KEY TERMS
Key Terms
-
Three-dimensional ultrasonography (3DUS, or volume ultrasound): an imaging method that fundamentally converts the acquired digital 2D picture elements (pixels) into a 3D volume.
-
Four-dimensional ultrasonography: an imaging method that adds the dimension of time via the fast, and continuous, acquisition and display of 3DUS volumes, especially useful for imaging moving targets (ie, fetal heart, moving limbs, etc).
-
Multiplanar display: the interactive display of three perpendicular views (ie, sagittal, transverse, and coronal views) around a reference point, otherwise known as orthogonal display.
-
Voxel: short for volume pixel. The smallest unit of a three-dimensional volume, equivalent of a pixel in a 2D image.
-
Rendering: the display of voxels using postprocessing software highlighting either surface features (surface rendering) or internal anatomic structures (volume rendering).
-
Reformatting: the process of volume exploration, at times using postprocessing algorithms, where particular image planes are obtained in a multiplanar, rendered display, or both.
-
STIC (spatio-temporal image correlation): a volume analysis technique specifically designed to acquire and display moving volumes of the fetal heart.
-
Matrix 2D array (or electronic 4D probes): a transducer with a large number of piezoelectric elements (thousands) in rows, allowing electronic beam steering, and focusing, in all directions, avoiding the limitations of mechanically swept probes. They make possible rapid real-time acquisition of moving objects such as limbs or fetal heart.
-
Volume contrast imaging: a 3D image projection that provides excellent contrast resolution by combining several layers of voxels (ie, derived from multiple image slices in a rather thin volume acquisition), as compared to a single layer of pixels used in 2D ultrasonography.
-
Biplane imaging: provides simultaneous display of high resolution, high frame rate images in two perpendicular planes. A matrix 2D array can steer scan planes in perpendicular orientations allowing for acquisition, and true real-time display of two orthogonal planes.
INTRODUCTION
Ultrasound imaging in obstetrics and gynecology is commonly used for numerous indications. The most commonly used technology in everyday practice is the conventional high-resolution two-dimensional ultrasound (2DUS), and is widely considered clinically useful. Using 2DUS imaging technology, the operator scans the anatomy with a flat 2D ultrasound beam to obtain cross-sectional planes. These 2D imaging planes are typically quite thin, and the corresponding images obtained represent thin cross-sectional anatomical information along the path of the 2D beam. As they scan through the anatomy, ultrasound professionals typically orient themselves by mentally developing a spatial three-dimensional (3D) concept of the region of interest, accounting for depth, and perspective. Depending on fetal position, using 2DUS at times some image planes can be very difficult, or even inherently impossible to be obtained with conventional 2DUS techniques, especially if they are perpendicular (or close to) to the axis of the beam.
Research work on 3D volume acquisition and display methods started in 1970.1 Sophisticated early prototypes had static arms, later equipped with position sensors to more reliably register the acquired 2D data in time and space. Early to mid-1990s saw this technology starting to leave the industry’s research and development labs and become a more appealing clinical tool.2-6 Significant developments in transducer technologies, especially manually swept mechanical probes, electronic 2D arrays, miniaturization, graphic boards, active cooling, as well as advances in computer signal processing and image display finally made possible reliable techniques to acquire and visualize ultrasound volumes.7
Volume ultrasound imaging can depict the anatomy of the region of interest as static, or dynamic, in motion. The volume derived displays have become fully interactive environments where the operator has full control over selecting any scan planes, and evaluate the anatomy in user-selectable uniplanar, biplanar, orthogonal, multiplanar displays, as well as rendered 3D views, alone or in a combination with any of the aforementioned. We know that volume imaging techniques are not new, as computed tomography (CT) and magnetic resonance (MR) imaging has acquired and displayed volume-derived image slices in a volume for years. What sets apart 3DUS volume acquisition and display technologies is that they provide classic volume imaging methods to be used in a much smaller anatomical scale, even intracavitary, with the known efficiency and economics of ultrasound.
Terminology can be at times an issue for some when describing 3D and 4D ultrasound imaging concepts. As the technology is being developed by several different competing manufacturers, often we see quite different terms, or acronyms, used for the same processes or technologies. Industry’s use of marketing catchphrases assigned to technological processes also can be confusing, especially for newcomers. In this chapter, we will describe and explain the most commonly used terms today, and when possible, provide also some of the more common alternatives. In the published literature, the terms “3D ultrasound” and “volume ultrasonography” are commonly used, interchangeably. They both will also be used here, understanding that they represent the same concept. In 3DUS, a volume (a number of slices rather than a single slice) of ultrasound data is acquired and stored. The stored data can be later visualized, reformatted, postprocessed, and analyzed in a number of ways. Uniquely, these volume data can also be reliably quantified, both in 2D and 3D. For the first time, we can reliably measure volumes of any organ system, provided they were included and could fit in the volume acquisition box, and provided that the organ’s borders have decent interfaces. All of the acquired volume data, along with any preprocessing information, can be digitally saved and retrieved at any later time. In addition, small movie clips of these volumes, rotated at different projections to enhance depth perception, can be created and stored as such.
The clinical applications of 3DUS in obstetrics and gynecology have been explored in numerous published works.8-19 Although there has been much more extensive research into the obstetrical applications of 3DUS, there is evidence in the literature that 3DUS does have very substantial applications in gynecology as well.10,20-24.
In daily use, volume ultrasound data represent the acquisition of a number of 2DUS data and an extension of conventional technique. With the introduction of matrix arrays, the thinking process becomes a matter of how thin, or thick, do you want the beam to be, in your region of interest, depending on what you want to see—thin slices for sectional straight cuts, or thick slices for depth perception, fluid filled or bony structures, surface anatomy, displayed in straight or curved planes. As such, volume imaging does not necessarily replace sectional 2D imaging, but rather extends its capabilities. Using 3DUS volume imaging for clinical applications initially may require an additional investment of time (typically 3-5 minutes). With some applications, this time depends on the complexity of the case and experience of the examiner. The additional time is not as much related to scanning and volume acquisition, as to exploring the saved volume data in different scan planes. As a result, although the total examination time may be longer due to the amount of information to be processed, the scanning time, or the time the patient is exposed to the ultrasound beam, with experience should be reduced. During the examination itself, the acquired volume is only briefly scanned to ensure that the region of interest has been adequately included, and there are no artifacts present. If so, the volume is saved in the hard drive of the machine or is sent to an archive server or picture archive and communication system (PACS). Exploration of the saved volumes can be performed after the patient leaves the room or is discharged. A typical sonographic examination workflow using a 3DUS system would involve: (1) scanning the anatomy using conventional real-time 2DUS, mapping the position and orientation of the organs to be visualized relative to the axis of the beam and obtaining standard image planes for volume acquisition; (2) acquiring volumes either slow or fast (depending on whether it is a moving target, or not) in specific acquisition planes known to yield the required planes; (3) volume exploration and inspection for the artifact-free presence of the expected image planes; (4) reformatting, or the quantitative and qualitative analysis of stored volume data such as multiplanar analysis, rendering, calculating volumes, and creating cine loop clips if need be; and (5) storage of volume data in the hard drive (Figure 45-1).
Figure 45-1.
Diagram of the 3DUS workflow, showing the main steps in scanning, starting with real time 2D targeting and followed by 3D/4D ultrasound acquisition and display. The scan is always started in 2D, and the area of interest is mapped out. The suitability of the volume acquisition plane is assessed in real time conventional 2D first. The volume is acquired, and by default displayed, in a user-selected or manufacturer default display mode. A quick exploration of the acquired data will ensure the intended image planes are indeed included in the volume and no artifacts are present. Depending on the clinical need, either a qualitative or quantitative analysis can be done, including at times creation of short video sequence, saved as an AVI loop. If the processed data are deemed informative and useful for comparison in follow-up scans, they are initially saved in the hard drive of the machine and eventually transferred to a longer storage option, such as a central server or large capacity hard drives.

RATIONALE FOR VOLUME SONOGRAPHY
Ultrasound operators often ask why 3DUS should be used in a particular clinical scenario. Experienced 2DUS operators especially have to convince themselves why they would need a 3DUS scan in addition to 2DUS, which has been successfully used for many years. If 3DUS proves to be useful, we all have to put additional amount of effort into learning about and keeping up with the latest developments and purchasing and using 3D equipment in everyday clinical practice.
There are two main reasons why a clinician would need to consider the use of 3DUS (Figure 45-2):
-
Volume ultrasound can obtain image planes that are either impossible or very difficult to obtain.
-
Volume ultrasound can provide depth, which is impossible with 2D ultrasound.
In everyday clinical work, an ultrasound operator is required to obtain the so-called standard ultrasound views and extract clinically useful information based on them. These standard views have been studied in the past for their ability to be acquired by most operators with adequate training. They also have been found to be useful in ruling out major abnormalities. Examples are the biparietal diameter (BPD) plane of the fetal head, the posterior fossa, the atria of the lateral ventricles, the 4-chamber view, and the outflow tracts, umbilical insertion, sagittal, and transverse views of the uterus and endometrial cavity in gynecology, etc. Although these standard image planes can be successfully obtained in the great majority of patients, factors such as fetal position makes their visualization quite difficult if not impossible at times. The patient might have to be brought back for a follow-up scan at a later time because of nonvisualization. The use of volume ultrasound imaging significantly reduces the number of these unsuccessful instances. It also reduces the time it takes to obtain certain anatomical views that might otherwise require quite a bit of scanning and probe manipulation. These views can be generated from an acquired volume even though the probe in 2D mode could not have had direct access to those planes. In addition, volume ultrasound imaging provides access to certain image planes that are not considered standard. Typically, these nonconventional image planes are either difficult (but not impossible) to obtain, such as the midline sagittal view of the fetal brain to visualize the corpus callosum, long axis of the cerebellar vermis, and/or the brainstem (Figure 45-3), or image planes that are simply impossible to obtain. The impossible image planes with 2DUS are typically those that are either perpendicular or close to perpendicular to the ultrasound beam (Figure 45-4). As a result, they are physically impossible and therefore not a requirement in any 2D ultrasound protocol. These impossible image planes can be generated from an ultrasound volume, giving the operator access to an unlimited amount of scan planes at virtually any angle. Of course, some of the main requirements and conditions of ultrasound should be met, such as acquisition plane, resolution, and depth.
Figure 45-3.
Multiplanar view of the posterior fossa in a fetus at 27 weeks’ gestation. The volume was acquired in an axial view with the ultrasound probe placed right above the lambdoid suture, providing the best window to the cerebellum as can be appreciated from (A), the acquisition plane. Note that in (C), in addition to the visualization of cerebellar vermis, tentorium, and cisterna magna, the upper part of the brain stem (white arrows) can also be appreciated in front of the fourth ventricle. In this case, the brain stem is visualized in a view that is 90 degrees from the ultrasound beam—something possible if acquired from 24 to 28 weeks’ gestation. Nevertheless, the brain stem is not always visualized by ultrasound, but it is usually easier to see from 22 to 28 weeks’ gestation if the volume is acquired ideally in an occipital sagittal orientation of the probe. In this case, the volume is acquired in an axial plane through the lambdoid suture.

Figure 45-4.
Graphic display demonstrating the possible and impossible image planes by conventional 2D and 3D ultrasound. A conventional 2D transducer can only visualize the transverse and sagittal views of this fetal spine, as these are the only views in the axis of the ultrasound beam. A volume probe, even though it scans in the same direction as a 2D transducer, acquires a volume of 2D data. From this volume, image planes that are physically impossible with a 2D transducer can be obtained, such as planes perpendicular (or close to perpendicular) to the ultrasound beam (ie, the coronal plane).

These planes can be very important clinically, as they might be the best views to rule out some difficult conditions. Examples of impossible planes with clinical importance are the coronal plane of the uterus and endometrium (Figure 45-5), coronal plane of the cervical canal, coronal planes of the rectum (Figure 45-6), or the coronal plane of the perineum with short-axis views of the urethra, vaginal canal, and rectum, as well as the long axis of the puborectalis portion of the levator plate seen in one single view, to name just a few (Figure 45-7).
Figure 45-5.
An illustration of the different scanning planes used to image the uterus in a volume. A-C: Different coronal planes through the uterus. D: Transverse planes. The image plane in (A) shows the coronal plane (in green) of the uterine fundus in an anteverted uterus, but clearly this same plane does not display the coronal plane of the lower uterine segment/cervix. The image plane in (B) shows the coronal plane of the lower uterine segment/cervix, but it does not display the coronal plane of the uterine fundus. In (C), the scanning plane has been curved up (bent upwards), accounting for the normal anteversion of the uterus so it can display at the same time the coronal plane of both the lower uterine segment/cervix and the uterine fundus. This is an important step in evaluating the uterus in a multiplanar display in the coronal plane. In (D), transverse planes of the uterus are displayed separately for the lower uterine segment and the uterine fundus, again accounting for the fact that they are not necessarily in the same plane.

Figure 45-6.
Composite image of a multiplanar view of the rectum (A–C) and two different transverse scan planes (D and E) in relation to the coronal image (F) of the rectum. This volume was acquired with a transvaginal volume probe. The probe was angulated upward right at the entrance of the vaginal canal in the perineum to be perpendicular to the long axis of the rectum. This approach puts less pressure in the rectum, reducing the amount of distortion as well as having better acceptance by patients compared to the endoanal approach. Sagittal view (A) displays a long axis of the internal and external sphincter (the whole length) ruling out any area with evidence of rupture, its location, and its extent, which cannot always be done with standard clinical examination. Window (B) is a transverse view, and window (C) is a coronal view displayed in a multiplanar display. Windows (D) and (E) are transverse rendered views at two different points of the rectum. Intrapartum damage to the internal and external sphincters can usually be seen at 12 o’clock in transverse views.

Figure 45-7.
Composite image of two different displays of the same volume of the perineum acquired with a transvaginal volume probe placed in the introitus. Window (A) is a sagittal view where several important landmarks are commonly visualized with 2DUS. Window (B) is a transverse view of the same area, and (C) is a coronal view of the perineum. Window (C) is impossible to acquire with 2D ultrasound as it is perpendicular to the ultrasound beam. Window (D) is a “niche” mode display of the perineum where all three image planes are shown in intersection. Windows (E) through (H) show the same anatomic region in a different patient acquired with the same transvaginal probe, but displayed with the tomographic ultrasound imaging display. In the reference window (E), three coronal slices are spread out to evaluate the perineum at depth. This display is quite useful in evaluating the integrity of the puborectalis muscle and rule out an avulsion. Windows (I) and (J) display the same volume in a rendered coronal view. This is a unique view where many important anatomical regions are displayed. This view is commonly acquired with MRI, but 3DUS can acquire the same landmarks with depth and even perform real-time dynamic studies (ie, under Valsalva). The main landmarks have been highlighted in color and numbered to better delineate them: (1) symphysis pubis, (2) urethra, (3) vagina, (4) distal part of rectovaginal septum, (5) pubovisceral muscle, and (6) rectum. The image on the right has a green marker highlighting the area of hiatal circumference that can be evaluated during rest, contraction, or Valsalva to evaluate strain, a component of pubovisceral muscle elasticity.

The second major reason to use volume sonography is that it can provide depth, which is also impossible to obtain with 2D ultrasound. The reason is simple: with 3DUS a volume of 2D ultrasound data is acquired. As a result, the beam is “thickened,” and the anatomy does not have to be viewed only by cross sections via a “thin” 2D beam (Figures 45-7I, J, and 45-8). The anatomy can be analyzed in user-selectable transparent and nontransparent (surface) modes through this “thick” beam. Depth is provided for both the possible conventional planes (ie, fetal profile or frontal face view; Figures 45-9, 45-10, 45-11), and for the impossible ultrasound views previously mentioned (Figures 45-12 and 45-13). In addition, there are some impossible image views that can only be adequately visualized using depth, as their shape is not straight and visualization of different depth levels is needed. Coronal views of the perineum, fetal secondary palate, and optic chiasm are typical examples (see Figures 45-7I, and J, 45-12, and 45-13).
Figure 45-8.
This figure presents a dual view of a conventional 2D image and VCI-A volume contrast imaging in plane (A) still TIFF image generated from a 4D video sequence. This figure shows a fetal bladder with an ureterocele in a fetus at 23 weeks’ gestation that was subsequently found to have a duplication of the collecting system in the left kidney. A: Two-dimensional ultrasound image of the fetal bladder where the ureterocele walls and shape are not well depicted. B: VCI-A image composed of 2-mm thickness. The volume-derived VCI-A view by thickening the beam displays critical clinical information not adequately seen in this case with conventional 2DUS that prompted the operator to look further and find a duplication of the renal collecting system. Note the significantly improved contrast resolution along the ureterocele wall and fluid interfaces in (B) not present in (A) representing the 2D image on the left. C and D: VCI-C (volume contrast image in plane C) of the cervix at 22 weeks’ gestation. D: Coronal plane of the cervix showing the cervical canal. This plane is inaccessible with conventional 2D imaging. Since this is a 4D sequence, the operator can scan with this mode to find the perfect midsagittal plane of the cervix using VCI-C as a reference, and then, as desired, the beam can be reverted back to the 2D mode to record the cervical length. This same concept can be applied to scanning any midsagittal plane.

Figure 45-9.
Surface rendering of fetal face profiles in a variety of conditions. A: Anencephalic fetus at 31 weeks’ gestation. B: Maxillary protrusion in a bilateral cleft lip and palate fetus at 25 weeks’ gestation. C: Pierre Robin fetus with micrognathia (and cleft palate not seen in this view) at 22 weeks’ gestation. D: Semilobar holoprosencephaly fetus with an abnormal coronal suture at 23 weeks’ gestation. E: Alobar holoprosencephaly fetus with a proboscis and cyclops eye (not seen in this profile view) at 24 weeks’ gestation. F: Thanatoforic dysplasia fetus at 27 weeks with frontal bossing and micrognathia. Note the huge amount of clinical information gathered from soft tissue via surface rendering.

Figure 45-10.
Composite image of four different fetuses shown in frontal rendered views of the face. A: Normal face at 27 weeks’ gestation rendered with 100% surface rendering showing details of normal morphology. This particular view provides a great amount of information regarding the mid-face, to rule out hypoplasia, lips, height of the filtrum, width of the nose, and eyes (up or down slanting, intercanthal distance versus the interorbital view we normally get with 2D ultrasound). B: Fetal face at 22 weeks’ gestation rendered with 65% surface and 35% maximum intensity projection to display the fetal metopic, sagittal, and coronal sutures. Typically, both of these rendering algorithms are combined to achieve visualization of the desired features. C: Unilateral cleft lip fetal face rendered with 100% surface rendering at 25 weeks’ gestation. The umbilical cord was superimposed in front of the face and was edited out with the electronic scalpel feature. D: Alobar holoprosencephaly fetus with a proboscis and cyclops eye (both eyes are fused in the middle) right underneath it at 24 weeks’ gestation.

Figure 45-11.
Composite image of three fetuses displayed with surface-rendering algorithms. A: Gastroschisis at 22 weeks’ gestation. B: Omphalocele with an umbilical cord cyst at 22 weeks’ gestation. Both images are generated from a slow 3D ultrasound volume with harmonics on, only one focal zone, and rendered with surface rendering 100% with slight adjustments in the lower threshold for eliminating low-intensity echoes. Volumes were acquired in such a way that a pocket of fluid was placed between the beam and the area of interest, in these cases bowel and liver. The gamma curve was adjusted afterwards to improve contrast. Surface-rendered views are informative as long as there are good tissue interfaces such as soft tissue versus fluid, as in these cases. The value here is identifying the content of these masses outside the abdomen, as well as help to identify the umbilical cord insertion. C: Surface-rendered view generated from a 4D volume acquisition sequence of a fetus at 25 weeks’ gestation with bilateral pleural effusion drained via two catheters placed a week prior. The catheters (green arrows) were identified bilaterally. Volume displays were not used for catheter placement guidance, but they were used for the follow-up scan targeting the chest area looking for their presence.

Figure 45-12.
Multiplanar and rendered view of the fetal optic chiasma acquired close to a midsagittal plane at the level of the corpus callosum with a slow 3D acquisition in a fetus at 26 weeks’ gestation. This plane is impossible to acquire with 2D ultrasound as it needs depth along a curved line. The volume box is placed below the septa pellucida as shown in (A) and (B), and is made very thin. The rendering line is curved down to follow the anatomic orientation of this structure. The rendering algorithm used is surface 70% and gradient light 30%. The optic chiasma appears hyperechoic. This is an important view in septal agenesis to evaluate the presence and integrity of the optic tract traditionally obtained with MRI.

Figure 45-13.
Multiplanar and rendered view of a normal primary and secondary fetal palate in a fetus at 26 weeks’ gestation. A 3D volume with slow settings, harmonics at midlevel, and compound resolution imaging (CRI) (1 level) was acquired in a transverse plane by angling first in the sagittal plane posterior to the palate. Note in (B) that the rendering box is kept thin and is curved to adjust to the normal curvature of the primary and secondary palate. The rendering settings were surface 100%.

In everyday practice, either one or both of these reasons (impossible planes and/or depth) are used to gain access to certain anatomic planes that are deemed clinically useful. As a rule, we use the mutiplanar views for cross-sectional analysis, using our knowledge gained over the years with 2DUS, and correlate that with the many choices offered by the rendered view, which offers depth. We do this analysis sometimes in the same display, and at other times in individual displays (Figures 45-14, 45-15, 45-16).
Figure 45-14.
Composite image of two different fetuses with bilateral cleft lip and bilateral primary and secondary cleft palate, both at 25 weeks’ gestation. The upper row shows the long axis of the fetal palate as seen in an axial plane of the head generated from a multiplanar display. The yellow arrows point to the premaxillary protrusion. The red arrows point to the secondary palate defects bilaterally. The green lines represent the axial scanning plane to obtain visualization of the long axis of the fetal palate. This type of analysis is typically done with the multiplanar and rendered view simultaneously displayed. A green line can be placed in the rendered view to indicate the scan plane in the axial window in the multiplanar display.

Figure 45-15.
Composite image of conjoined twinning in a monoamniotic monochorionic pregnancy at 10 weeks’ gestation acquired with a slow 3D volume with a transvaginal probe. The uterine wall around the fetuses is removed with electronic scalpel. A multiplanar and rendered display is used to evaluate these fetuses and rule out conjoined twinning. In (A), the scan plane (green line) is placed at the level of the abdomen. At this level the fetuses are separate from each other, as seen from the axial view in the multiplanar view. In (B), at the level of the chest, again both fetuses are separate as seen in the axial view. In (C), the heads appear to be adjacent to each other, but in (D), there is evidence of brain sharing as seen in the axial view. This is a good illustration of the ability of volume ultrasound to obtain image planes that could not be reached with conventional 2D ultrasound as the axial planes for both fetuses were not accessible at every desired level due to fetal position.

Figure 45-16.
Multiplanar and rendered view of a monoamniotic monochorionic twin pregnancy at 15 weeks’ gestation. In conventional 2D ultrasound, the cords could not be followed all the way to their placental insertion site. A 4D ultrasound sequence was initialized, and after a good acquisition plane was identified this way, a single slow 3D volume was acquired and analyzed. Note in the rendered display that the umbilical cords are entangled several times. This case is a good illustration of the main volume imaging capabilities that prove to be clinically very useful: providing access to a plane with depth. Both features are not possible to be obtained with 2D. The rendered plane is perpendicular to the ultrasound probe. The image generated is a product of several 2D slices in a volume providing depth perception, which was key to the diagnosis. The red arrows in sagittal, transverse, and coronal views demonstrate the several different depth levels that are displayed in one single view in the rendered display.

VOLUME ACQUISITION
Volume acquisition is arguably the most important part of the whole process of using 3DUS clinically.
Ultrasound volume acquisition methods are divided into three main categories:
-
The initial, and the oldest, way is the manual or “free-hand” acquisition method, which acquires volumes using a conventional 2DUS probe. In some of the better configurations, a position sensor is mounted externally to the probe assembly to provide position-sensing data to accurately register the acquired 2DUS data in space and time. The free-hand technique can be used in both inline (where image acquisition, transfer, storage, and manipulation is done in the same unit) and offline systems. With offline systems, volume data are commonly captured using the ultrasound machine’s video-out port and downloaded to an offline computer workstation equipped with a video frame grabber PCI card. Volume imaging software is used for subsequent 3DUS image reconstruction and analysis. Although the free-hand technique can be economically implemented into existing systems as it uses conventional 2DUS probes, its main drawback is that it cannot be used for accurate measurements, and its volumes are likely to suffer from acquisition-related artifacts.7,25 With offline systems, the external probe-holding mechanisms are typically bulky, and the image transfer process to an off-line workstation is labor intensive and time-consuming (Table 45-1). The free-hand acquisition techniques are traditionally used to image parts of the anatomy that do not move (the nonpregnant uterus and adnexa) and generally are avoided when imaging moving targets such as the fetus (Figures 45-17 and 45-18).
-
Mechanically swept 3DUS probes. These probes (ie, probes with a mechanized drive contained within the probe case itself) are commonly referred to as dedicated volume probes. When these probes are activated, the transducer elements are swept by a motor through the region of interest within the limits of a volume box, with an operator-selected speed and angle, while the probe is held stationary. The most common design of these probes has an electronic array achieving imaging in one direction (the 2D beam) that is almost simultaneously swept mechanically in the orthogonal direction (volume acquisition in the Z plane). So, two orthogonal planes are acquired: one is achieved electronically, and the other mechanically by sweeping the beam. The main advantage of this method is that images obtained with the automated approach permit accurate 2D and volume measurements and demonstrate more precise spatial relationships. The dedicated volume acquisition methods are considered more reliable, especially in imaging moving targets. As the operator’s hand stands stationary and the transducer elements move through a motor, the volumes can be acquired and displayed fast, at over 60 times per second, allowing the depiction of moving targets as they change position (fetus, fetal heart). This fast acquisition and display method is commonly called four-dimensional ultrasound (4DUS) with the element of time considered the temporal or the fourth dimension (see Figure 45-17). One major drawback of this method is that it does not focus in the elevational plane, or the plane along the depth or volume acquisition axis (see Table 45-1). While the volumes can be also acquired with color or power Doppler, as well as with B-flow, these acquisitions cannot be obtained fast and in continuity in a 4D sequence. They can only be obtained as one single volume, and the volume acquisition time is typically longer compared with grayscale-only volumes.
-
Two-dimensional matrix arrays. These transducers represent the future of volume acquisition. They are composed of a transducer mosaic arranged in rows and columns with a very high number of 2D piezoelectric elements (8000 elements and even more projected in the near future). These probes scan in both orthogonal planes electronically and can acquire a pyramidal-shaped volume.14,26-28 Because these transducers allow volume acquisitions in all planes, they allow focusing not only in one plane, but in the elevational plane as well, significantly increasing the quality of the acquired volumes. In addition, these transducers provide very fast frame rates without significant deterioration in image quality because all the transducer elements are fired at the same time (or in sequential groups). To increase the area of interest, they may employ 3D beam steering (see Table 45-1). The information is typically displayed in a biplanar display or typical orthogonal multiplanar displays, as well as in rendered displays. Due to the huge amount of data these transducers collect, the hardware and software needed to process this information is still in its early days, and in constant evolution (see Figures 45-17 and 45-18). One workaround for this problem is the reduction in the number of available channels, otherwise called sparse arrays, which results in inferior image quality, but the data can be processed faster.
Acquisition Method | Advantages | Disadvantages |
---|---|---|
Freehand, no position sensor (online or offline) | Economical way to acquire volumes. Can be easily implemented into existing systems via video-out port. Qualitative analysis possible. | Very susceptible to registration errors and acquisition motion artifacts. Volume data cannot be used for measurements. |
Free-hand with position sensor and/or probe holders (online or offline) | Can be externally attached to the existing 2D systems. Much improved image registration. Portable and available to more than one piece of equipment. Both quantitative and qualitative analyses possible. |
Motion artifacts are still a major issue when scanning moving targets. Articulated arms or probe holders are cumbersome to use. |
Dedicated mechanically swept probes | Quite reliable volume acquisition and registration. Optimized equipment. No need for manual movements of the probe. Able to achieve very fast acquisition speeds to capture moving targets. | Do not focus in the elevational plane. Slightly larger footprint, heavier, and more costly than conventional 2D probes. |
2D matrix array | High number of information channels possible. It can focus in the elevational plane. Fast frame rates. Very small footprint probes. Reasonable image spatial resolution. Biplanar real-time imaging of 2 orthogonal planes without resolution loss. | Quite narrow apertures generating small volumes. Not yet on par with the resolution of 2D probes. Signal-to-noise ratio and dynamic range not comparable with 2D systems. Cost issues at the moment. Very limited probe configurations mainly in lower frequencies. |
Figure 45-17.
Graphic illustration of different volume ultrasound probes, volume acquisitions, and beam characteristics. A: Free-hand acquisition where the probe is physically moved over the area of interest. B: Volume acquisition with a dedicated mechanical sweep. The transducer is maintained stationary, but the transducer elements are mechanically swept in an operator-selected angle of acquisition and speed. C: Graphic illustration of beam shape in a 2D matrix array probe demonstrating the full volume as acquired. Note that the probe is acquiring volumes electronically in both orthogonal directions that make focusing in the elevational plane possible. D: Shows the ability of the 2D matrix array to focus at any direction in 360 degrees. E: Graphic illustration of the scanning ability of 2D matrix arrays to obtain a biplanar image fully focused and rotated it real time. F: Shows lateral tilting ability of this probe. These features are unique to 2D matrix arrays. (Used with permission from Amy M. Lex MS, RT(R), RDMS, Philips Ultrasound, Bothell, WA, USA.)

Figure 45-18.
This is a composite image of various 3D/4D ultrasound instruments. A: Traditional offline system setup where the main 2DUS machine (2) connects via its video-out port to a computer workstation (1) equipped with a video frame grabber card. Volumes are acquired manually (free-hand method) with the 2DUS machine via its 2D transducer, and postprocessed and displayed at the offline workstation (1). A position-sensing device, seen on the examination table, is externally attached to the 2D transducer to improve the registration process. B: Dedicated volume ultrasound machine (3) where the volumes are acquired automatically with a mechanically swept transducer and later processed and displayed in the same unit. C: Several 2D and volume probes: (4) is a 2D matrix array probe; (5) is a conventional 2D probe. Note the small footprint of the matrix array transducer even though it packs a large amount of transducer elements; (6) is a transvaginal volume probe; and (7) a conventional 2D transvaginal probe. Note that both probes are of roughly the same size even though the volume transvaginal probe is housing a motor to sweep the transducer elements. D: (from left to right) Volume probes for transvaginal (8), transfontanelar for neonatal head imaging (9), transabdominal (10), and breast imaging (11).

The 2D matrix arrays can acquire the following: (1) a single 3D volume, which is typically acquired very fast, in roughly 1 to 2 seconds; but again, the speed of acquisition depends on volume size; (2) a 4D volume sequence, where the volumes are acquired one after another, but they are not that fast because all of the elements are sequentially fired at full aperture, so they do not yet acquire in real time; these volumes can also be acquired with color or power Doppler and this is one of the strongest points for this mode; (3) fast 4D real-time imaging of up to 24 volumes per second; the trade-off here is that the transducer only uses roughly one-third of the aperture; and (4) real-time biplanar imaging where two orthogonal images are acquired and displayed in high-resolution grayscale as well as with color or power Doppler.28 The biplanar image is fully focused and can rotate as well as tilt laterally in real time (Figures 45-19, 45-20, 45-21). This particular mode is extremely helpful and has a central role in the use of 2D matrix arrays.
Figure 45-19.
Biplane mode enables the probe to steer the scan planes in perpendicular orientations allowing for acquisition and real-time display of two orthogonal planes. The operator is able to quickly review the fetal palate from the profile view as he is scanning in real time. (Used with permission from Dr. Peter Falkensammer, GE Healthcare.)

Figure 45-20.
Active cooling is an innovative solution to dissipate heat from the transducer electronics and enables the Voluson eM6C probe to achieve greater penetration, frame rates, and image quality compared to passive cooling techniques. (Used with permission from Dr. Peter Falkensammer, GE Healthcare.)

Figure 45-21.
Two stage beam formation. Voluson e4D is a synergy of probe and system design that utilizes two stage beamformation to extract the maximum amount of information from the eM6C probe. The first stage of beamformation occurs within the probe handle, which itself is equivalent to a mini ultrasound system that translates the information from the less than 8000 elements in the transducer to the system. The Voluson E10 with the Radiance System Architecture provides the second stage of beamformation. During the second stage, the system rapidly processes information while applying advanced image processing algorithms to create the final image. (Used with permission from Dr. Peter Falkensammer, GE Healthcare.)

In order to get a better appreciation and understanding of electronic 4D imaging, it would be informative to briefly present the evolution of matrix array technology. For many years, the main ultrasound manufacturers have used matrix array technology to enhance image quality. The first matrix probes were primarily developed for 2D imaging. Matrix arrays introduce multiple element rows, which allow focusing in the near, mid, and far field—providing a uniformly thin beam throughout the field of view. With standard volume probes, the transducer is designed with a single row of elements aligned parallel to one another across the array. This standard array design has a fixed elevation focus, with the resulting slice thickness to increase in both near and far field, as you move away from the focus area. Increased slice thickness, outside of the focus area, results in volume averaging and decreased contrast resolution in near and far field. To create a volume, mechanical 3D probes utilize a mechanical motor to sweep the beam throughout the field of view. A series of 2D images are acquired and translated into a volume.
Mechanical matrix probes utilize a more sophisticated transducer array compared to standard volume probes. With mechanical matrix volume probes, elements are diced in two directions for improved elevation plane focusing. The end result is a uniform slice thickness from near field to far field with less volume averaging. The resultant image has improved image quality and uniformity compared to a standard transducer array. To create a volume, the array is once again swept through the field of view by a mechanical motor, and 2D images are acquired and translated into a volume. While the addition of multiple element rows provides significant improvements in image quality, we still experience the same limitations associated with mechanical probes—volume rates and orthogonal plane resolution.
With electronic 4D probes, a matrix array contains a multitude of element rows and thousands of elements. With more than 8000 elements, they provide so much control that the ultrasound beam can be actively focused in any direction, creating a narrow symmetric beam, which leads to excellent resolution in all imaging planes. This ability to actively steer and focus the ultrasound beam in any direction, in any sequence, provides an incomparable level of control, efficiency, and image quality. These features provide greater flexibility in imaging planes to enable advanced functionality such as real time biplane imaging, or real time VCI-A (Figures 45-22, 45-23, 45-24).
Figure 45-22.
Volume contrast imaging (VCI). The graphic depicts how multiple planes acquired in a thin volume are combined to create a thick slice 2D image with excellent contrast resolution. VCI imaging delivers excellent contrast resolution by combining multiple images from a thin volume acquisition. The ultra fast volume rates of Voluson e4D allow VCI-A to be used in real time as a standard mode to help acquire images with improved contrast of the fetal brain, extremities, heart in technically difficult patients. (Used with permission from Dr. Peter Falkensammer, GE Healthcare.)

Figure 45-24.
VCI-A of the fetal arm showing out of plane structures in a single plane for a quick and detailed anatomical review. Having multiple planes acquired gives VCI-A the ability to show anatomical structures that are otherwise difficult to be seen in a single 2D scan plane. (Used with permission from Dr. Peter Falkensammer, GE Healthcare.)

Heat is an inherent side effect of generating ultrasound energy, whether it is from the advanced electronics used to control the thousands of elements in the probe or from the excitation of the piezoelectric material that generates the ultrasound beam. To maximize performance, General Electric’s (GE Healthcare) eM6C probe incorporates active cooling, an innovative solution to dissipate heat from the transducer. Active cooling utilizes a thermally conductive liquid that is cycled through the probe handle and probe connector. Warm fluid travels from the probe handle to the connector where it is cooled and cycled back up to the probe (see Figure 45-20). Actively cooling the transducer allows the eM6C to realize its full potential and achieve greater penetration, frame rates, and image quality compared to passive cooling techniques.
With standard probes, the control and timing of the individual elements for transmit and receive beam-formation lies within the ultrasound system. The transducer cable links each individual element to the ultrasound system. While this is a proven and robust architecture, it is not feasible with electronic 4D probes that contain thousands of elements. GE Healthcare’s Voluson e4D is a synergy of probe and system design that utilizes a two stage beam-formation (see Figure 45-21) to extract the maximum amount of information from the eM6C probe. The first stage of beam-formation occurs within the probe handle, which itself is equivalent to a mini ultrasound system that translates the information from the less than 8000 elements in the transducer to the system. The sophisticated electronics within the probe provide the beam agility to steer and focus the ultrasound beam in any direction and any sequence. This beam agility is the key to unlocking new modes in which multiple planes are imaged simultaneously. The Voluson E10 provides the second stage of beam-formation. During the second stage, the system rapidly processes information while applying advanced image processing algorithms to create the final image.
Before a volume is acquired, certain important principles should be considered. Considering that the most widely available and used probes currently in the market are the mechanically swept ones, these guidelines deal mainly with issues pertaining them, even though many of these principles apply to matrix arrays as well.
Prior to volume acquisition, optimize the 2D image to maximize the quality of the volume by adjusting depth (improving the refresh rate and making the acquisition faster), aperture (or beam angle, improving lateral resolution), number of focal zones (one is usually enough), and harmonics. If imaging the fetal heart, lower or even completely remove the persistence value, as frame averaging does not adequately display the valve motion. Pay close attention to the refresh rate value displayed in the screen, as a lot of the preprocessing options such as compound resolution imaging, speckle reduction, or coded excitation may negatively influence it (Figure 45-25).
Acquisition of volumes can be accomplished via both transabdominal and transvaginal transducers. In gynecology and first-trimester acquisitions, although the quality of the transabdominal acquisitions and rendering is adequate, transvaginally the quality of the volumes is generally very high. Otherwise, second- and third-trimester acquisitions are performed transabdominally. The examination is started in conventional 2D mode to target the region of interest (ie, the fetus), preferably in a long-axis view. The aperture is kept at the narrowest possible but still including the longest axis of the fetus. Acquisition is started with the lowest speed that translates into the highest quality setting. As a rule, the faster the acquisition speed, the lower the number of 2D slices acquired in the volume. Therefore, the multiplanar analysis in the planes other than the acquisition plane is likely to display low-resolution quality. If the fetus is moving, a fast 4D acquisition is needed to keep up with the fetal motion and avoid artifacts. The slower the acquisition speed, the greater the number of 2D slices in the acquired volume. Consequently, slower acquisition speeds yield higher resolution volumes. On the downside, lower speeds typically are more likely to suffer from motion artifacts (especially when imaging a moving target) (Figure 45-26). Registration artifacts are more prevalent in the views other than the acquisition plane.
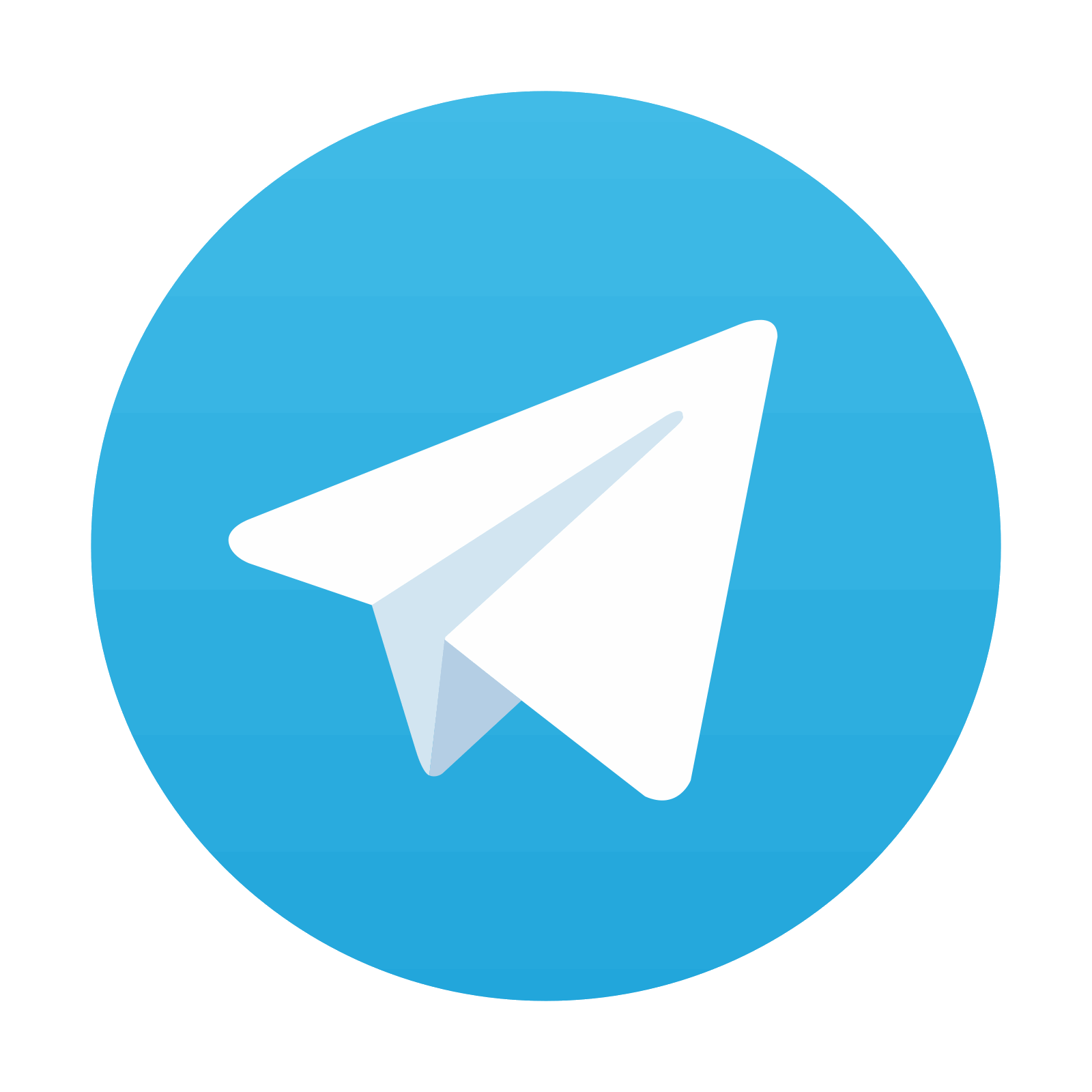
Stay updated, free articles. Join our Telegram channel
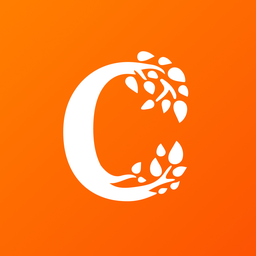
Full access? Get Clinical Tree
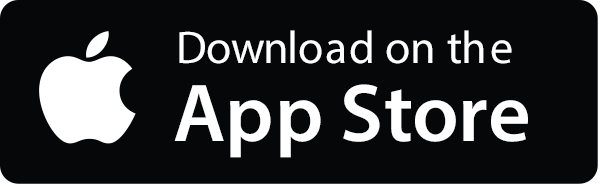
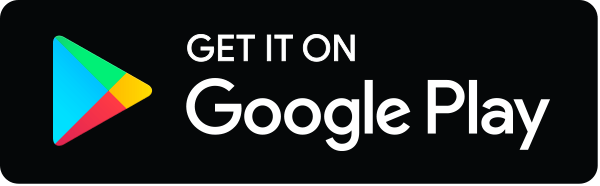