KEY POINTS
- 1.
We still need a universally accepted definition of severe hyperbilirubinemia; no particular thresholds of unconjugated or total bilirubin levels have been definitively associated with acute or chronic bilirubin encephalopathy.
- 2.
Several measurements can be useful for the assessment of hemolysis, such as those of end-tidal carbon monoxide, blood levels of analytes such as carboxyhemoglobin and haptoglobin, reticulocyte percent and number, cell-free hemoglobin, and hemoglobinuria.
- 3.
Hemolytic causes of hyperbilirubinemia include alloimmune hemolytic diseases. Nonimmune negative hemolytic disease can arise in red blood cell (RBC) enzymopathies, RBC membrane disorders, and globin disorders.
- 4.
Phototherapy is the standard of care for all cases of hyperbilirubinemia. In severe hyperbilirubinemia, exchange transfusion may also be indicated. Efforts are ongoing to evaluate whether intravenous immunoglobins, metalloporphyrins, and phenobarbital might be useful in specific conditions.
Overview
There is no universal definition of severe hyperbilirubinemia because no threshold of laboratory values has been definitively associated with acute or chronic bilirubin encephalopathy. Some groups have suggested that the unconjugated fraction of bilirubin alone can cross the blood-brain barrier and cause bilirubin encephalopathy, and therefore only the unconjugated bilirubin fraction should be considered when evaluating a neonate for treatment of hyperbilirubinemia. Others have suggested that it is the unconjugated bilirubin, not bound to plasma proteins (e.g., albumin), that is more robustly associated with bilirubin encephalopathy. , Because none of these hypotheses have been upheld with uncontroversial evidence, we utilize the total serum bilirubin measurement in our evaluation of neonatal hyperbilirubinemia.
Understanding the evaluation and treatment of severe neonatal indirect hyperbilirubinemia is simplified by understanding the fundamental steps in heme metabolism and bilirubin conjugation and excretion ( Fig. 42.1 ). These crucial steps include (1) red blood cell (RBC) lysis or death, (2) the conversion of heme into biliverdin by heme oxygenase, and (3) the intrahepatic conjugation or glucuronidation of bilirubin by uridine diphosphate (UDP) glucuronosyl transferase (UGT1A1). In the most simple terms, hyperbilirubinemia is the result of either (1) excessive bilirubin production, (2) decreased bilirubin metabolism or elimination, or (3) a combination of the two. Aberrations in these crucial steps result in any of these broad etiologies.

This chapter will explore both well-established and innovative approaches to the evaluation and treatment of severe hyperbilirubinemia. Ultimately, the goal of evaluation and treatment of severe hyperbilirubinemia is to prevent bilirubin-induced neurologic dysfunction.
Determining Causes of Severe Hyperbilirubinemia
Determining the cause of severe indirect hyperbilirubinemia can have implications on immediate and long-term management of infants. Historically, our ability to identify the cause of severe hyperbilirubinemia has been poor. In the US voluntary Kernicterus Registry, conducted in the 1990s by Drs. Bhutani and Johnson, no clear explanation for the hyperbilirubinemia was identified in 55% of the 125 patients in the registry. Christensen et al. reported similar findings retrospectively in the Intermountain Healthcare system in the Western United States, where among 302,399 live births, 32 neonates had a total serum bilirubin >30 mg/dL. Of these 32, only 11 (65.6%) had an explanation found for their extreme hyperbilirubinemia and the remaining patients were listed as having “idiopathic” jaundice.
Subsequently, in a pilot registry in the state of Utah, Christensen successfully identified the cause of hyperbilirubinemia in all cases of acute bilirubin encephalopathy between 2009 and 2018 (seven cases). Two cases were caused by immune-mediated hemolysis. The remaining five had genetic mutations causing increased bilirubin production through hemolytic mechanisms. Two of these five patients also had a genetic mutation retarding bilirubin conjugation (Gilbert syndrome). This study, although small, illustrated the growing availability and value of genetic sequencing in seeking the diagnosis of Coombs-negative hemolytic jaundice (and even nonhemolytic jaundice). At least two laboratories in the United States offer next-generation sequencing panels designed to diagnose genetic causes of severe hyperbilirubinemia: ARUP Laboratories (Salt Lake City, Utah) and Mayo Clinic Laboratories (Rochester, Minnesota). Although these panels are labeled “hereditary hemolytic anemia” panels, they both also include genes involved in bilirubin transport, conjugation, and excretion. Due to cost, we reserve these panels for more severe cases when a narrower differential diagnosis does not lead us to a more directed approach to diagnosis.
Our general approach to evaluating severe hyperbilirubinemia is to first determine whether hemolysis (increased bilirubin production) is a contributing factor, keeping in mind that the presence of hemolysis does not exclude coinheritance of mutations that slow bilirubin elimination. In fact, it is our experience that cases where increased bilirubin production and retarded bilirubin elimination are both present result in the most severe hyperbilirubinemia. Determining whether the rate of bilirubin production is significantly increased will then help refine the differential diagnosis and guide the approach to the patient ( Fig. 42.2 ). Here, we will first discuss tools used to differentiate hemolytic from nonhemolytic jaundice. Then we will discuss the most common hemolytic and nonhemolytic causes of severe hyperbilirubinemia.

Assessing for Hemolysis
End-Tidal Carbon Monoxide (ETCO)
Elevated carbon monoxide levels in neonates with erythroblastosis fetalis was first described in the 1971 by Jeffrey Maisels in the laboratory of David Nathan. Carbon monoxide (CO) is generated in equimolar amounts to bilirubin as heme is metabolized to bilirubin. Thus ETCOc (end-tidal carbon monoxide [CO] “corrected,” which is end-tidal CO minus ambient CO) in exhaled breath can be used to quantify the hemolytic rate. For many years, measurements of CO in the blood were only available by gas chromatography, and devices to measure CO in the breath did not exist. Portable devices are now available to obtain an accurate measurement of the ETCO noninvasively, at the patient’s bedside, in less than 2 to 3 minutes. A wealth of literature supports the use of ETCOc measurement devices to quantify the rate of hemolysis in a patient and therefore identify patients with hyperbilirubinemia for whom increased hemolysis is a contributing factor. Of the techniques described here, we consider ETCOc determinations to be the most helpful because they are sensitive and accurate, require little time, and require no phlebotomy. Based on a publication by Christensen et al. in 2015, we consider an ETCOc measurement >1.7 ppm in a term newborn in the first week of life to be consistent with hemolysis. Beyond 1 week, ETCOc determinations >1.0 ppm should raise concern for hemolysis.
Blood Carboxyhemoglobin
Carboxyhemoglobin levels can be measured directly in the blood and have utility to detect increased hemolysis similar to the ETCOc measurement. Carboxyhemoglobin values are often obtained from a blood gas by cooximetry and are reported as a percentage of hemoglobin “saturated” with CO. The upper limit in the newborn has been reported between 1.5% and 2.0%. Although this measure is not sensitive, it seems to be specific when elevated above 2.0%.
Serum Haptoglobin Concentration
Haptoglobin is a protein synthesized by the liver that is used to scavenge and recycle free hemoglobin. Once plasma hemoglobin is bound to haptoglobin in a haptoglobin-hemoglobin complex, it is rapidly cleared from the circulation. Therefore depletion of serum haptoglobin can be a sign of excess cell-free hemoglobin resulting from hemolysis. However, haptoglobin levels are normally lower in the newborn than in a child or adult. Therefore the validity of the serum haptoglobin concentration as a marker of hemolysis is variable. We judge that in a neonate, a serum haptoglobin level that is falling or is below the lower limit of detection is consistent with hemolysis.
Reticulocyte Percentage and Number
Reticulocytosis is the physiologic response to hypoxia due to anemia. At the time of delivery, the newborn is exposed to a relative hyperoxic environment that suppresses erythropoietin production and results in a decrease in red cell production and decreased reticulocyte production. Therefore the reference interval for reticulocyte percentage or number in the newborn is highly dependent on postnatal age. Because anemia may not be a result of ongoing RBC destruction, reticulocytosis is not specific for hemolysis. A reticulocyte percentage >15% can support the diagnosis of hemolysis but could also arise several days after severe hemorrhage. The immature reticulocyte fraction, reported as a percentage, is an automated reticulocyte parameter that quantifies the proportion of reticulocytes that have a particularly high RNA fraction by flow cytometry. It provides an early and sensitive index of marrow erythropoietic activity and therefore may be even more sensitive than standard reticulocyte measurements. Immature reticulocyte fraction values greater than 7% at birth or >3% after 24 hours of life are consistent with a diagnosis of hemolysis.
Hemoglobinuria
Cell-free hemoglobin should be uncommon in the healthy newborn. Therefore, trace hemoglobinuria or greater is evidence of hemolysis.
Hemolytic Causes of Hyperbilirubinemia
Alloimmune Hemolytic Diseases of the Newborn
The primary differentiation to be made when determining causes of hemolytic hyperbilirubinemia is whether or not the process is immune-mediated (see Fig. 42.2 ). Historically, there has been significant emphasis placed on alloimmune-mediated hemolysis as the primary cause of severe neonatal hyperbilirubinemia (hemolytic disease of the fetus and newborn [HDFN]). This is likely due to the mortality burden of HFDN prior to the introduction of Rh(D) immune globulin (RhIg) in 1968. Although alloimmunity remains a serious and common cause of hyperbilirubinemia, its frequency in North America and Europe has decreased dramatically.
Some hospitals in the United States and Canada perform a blood type and antibody screen on all newborns born to mothers with blood type O. In the case of a mother who has a positive antibody screen, significant concern for the welfare of the fetus and newborn is warranted. The 2004 American Academy of Pediatrics guidelines, though, state that if the maternal blood group is O (+), it is an option to test the cord blood for the infant’s blood type and to perform a direct antiglobulin test (DAT), but it is not a requirement to do so provided there is appropriate surveillance. Recent studies by our group and others support eliminating routine blood typing and DAT in neonates born to blood group O (+) mothers in the absence of a positive antibody screen. We found that neonates who were blood group A or B and born to mothers of group O (+) (and thus were at some risk for ABO hemolytic disease) did not have a higher incidence of severe neonatal hyperbilirubinemia than did neonates of blood group O who were born to group O (+) mothers (and thus were at no risk for ABO hemolytic disease). We found more in-hospital phototherapy use among babies born to group O mothers compared with mothers of other blood groups. We suggested that this supports our theory that universal bilirubin screening of neonates in the birth hospital identifies those who need phototherapy, including those with significant ABO hemolytic disease, and that this obviates their need for routine blood type testing and DAT.
Because alloimmune HDFN is well-covered in other texts, this chapter will focus on DAT-negative causes of hemolysis in the newborn.
Nonimmune (DAT-Negative) Hemolytic Disease of the Newborn
There are many causes of increased RBC breakdown, and therefore increased bilirubin production, that are not due to alloimmunity. All of those described here have a genetic etiology that results in a shortened RBC life span and are called hereditary hemolytic anemias. They can be subdivided into the following groups: (1) RBC enzymopathies, (2) RBC membrane disorders, and (3) globin disorders. Here, we will further subdivide RBC enzymopathies into (1) enzymes involved in the hexose monophosphate (HMP) shunt and glutathione metabolism, (2) enzymes involved in the glycolytic pathway, and (3) enzymes involved in nucleotide metabolism.
RBC Enzymopathies—HMP Shunt and Glutathione Metabolism
The unifying characteristic of defects in the HMP shunt and glutathione synthesis and metabolism is the inability of the RBC to withstand oxidative stress. Therefore the phenotype of red cell morphologies observed in these disorders is similar. Glucose-6-phosphate dehydrogenase (G6PD) deficiency accounts for a vast majority of cases attributed to RBC enzymopathies in the HMP shunt.
Glucose-6-Phosphate Dehydrogenase Deficiency
G6PD is an enzyme in the HMP shunt pathway responsible for the oxidation of glucose-6-phosphate to 6-phosphogluconate, at the same time reducing NADP to NADPH. The HMP shunt results in the production of glutathione, an important antioxidant in RBCs. Inability to maintain adequate glutathione levels, especially as newborns transition to an oxygen-rich ex-utero environment at birth or encounter other oxidative stress, results in denatured and dysfunctional proteins leading to cell lysis and death.
G6PD is located within q28 of the X chromosome and therefore is inherited in an X-linked dominant fashion. Symptomatic patients are almost exclusively male, but female carriers with unfavorable lyonization can also be affected. It is the most common enzymatic disorder of RBCs and affects 400 million people worldwide. The geographic distribution G6PD deficiency prevalence is highly correlated with regions in which malaria was once endemic, leading to the hypothesis that G6PD deficiency may have conferred an advantage against malaria infection. Different genetic variants result in varying degrees of disease severity. Disease variants are named for and usually coincide with patients’ ethnicities.
The peripheral blood smear of a patient who has G6PD may reveal eccentrocytes, also known as “bite” or “blister” cells, which result from splenic removal of Heinz bodies. Special stains can reveal the presence Heinz bodies, which are collections of denatured globin chains often attached to the RBC membrane.
When G6PD is the suspected cause of hyperbilirubinemia, a quantitative enzyme measurement assay is the preferred initial screening test. This test is available at many reference laboratories. Values less than 10.0 U/g hemoglobin (Hgb) are concerning for G6PD deficiency. The quantitative enzyme assay is sufficient to confirm the diagnosis for G6PD. If the patient’s clinical course is consistent with the suspected variant based on ethnicity, further genetic sequencing is likely unnecessary. If the patient has refractory hyperbilirubinemia or anemia not expected based on ethnicity, then genetic sequencing may be indicated. Newborn physicians and hematologists should counsel parents of newborns with G6PD deficiency regarding drugs and foods to avoid prior to discharge. Our group schedules each child with uncomplicated G6PD deficiency in the hematology clinic between 2 and 3 months of life to recheck a complete blood cell count and provide additional counseling.
Glutathione Reductase Deficiency and Glutathione Synthetase Deficiency
Both glutathione reductase deficiency and glutathione synthetase deficiency are very rare diseases. Both result in increased RBC susceptibility to oxidative stress and present with phenotypes similar to G6PD deficiency.
RBC Enzymopathies—Glycolytic Pathway
The primary purpose of the glycolytic pathway, also known as the Embden-Meyerhof pathway, is the oxygen-independent production of energy. Enzymatic defects in this pathway result in common RBC morphologies suggestive of inadequate energy production.
Pyruvate Kinase Deficiency
Pyruvate kinase (PK) is responsible for the conversion of phosphoenolpyruvate to pyruvate with concomitant phosphorylation of adenosine diphosphate to adenosine triphosphate. PK deficiency is rare, with a prevalence estimated at <51 cases per million population. It has a worldwide distribution, but it is more common among people of northern European ancestry. Despite being rare, PK deficiency is the most common enzymopathy in the glycolytic pathway, and some have suggested that PK deficiency is the second most common hereditary variety of Coombs-negative, nonspherocytic hemolytic disease in the United States.
The PKLR gene located on chromosome 1q22 encodes both the liver and RBC isozymes. PK deficiency follows an autosomal recessive inheritance pattern (often compound heterozygosity), and as such, heterozygous carriers of PKLR mutations are asymptomatic. More than 260 different PKLR mutations have been described and associated with hemolytic anemias, most of which are single-nucleotide missense mutations.
Newborns with PK deficiency present with severe hyperbilirubinemia that can be difficult to control with phototherapy. Although the severity of symptoms and chronic anemia vary greatly, the disease is lifelong and may result in a need for chronic transfusions.
Other enzyme deficiencies of the glycolytic pathway have been described in case reports but are rare. Those described include aldolase, phosphofructokinase, phosphoglycerate kinase, glucose phosphate isomerase, hexokinase, and triosephosphate isomerase.
RBC Enzymopathies—Disorders of Nucleotide Metabolism
There are two main types of 5′‐nucleotidases found in erythrocytes. They are known as P5′N1 and P5′N2. Defects resulting in hemolytic anemia have only been described in P5′N1 (NT5C3A). , The nucleotides of normal red cells consist largely of purine derivatives, with very low levels of pyrimidine nucleotides. P5′N1 deficiency results in accumulation of pyrimidines because it converts pyrimidine nucleoside monophosphates into cytidine and uridine, which can diffuse across the cell membrane. Excessive pyrimidines are thought to have toxic effects on RBCs. Their accumulation and subsequent intracellular persistence of ribosomes/RNA results in basophilic stippling. Although this finding is sensitive for P5′N1 deficiency, it is clearly not specific for this condition. It is also seen in lead poisoning, sideroblastic anemia, and certain hemoglobinopathies. ,
More than 25 different mutations have been identified in NT5C3A. The majority of patients with P5′N1 deficiency are homozygous for a single mutation and the remainder are compound heterozygotes. The clinical manifestations of P5′N1 deficiency are lifelong mild‐to‐moderate hemolytic anemia accompanied by jaundice and splenomegaly.
Assaying for the presence of pyrimidine nucleotides serves as a surrogate marker for P5′N1 deficiency and is available at a few reference laboratories including the Mayo Clinic. Alternatively, the NT5C3A gene is included as part of the hereditary hemolytic anemia gene panels available at the Mayo Clinic and ARUP laboratories.
RBC Membrane Disorders
Disorders due to RBC membrane structural protein defects include hereditary spherocytosis (HS), hereditary elliptocytosis, and hereditary pyropoikilocytosis. The structural proteins involved in these disorders are closely related.
Hereditary Spherocytosis
HS is a disorder characterized by defects in RBC membrane proteins resulting in a loss of RBC membrane surface area and thus sphere-shaped, hyperdense, poorly deformable RBCs. The cells have a shortened life span, which causes a hemolytic anemia found worldwide in individuals from all races and ethnicities. HS is the most common inherited hemolytic anemia in people with Northern European ancestry, with an estimated incidence of 1 per 1000 to 1 per 3000 births. , Seventy-five percent of cases are inherited in an autosomal dominant manner and the remaining are inherited in an autosomal recessive manner or are from a de novo mutation. The most commonly mutated gene associated with HS in the Northern European population is ANK1 (40%–65%). Other genes where mutations cause HS include SPTA1 , SPTB , SLC4A1 , and EPB978-0-323-69415-5.
The practitioner should consider HS when a neonate has Coombs-negative jaundice, anemia, and microcytosis. Christensen and Henry in 2010 also showed that an mean corpuscular hemoglobin concentration (MCHC) >36 g/dL in newborns with a total serum bilirubin >20 mg/dL is both sensitive and specific for HS. In a subsequent publication by Christensen, the author estimated the ratio of mean corpuscular hemoglobin concentration to mean corpuscular volume (MCHC/MCV) >0.36 to be 97% sensitive and >99% specific for HS.
The traditional diagnostic test for HS has been osmotic fragility. However, more recently it has been shown that the diagnostic criteria for HS used in adults and older children are unreliable in newborn infants because in both preterm and term infants, RBC membranes have an increased osmotic resistance. A relatively new diagnostic test for HS, eosin-5-maleimide flow cytometry, quantifies the erythrocyte membrane band 3 complex using an eosin-5-maleimide dye flow cytometric analysis. , A reduction in band 3 complex is consistent with HS. Therefore the test is commonly referred to as “band 3 reduction” at reference laboratories that offer it. This test performs well in neonates even in the first days of life. , If the patient has no family history of HS or the causal mutation is unknown, sequencing the genes that can cause HS is indicated. Different mutations result in a variety of disease severities. Identifying the causal mutation can also aid in identification of the inheritance pattern and reproductive risk for the proband.
Nonhemolytic Causes of Indirect Hyperbilirubinemia
Most known nonhemolytic causes of indirect hyperbilirubinemia are due to either (1) impaired uptake into the hepatocyte or (2) impaired conjugation of the unconjugated bilirubin molecule, with the latter being much more common.
SLCO1B1 and SLCO1B3
Solute carrier organic anion transporter family members 1B1 and 1B3 (SLCO1B1 and SLCO1B3) encode proteins expressed on the hepatic cell membrane that facilitate the transport of bilirubin into the hepatocyte.
Gilbert Syndrome
Both Gilbert syndrome and Crigler-Najjar syndrome are caused by inadequate glucuronidation (conjugation) of bilirubin by UDP-glucuronosyltransferase (UGT1A1) . Gilbert syndrome is typically caused by genetic variants in the promotor region of UGT1A1. Therefore, in contrast to patients with Crigler-Najjar syndrome, patients with Gilbert syndrome produce UDP-glucuronosyltransferase enzymes that have normal function but are decreased in amount. The normal sequence of the TATAA element within the promoter of UGT1A1 is A(TA)6TAA, or simply (TA)6. The most common variant is a longer version of the TATAA sequence A(TA)7TAA, known as UGT1A1*28 , that reduces the production of UDP-glucuronosyltransferase due to decreased affinity of transcription factors to the promotor region. Traditional thought is that Gilbert syndrome manifests only in people who are homozygous for the variant promoter. As a result, its inheritance is most consistent with an autosomal recessive trait. However, heterozygotes for the Gilbert genotype also have a partially decreased UGT1A1 enzyme level. We observed moderate to severe hyperbilirubinemia in UGT1A1*28 heterozygotes when combined with another mildly hemolytic etiology (e.g., anti-A alloimmunization or heterozygosity for allele alpha-LELY in SPTA1 ). Epidemiologic studies of Gilbert syndrome (specifically, TA[7]) in Caucasian populations estimate a prevalence between 9.8% and 16%. Other alleles such as TA(5) and TA(8) are found in Caucasian populations but with much lower prevalence. These alleles can also result in decreased UGT1A1 expression, resulting in bilirubin levels above normal. Because of the high prevalence of UGT1A1 promoter polymorphisms, we find it that it is a common contributor to hyperbilirubinemia—often in combination with other bilirubin production or elimination aberrations.
Crigler-Najjar Syndrome
Crigler-Najjar can be divided into two types. Type I is characterized by absent uridine diphosphate glucuronosyltransferase (UGT) activity and little or no conjugated bilirubin. This results in early-onset, severe, lifelong indirect hyperbilirubinemia and is caused by a subset of mutations within UGT1A1 coding regions that result in essentially no functional UGT enzyme. Type II is characterized by varying degrees of decreased enzyme activity that are caused by less deleterious mutations in the coding regions of UGT1A1. Children with Crigler-Najjar Type I and those with persistent hyperbilirubinemia due to Crigler-Najjar Type II should be referred to a transplant hepatology center for evaluation and management.
In 2020, Straus and colleagues published their experience in caring for 28 children with Crigler-Najjar Type I over 30 years. Seventeen required liver transplantation. Prior to liver transplantation, neonates are phototherapy-dependent and require 15 to 20 hours per day on phototherapy.
Treatment of Severe Hyperbilirubinemia
Phototherapy is the standard of care for nearly all cases of hyperbilirubinemia. In cases of severe hyperbilirubinemia, especially those with signs of acute bilirubin encephalopathy, exchange transfusion may also be indicated. This section will briefly review evidence for or against less common therapies with unproven efficacy.
Intravenous Immunoglobulin
Because of the risks associated with exchange transfusions, intravenous immunoglobulin (IVIg) has been suggested as an alternative to avoid exchange transfusion. IVIg may reduce the rate of hemolysis by blocking the Fc receptors on macrophages that are thought to mediate destruction of RBCs coated with anti-D antibodies. The 2018 Cochrane Review (systematic review and meta-analysis) by Zwiers et al. examines the most relevant literature regarding IVIg for the treatment of alloimmune hemolytic disease of the newborn. That review identifies the only two published studies with sufficiently low risk of bias to assess the efficacy of the therapy: a 2011 study by Smits-Wintjens et al. conducted in the Netherlands and a 2013 study by Santos et al. conducted in Brazil. Both of those trials conclusively found no benefit of IVIg in reducing the need for and number of exchange transfusions in cases of Rh hemolytic disease. The authors of the Cochrane Review state that the seven other studies included in their analysis were observational and had a high risk of bias. Our group has concluded that the Netherlands and Brazil studies were sufficient to show that IVIg has little or no benefit in the treatment of alloimmune hemolytic disease of the newborn, and children with hyperbilirubinemia not controlled by phototherapy should be transferred to a center that is able to perform an exchange transfusion.
Metalloporphyrins
Currently, the only means available to reduce the hemolytic rate in neonates with severe hemolytic jaundice is to perform a double-volume exchange transfusion, removing hemolyzing erythrocytes and replacing them with donor adult erythrocytes. Metalloporphyrins are potent inhibitors of heme oxygenase, the rate-limiting enzyme in the production of bilirubin from heme. Two metalloporphyrins have been studied to assess their ability to decrease the risk of hyperbilirubinemia. Tin mesoporphyrin has been tested in animals and humans and has shown promising results. Zinc protoporphyrin has been studied in only animals. Unfortunately, these drugs are not approved for use in the United States and have not been produced for clinical use.
Phenobarbital
Phenobarbital can induce UGT activity and can promote conjugation of bilirubin to its water-soluble form. We reserve the use of phenobarbital for neonates with genetically proven Crigler-Najjar Type II (or strong suspicion of the disease, awaiting confirmation). Neonates with Crigler-Najjar Type I do not respond to phenobarbital therapy and this lack of response can be used to differentiate Type I from Type II.
REFERENCES
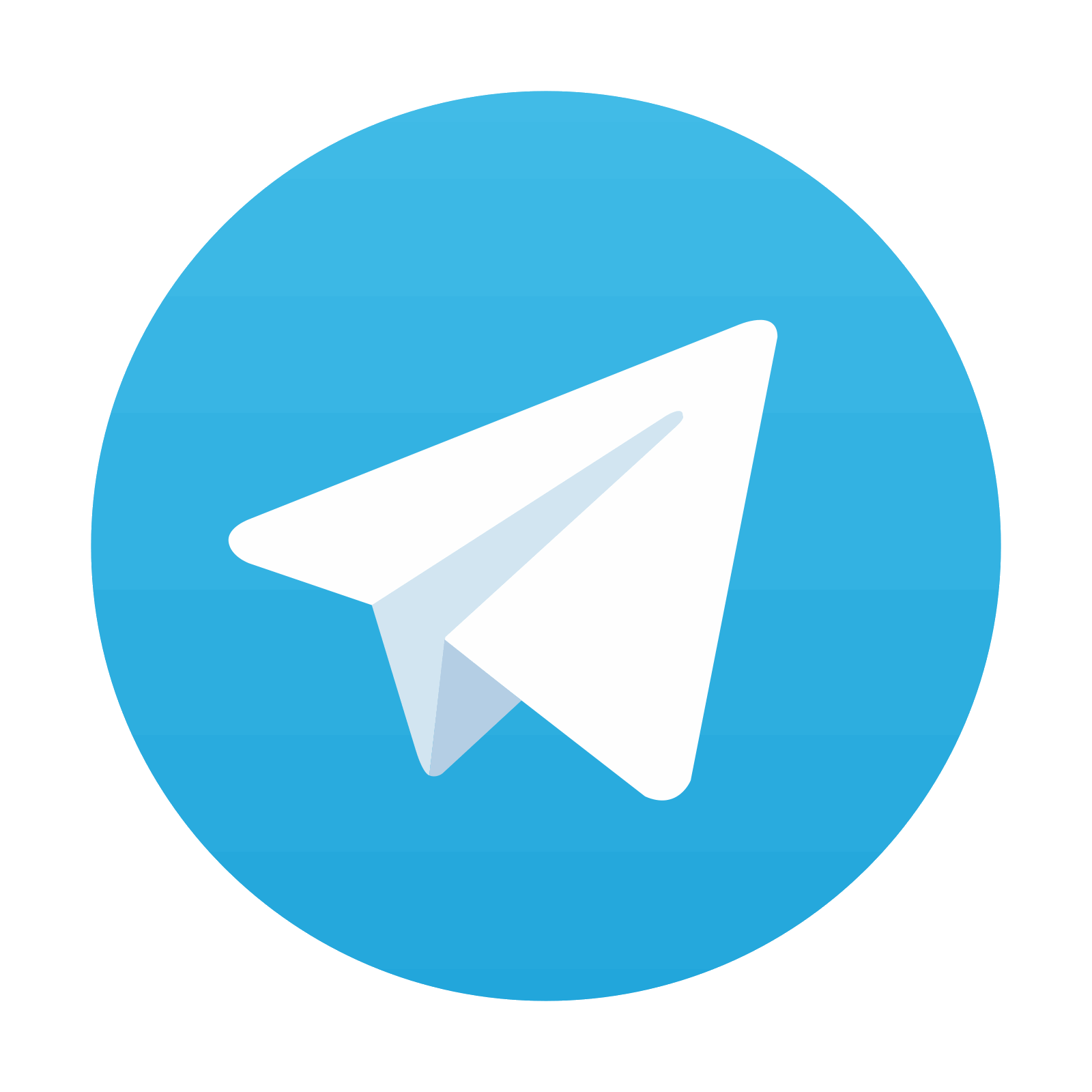
Stay updated, free articles. Join our Telegram channel
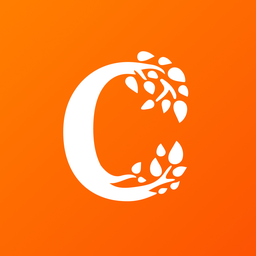
Full access? Get Clinical Tree
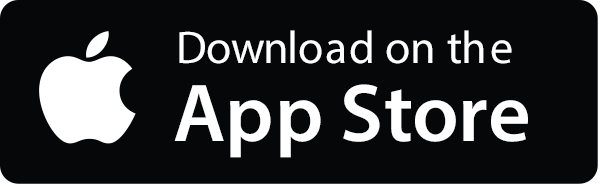
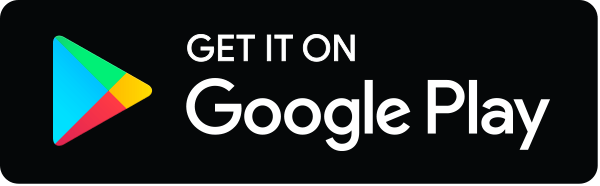
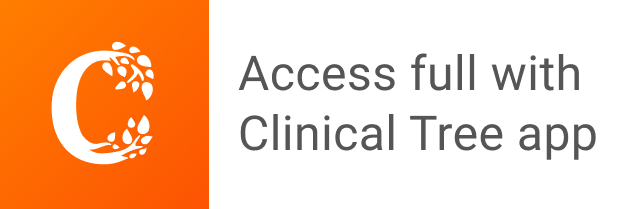