pressure of arterial oxygen (Pao2) equivalent to the application of continuous distending pressure. Because endotracheal intubation abolishes grunting, maintenance of lung volume with positive end-expiratory pressure (PEEP) is important following intubation.
Cyanosis
Central cyanosis, best observed by examining the tongue and oral mucosa, is an important indicator of impaired gas exchange. Clinical detection of cyanosis depends on the total amount of desaturated hemoglobin. Thus, patients with anemia may have a low Pao2 without clinically detectable cyanosis, and patients with polycythemia may be clinically cyanotic despite a normal Pao2. Peripheral cyanosis may be normal in neonates, but also occurs in situations of decreased cardiac output. The clinical features of cyanosis and their significance are discussed in detail in Part 13, The Cardiovascular System.
Cardiovascular Assessment
Blood Gas Measurements
Maintaining optimal gas exchange is the primary function of the lung. Thus, pulmonary evaluations should include blood gas estimates in addition to measurements of pulmonary mechanics. Both invasive and noninvasive blood gas measurements are available in the neonatal population. Invasive blood gas measurements are the optimal mode for accurate measures of oxygen and carbon dioxide, whereas noninvasive measurements are ideal for continuous documentation of the rapid changes that occur in this population. These alterations began with the initiation of the first breath after normal delivery, resulting in a rapid fall in Paco2 within minutes of birth. During this time, Pao2 rises quickly to levels of 60 to 90 mm Hg, although some degree of mismatching of is evident in the first 1 to 2 hours of life. This is believed to be the result of intracardiac and pulmonary right-to-left shunting. More recent data in larger groups of infants have characterized the increase in oxygenation following birth by way of pulse oximetry, allowing for further differentiation between preductal and postductal levels (Figure 71-2).49 The speed with which pulmonary ventilation and perfusion are uniformly distributed is an indication of the neonate’s remarkable capacity for maintaining homeostasis.

During the early weeks of postnatal life, preterm infants are exceptionally unstable, requiring close monitoring of blood gas status. Pulse oximetry and blood gas measurements are the most widely used clinical methods for assessing pulmonary function in neonates and form the basis for diagnosis and management of cardiorespiratory disease.
Invasive Blood Gas Measurements
Partial Pressure of Alveolar Oxygen and Carbon Dioxide
Changes in partial pressure of alveolar oxygen (Pao2) and Paco2 can identify disordered pulmonary function in various clinical situations. Pao2 and Paco2 values depend on the composition and volume of alveolar gas, the composition and volume of the mixed venous blood, and the mechanisms of pulmonary gas exchange impairment. Alveolar gas composition can be obtained from the equation

where PiO2 is partial pressure of inspired oxygen (PiO2 = Fio2 × [barometric pressure − water vapor pressure]). At sea level, barometric pressure is 760 mm Hg; at 100% humidity, water vapor pressure is 47 mm Hg. Paco2 is the partial pressure of alveolar carbon dioxide, and R is the respiratory quotient (usually 0.8). The mechanisms of pulmonary gas exchange impairment include mismatch, shunt, hypoventilation, and diffusion limitation. Appropriate matching of the alveolar gas with the mixed venous blood yields optimal gas exchange. Mixed venous blood composition and volumes are determined by the arterial blood gas content, cardiac output, oxygen consumption, and carbon dioxide production.
Techniques of blood gas determination in infants with RDS are discussed in Chapter 72. Gas exchange during assisted ventilation is discussed in Chapter 73. Acid-base physiology is discussed in Chapter 44.
Partial Pressure of Arterial Oxygen
Depending on the efficacy of gas exchange, the alveolar oxygen partial pressure (tension) tends to equilibrate with the mixed venous blood, resulting in the Pao2, which determines the degree of oxygen saturation of hemoglobin. In addition to its chemical combination with hemoglobin, oxygen is also dissolved in plasma and red blood cells (RBCs). Most of the oxygen in whole blood is bound to hemoglobin (measured clinically as oxygen saturation), whereas the amount of dissolved oxygen is only a small fraction of the total quantity carried in whole blood.
The quantity of oxygen bound to hemoglobin depends on the Pao2 and the oxygen dissociation curve (Figure 71-3). The blood is almost completely saturated at a Pao2 of 90 to 100 mm Hg. The flattening of the upper portion of the S-shaped dissociation curve makes it virtually impossible to estimate oxygen tension greater than 60 to 80 mm Hg by using arterial oxygen saturation alone. The dissociation curve of fetal hemoglobin (compared with adult hemoglobin) is shifted to the left, and at any Pao2 less than 100 mm Hg, fetal blood binds more oxygen. The shift appears to be the result of the lower affinity of fetal hemoglobin for 2,3-diphosphoglycerate. Note that pH, Paco2, temperature, and diphosphoglycerate (DPG) content influence the position of the dissociation curve.

Arterial oxygen content (Cao2) is the sum of hemoglobin-bound and dissolved oxygen, as described by the following equation:

where the arterial oxygen content is in mL/100 mL of blood, 1.37 is the approximate amount of oxygen (in milliliters) bound to 1 g of hemoglobin at 100% saturation, Hb is hemoglobin concentration (g/100 mL), Sao2 is the percentage of hemoglobin bound to oxygen, and 0.003 is the solubility factor of oxygen in plasma (mL/mm Hg). In this equation, the first term (1.37 × Hb × Sao2) is the amount of oxygen bound to hemoglobin. The second term (0.003 × Pao2) is the amount of oxygen dissolved in plasma and red blood cells.
Most of the oxygen in the blood is carried by hemoglobin. For example, if an infant has a Pao2 of 80 mm Hg, an Sao2 of 99%, and a hemoglobin concentration of 15 g/100 mL, Cao2 is the sum of oxygen bound to hemoglobin ([1.37 × 15 × 99]/100 = 20.3 mL) plus the oxygen dissolved in plasma (0.003 × 80 = 0.24 mL). In this example, just over 1% of oxygen in blood is dissolved in plasma and almost 99% is carried by hemoglobin.
The partial pressure of oxygen in arterial blood not only depends on the ability of the lungs to transfer oxygen as determined by alveolar ventilation, but also is largely influenced by the . For normal gas exchange, the ventilation and perfusion should be proportional. The ratio should be very close to 1 : 1; that is, for every milliliter of gas that passes the alveoli, there should be a proportional volume of blood in the pulmonary capillary bed. If the
is decreased (as in RDS), only partial oxygenation and CO2 removal from the mixed venous blood will occur. Oxygen supplementation can largely overcome the hypoxemia when the
is decreased. If the
is high, as in overventilation, partial pressure of oxygen is increased slightly.
The mechanism of shunting becomes evident when blood bypasses the alveoli, as occurs in congenital cyanotic heart disease, persistent pulmonary hypertension, or atelectasis. Oxygen supplementation does not prevent the hypoxemia produced by such a shunt. Hypoventilation (e.g., caused by apnea) is a common cause of hypoxemia. Diffusion limitation can affect oxygenation slightly, but this mechanism is not a common cause of severe hypoxemia in neonates. Hypoxemia caused by either hypoventilation or diffusion limitation usually can be treated easily with oxygen supplementation.
Three indexes can be used to estimate the degree of oxygenation derangement (see Chapter 78). The arterial-alveolar oxygen tension ratio (Pao2/Pao2 or a/ao2 ratio) has no units, decreases with worsening oxygenation, and can be obtained from the equation

where R is the respiratory quotient. Because [Fio2 + (1 − Fio2)/R] approximates 1.0 and Paco2 approximates Paco2, the equation can be simplified as follows:


or

The oxygenation ratio is expressed in mm Hg, decreases with worsening oxygenation, and can be obtained from the equation

The oxygenation ratio is less often used because it is subject to inaccurate assessment of the oxygenation derangement when Paco2 varies markedly. Often it is necessary to correct the degree of oxygenation for the ventilatory support because oxygenation is strongly influenced by mean airway pressure () during assisted ventilation. The oxygenation index (OI) is useful under these circumstances. The OI, which increases with worsening oxygenation or increasing
, has units of centimeters of water per mm Hg and can be obtained by the equation

Partial Pressure of Arterial Carbon Dioxide
Paco2 is an important measure of pulmonary function in neonatal respiratory disease. Mechanisms responsible for impairment of pulmonary exchange of CO2 include hypoventilation, mismatch, and shunt. In addition, an increase in dead space, as occurs with alveolar overdistention or gas trapping, impairs CO2 elimination. Because of the high solubility coefficient of CO2, diffusion limitation rarely affects CO2 exchange. As tissue levels of CO2 increase above those of arterial blood, molecules diffuse into the capillaries and are transported in RBCs and plasma. Unlike the S-shaped dissociation curve for O2, the relationship between CO2 tension and content is almost linear over the physiologic range.
Noninvasive Blood Gas Measurements
Carbon Dioxide
Noninvasive estimates of CO2 by end-tidal or transcutaneous detectors provide a reasonable alternative to frequent blood gas sampling, allowing for continuous monitoring of CO2 over prolonged periods of time. End-tidal CO2 monitoring is a noninvasive technique that measures CO2 concentration in the gas at the end of expiration. Quantitative measurements of end-tidal CO2 have been shown to correlate well with arterial blood gases in term and preterm infants without pulmonary disease and can be useful for detection of rapid changes in exhaled CO2 with data displayed on a breath-by-breath basis.53 When there is minimal pulmonary disease, quantitative end-tidal CO2 monitoring can assist in detection of hypercapnia and hypocapnia. However, with small tidal volumes and high respiratory rates it may underestimate the true alveolar gas in some neonates. Alternative designs, such as disposable colorimetric end-tidal CO2 detectors, have a high diagnostic accuracy for confirmation of endotracheal tube placement.77 A positive end-tidal CO2 test confirms endotracheal tube placement, and a negative test strongly suggests esophageal intubation. However, many infants can have falsely negative results at birth.62
Transcutaneous CO2 detectors have been shown to be superior to end-tidal CO2 in ill neonates.53 However, sensor preparation, taping, and the need for changes in sensor location may limit transcutaneous CO2 usefulness. Despite the limitations of end-tidal and transcutaneous monitoring of CO2, these methods provide critically important continuous information pertaining to changes in the respiratory system and minimize the need for blood gas analysis.
Oxygen
Maintenance of oxygenation in an acceptable range requires constant monitoring that is not possible with intermittent blood gas measurements. This has led to the widespread implementation of pulse oximetry as a means of continuous noninvasive O2 monitoring (see Chapter 71). Pulse oximetry measures the amount of hemoglobin molecules that is bound with oxygen. It has a rapid response time and can be used as an estimate of Pao2 for detecting short intermittent hypoxic episodes. However, as shown by the oxyhemoglobin dissociation curve (see Figure 71-3), oxygen saturation levels should remain below 95% in infants requiring supplemental O2 as hyperoxic values of Pao2 cannot be distinguished beyond that range.
Pulse Oximetry: Optimal Oxygen Saturation Target Range.
The ideal oxygen saturation target range is currently unknown. The avoidance of oxygen saturations greater than 95% in very preterm infants is generally accepted as this target range has been associated with both increased pulmonary complications2 and retinopathy of prematurity. Two of three recent multicenter clinical trials have shown an increase in survival with an oxygen saturation target of 91% to 95% as compared to a target of 85% to 89%.61,67,72,76 Although there was increased retinopathy with a target of 91% to 95%, this target range did not increase blindness.75 Compounding the outcome of all three multicenter trials were the varying levels of success in maintaining saturation values within a given range36 and the increased number of intermittent hypoxemic events in the low (85% to 89%) target group.20 Studies have shown that the time in target67 and the patterns of intermittent hypoxia are associated with morbidity.19,21 Although many questions remained unanswered, based on the increased mortality associated with a lower target of 85% to 89%, it appears prudent to maintain an oxygen saturation target in the 90% to 95% range8,56 and to minimize large swings in oxygen saturation. Studies combining pulse oximetry with automated adjustments in Fio2 show promise in maintaining oxygen levels within the desired range, mainly by reducing exposure to hypoxemia.7 However, the long-term benefits and implications of new patterns of oscillations in oxygenation induced by automated control systems have yet to be determined.
Pulse Oximetry: Screening for Critical Congenital Heart Disease.
About 25% of infants with critical congenital heart disease (those who require surgery or catheter intervention during the first year of life) may not be symptomatic and diagnosed until after initial discharge from the newborn nursery if oxygen saturation screening is not performed. Multiple cohort studies have shown a reasonable detection rate of critical congenital heart disease with oxygen saturation screenings. A sensitivity of around 70% to 75% with a specificity and negative predictive value of about 99.9% have been reported using a cutoff oxygen saturation of usually less than 95% at greater than 24 hours after birth as abnormal.45,74 The sensitivity is not higher because oximetry screening is less effective at identifying newborns with obstructive left heart lesions, including hypoplastic left heart syndrome, aortic stenosis, and coarctation of the aorta. Overall, pulse oximetry is highly specific for detection of critical congenital heart disease. Pulse oximetry newborn screening before hospital discharge is widely employed in the United States.
Pulse Oximetry: Perfusion Index.
The perfusion index (PI), a noninvasive estimate of tissue perfusion, is obtained by comparing the pulsatile signal with the nonpulsatile signal of the oxygen saturation waveform.59 A perfusion index of less than or equal to 1.24 has been shown to be predictive of high illness severity in newborn infants.17
Hyperoxia-Hyperventilation Test
The hyperoxia test aids in differentiating between primary lung disease and congenital heart disease with right-to-left shunting. The test is performed by placing the infant in 100% oxygen for 5 to 10 minutes followed by monitoring oxygenation by arterial blood gas or noninvasive measures (see Chapter 72). In patients with primary lung disease, oxygen should diffuse into the poorly ventilated areas and improve oxygenation by 5 to 10 minutes of oxygen exposure. Persistent hypoxemia after this time period would suggest the presence of right-to-left shunting.
Evaluation of Shunting
Desaturated blood from the right side of the heart enters the main pulmonary artery and crosses the ductus arteriosus to the descending aorta when right-to-left ductal shunting occurs. Because the ductus arteriosus almost always enters the aorta after the origin of the right subclavian and carotid arteries, blood arriving at these two sites is well oxygenated, whereas blood from the aorta, and usually the left subclavian artery, is less oxygenated if there is a right-to-left ductal shunt. Thus preductal blood gases can be obtained from the right radial artery, whereas blood in the descending aorta, and usually the left radial artery, can include blood of postductal origin. Alternatively, placement of two pulse oximeters (one on the right hand and the other on the left hand or either foot) accomplishes the same effect in differentiating preductal and postductal Pao2 or oxygen saturation.
Patients with PPHN sometimes have right-to-left shunting through the foramen ovale and the ductus arteriosus. Ductal shunting can be demonstrated by the presence of a simultaneous oxygenation gradient between preductal and postductal arterial blood, whereas foramen ovale shunting affects both preductal and postductal oxygenation. Echocardiography can be used to confirm foramen ovale and ductal shunts.
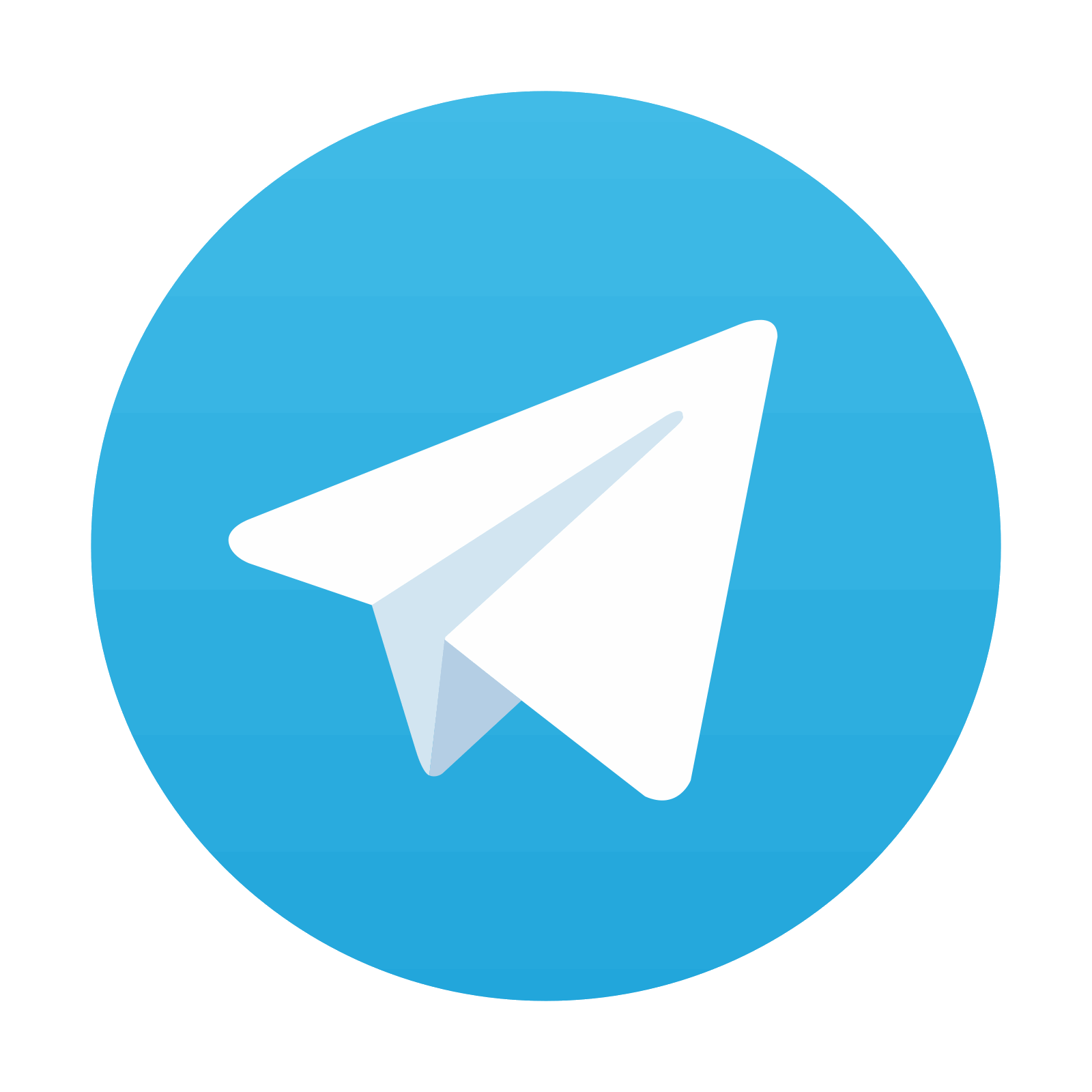
Stay updated, free articles. Join our Telegram channel
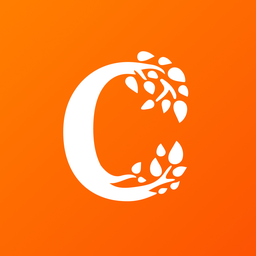
Full access? Get Clinical Tree
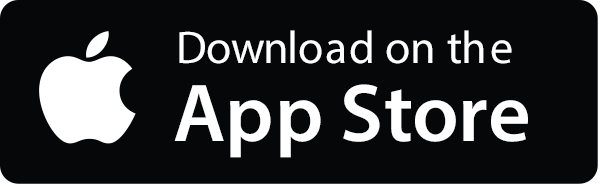
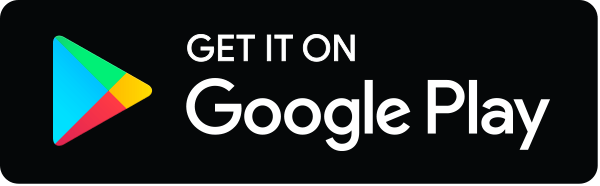