Fig. 31.1
The chemical structure of the potent inhalational anesthetic agents includes both an alkane (halothane) and ethers. Of the four ethers, isoflurane, enflurane, and desflurane are substituted methyl-ethyl ethers and sevoflurane is a methyl-isopropyl ether
Table 31.1
Physical properties of the inhalational anesthetic agents
Inhalational anesthetic agent | Vapor pressure, mmHg at 20 °C | Blood-gas partition coefficient | Minimum alveolar concentration (%) |
---|---|---|---|
Halothane | 243 | 2.54 | 0.76 |
Enflurane | 175 | 1.91 | 1.7 |
Isoflurane | 238 | 1.46 | 1.2 |
Sevoflurane | 160 | 0.69 | 2 |
Desflurane | 664 | 0.42 | 6 |
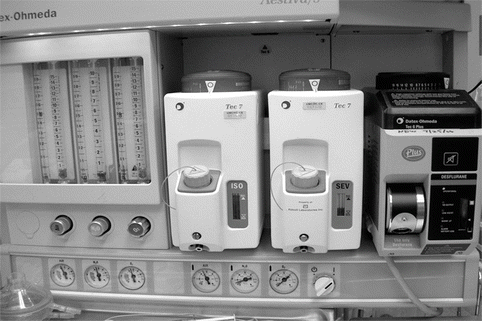
Fig. 31.2
Standard, agent-specific vaporizers on an anesthesia machine
31.2.2 Uptake and Distribution
A unique aspect of the inhalational anesthetic agents which offers various advantages and disadvantages in the pediatric ICU setting is their administration via the respiratory tract. The onset of effect and duration of action of an inhalational anesthetic agent are determined by one its physical properties known as the blood-gas solubility coefficient. This coefficient describes how the anesthetic agent partitions itself between the blood and the alveolar gas when equilibrium is reached. In effect, this describes its solubility in the blood. The lower solubility in blood allows the alveolar concentration of the agent and hence the brain concentration of the agent to increase more rapidly than agents with a higher solubility in blood. As the goal is to achieve a specific partial pressure of the inhalation anesthetic in the brain to create the desired effect, an agent with a high blood-gas solubility (partition) coefficient has a slower onset of action than an agent with a low blood-gas solubility coefficient. Likewise, the higher blood-gas solubility also results in a longer duration of action when administration of the agent is discontinued. Although this difference is most notable during the induction of anesthesia when agents with a low blood-gas solubility coefficient have a more rapid onset, the depth of anesthesia can also be adjusted more quickly with an agent that has a lower blood-gas solubility coefficient. Desflurane has the lowest blood-gas solubility coefficient and therefore the most rapid onset and offset of activity, followed in order by sevoflurane, isoflurane, enflurane, and halothane (Table 31.1).
Although the inhalational anesthetic agents are administered via the respiratory system, their delivery and distribution to the body tissues are governed by many of the same principles as medications administered via the intravenous route. The increase in the alveolar concentration of the agent is determined by the minute ventilation and the inspired concentration of the agent. The inhalational anesthetic agents are then taken up from the alveoli into the blood. Their uptake is dependent on their solubility in the blood (blood-gas partition coefficient), blood flow through the lungs (cardiac output), distribution of blood flow to the various tissue beds, and their solubility in these tissues (blood-tissue solubility coefficient). The end-capillary venous blood from the lungs which empties into the left atrium and eventually becomes the arterial blood leaving the left ventricle rapidly equilibrates with the alveolar concentration (Eger and Bahlman 1971). In turn, the arterial concentration of the inhalational anesthetic agent equilibrates with the brain tissue to provide the anesthetic effect. Given these principles, the alveolar concentration of the agent parallels the brain tissue concentration. This occurs given the high blood flow to the brain compared to other organs. These principles describe why agents such as desflurane and sevoflurane that are relatively insoluble in blood and have a low blood-gas solubility coefficient result in a more rapid rise in the alveolar concentration and therefore the most rapid onset of action. Although these effects may be of limited importance when these agents are used in the PICU setting, they govern the onset of action during the induction of anesthesia in the operating room setting.
The increase in the alveolar concentration depends on the difference between the delivery of the agent to and its removal from the alveolus. Delivery is controlled by the inspired concentration of the agent and the patient’s minute ventilation. The removal of these agents from the alveoli is dependent on the solubility of the agent in the blood (blood-gas partition coefficient), the pulmonary blood flow, and the concentration of the agent in the mixed venous blood. Therefore, the rise in the alveolar concentration of the inhalational agent may be altered by the presence of congenital heart disease and right-to-left or left-to-right shunts. In a patient with a left-to-right shunt, blood with a high concentration of the inhalational anesthetic agent returns from the lung and enters the left atrium. This blood then recirculates through the lungs through the left-to-right shunt, thereby increasing the mixed venous oxygen tension of the inhalational anesthetic agent more rapidly than the normal. This accelerates the rise in the alveolar concentration and thereby the onset of action of the agent. In a patient with a right-to-left shunt, the opposite effect occurs with a delayed onset of action of these agents.
31.2.3 Potency (Minimum Alveolar Concentration or MAC)
The potency of an inhalational anesthetic agent is measured by using the principle of MAC (minimum alveolar concentration). MAC is defined as the end-tidal concentration (percentage) of the inhalational anesthetic agent in 1 atm that prevents 50 % of patients from moving in response to a surgical stimulus. It mirrors brain partial pressure and therefore allows for comparison between these agents. The most potent of the agents will have the lowest MAC value as less is required to produce a given clinical effect (Table 31.1). Halothane is the most potent of the inhalational anesthetic agents with a MAC of approximately 0.76 % followed in order by isoflurane (1.2 %), enflurane (1.7 %), sevoflurane (2 %), and desflurane (6 %). Both patient-related factors and the concomitant administration of other medications can affect MAC. Medications which lower the MAC of the inhalational anesthetic agents include opioids, α2-adrenergic agonists, and benzodiazepines. Other factors that may affect MAC include age, pregnancy, and central nervous system disorders. MAC is lower in preterm infants, increases in term infants, and then decreases slightly with advancing age (Le Dez and Lerman 1987; Gregory et al. 1969). As it would be undesirable to have 50 % of patients moving during surgical procedures, 1.5–2.0 MAC of an inhalational anesthetic agent may be administered intraoperatively during anesthetic care. Alternatively, a lower concentration agent of the agent (1.0–1.5 MAC) may be combined with other agents such as nitrous oxide (N2O), opioids, or intravenous anesthetic agents to provide maintenance anesthesia. Another term, MAC awake describes the partial pressure (end-tidal concentration) at which most patients will open their eyes and awake from the general anesthetic. This is generally 0.3–0.4 MAC. In the pediatric ICU setting, when these agents are used for various therapeutic purposes, the concentration required to achieve the desired effect will vary considerably depending on the clinical scenario, the indication for its use, the patient’s status, and the duration of administration.
31.3 End-Organ Effects
As with any medication used in the pediatric ICU setting, the inhalational anesthetic agents may have deleterious effects on physiologic functions. Unlike their earlier predecessors, all of the inhalational anesthetic agents in current clinical use are nonflammable and nonexplosive. They provide the prerequisites of a general anesthetic including amnesia, analgesia, skeletal muscle relaxation, and to some extent control of the sympathetic nervous system. Although the inhalational anesthetic agents share these effects on the central nervous system, in other regards, given the variances in their chemical structure, their adverse effects on end-organs may be quite different.
31.3.1 Central Nervous System
Recent work suggests that the inhalational anesthetic agents stabilize critical proteins, possibly receptors of neurotransmitters particularly those that have an inhibitory effect in the CNS including the γ-amino butyric acid (GABA) system, the same system involved in the CNS effects of benzodiazepines, barbiturates, and propofol (Eckenhoff et al. 2005). Given their depressant effects on the CNS, the inhalational anesthetic agents cause a dose-related decrease in CNS activity, reduce the cerebral metabolic for oxygen, and depress electroencephalographic (EEG) activity. In contrast to their usual depressant effects on the EEG pattern, in specific circumstances, both enflurane and sevoflurane can activate the EEG and produce EEG evidence of epileptiform activity (Eckenhoff 1998). Although these EEG changes are rarely of clinical significance, they are also occasionally accompanied by motor manifestations suggestive of seizure activity such as rhythmic movements of the extremities or tonic posturing. This EEG activation generally occurs during the rapid increase in the alveolar concentration of the agent or the administration of high inhaled concentration such as the inhalational induction of anesthesia when inspired concentration of 6–8 % sevoflurane is commonly used. In this situation, EEG activation is enhanced by hyperventilation and the development of hypocarbia. Clinical practice also suggests that such activation may be more common in children with abnormal neurologic function or underlying disorders of the CNS. Despite this property, as outlined below, all of the inhalational anesthetic agents including sevoflurane depress EEG activity and have been used in the treatment of status epilepticus.
Although the inhalational anesthetic agents decrease the cerebral metabolic rate for oxygen, they increase cerebral blood flow (CBF) in a dose-dependent manner via a reduction in cerebral vascular resistance. This cerebral vasodilatation may elevate intracranial pressure (ICP) in patients with compromised intracranial compliance. Additionally, the hemodynamic effects may lower mean arterial pressure (MAP) which coupled with the increase in ICP may further decrease cerebral perfusion pressure (CPP) (Sponheim et al. 2003). The adverse effects on ICP are least with isoflurane, minimized by limiting the concentration to 1.0 MAC, and are blunted by hyperventilation leading to hypocarbia (PaCO2 of 25–30 mmHg) (Adams et al. 1981; Drummond et al. 1986). However, given these effects both directly on ICP and indirectly on CPP via the effects on MAP, these agents should be used cautiously in patients at risk for alterations in ICP or those who may not tolerate decreases in CPP. In addition to these effects on the CNS, the potent inhalational anesthetic agents depress neuromuscular activity and enhance the effect of non-depolarizing neuromuscular blocking agents.
31.3.2 Airway, Respiratory System, and Control of Ventilation
In the practice of pediatric anesthesia, infants and children frequently present to the operating room without intravenous access. As such, anesthesia is induced by the inhalation of increasing concentrations of an inhalational anesthetic agent. In clinical practice for decades, the only agent that was nonpungent to the airway and therefore suitable for this purpose was halothane. However, given its adverse effects on hemodynamic function and the potential to cause morbidity and even mortality, the search continued for alternative agents which led to the introduction of sevoflurane into clinical practice in the 1990s. Given its limited effects on myocardial contractility and chronotropic function when compared with halothane, sevoflurane became the preferred agent for the inhalational induction of anesthesia with the eventual removal of halothane from anesthetic practice. Such a move was prompted by data implicating halothane as the primary agent responsible for the majority of cardiac arrests during general anesthesia in infants and children (Morray et al. 2000). Additionally, given its lower blood-gas partition coefficient, the speed of induction is faster and recovery more rapid with sevoflurane than with halothane.
The inhalational anesthetic agents result in a dose-dependent depression of ventilatory and cardiovascular function. These effects are modified by the coadministration of other medications as well as the presence of comorbid disease processes. With an increasing inspired concentration and anesthetic depth, there is a progressive decrease in alveolar ventilation characterized by a reduction in tidal volume and an increase in PaCO2 in spontaneously breathing patients. Clinically this is manifested by a rightward shift of the CO2 response curve. The inhalational anesthetic agents also blunt the normal ventilatory responses to hypercarbia and hypoxia (i.e., the “hypoxic drive” is depressed). Additionally, these agents may impair oxygenation especially in patients with pulmonary parenchymal disease or atelectasis through the inhibition of hypoxic pulmonary vasoconstriction (HPV) (Benumof et al. 1987). As with many of the other physiologic effects, the impact on HPV and hence oxygenation is dose dependent with limited effects at a MAC of ≤1. Beneficial effects on the airways include a direct effect on bronchial smooth muscle with a decrease in the cytoplasmic calcium availability thereby result in bronchodilatation (Hirshman et al. 1982). Given this effect, the inhalational anesthetic agents have been used effectively outside of the OR for the treatment of patients with refractory status asthmaticus (see below). The airway effects result from both a depression of airway reflexes and a direct effect on the airway smooth musculature (Hirshman et al. 1982; Warner et al. 1990). Although these effects are shared by all of the inhalational anesthetic agents, animal data suggest that the effect may be greatest with halothane (Katoh and Ikeda 1994). However, given its negative inotropic effects and the potentially greater morbidity and mortality with its intraoperative use when compared with other agents, halothane is no longer used in clinical practice.
31.3.3 Cardiovascular System and Hemodynamic Effects
The inhalational anesthetic agents share some hemodynamic effects including a decrease of MAP, a depression of myocardial contractility, and a reduction of myocardial oxygen consumption. The decrease in MAP and cardiac output that occurs with these agents may secondarily affect the function of other end-organ by decreasing perfusion. For example, the MAP reduction may lead to a reduction of CBF as well as hepatic and renal perfusion. Although these vascular beds autoregulate within a specific MAP and thereby maintain flow, these autoregulatory effects may be blunted to some extent by the inhalational anesthetic agents. As with the effects on the control of ventilation, the effects on hemodynamic function and cardiac output are dose dependent. Additionally, these effects are modified by the patient’s intravascular status, underlying myocardial function, and the coadministration of other anesthetic agents.
Although the inhalational anesthetic agents in general depress hemodynamic and cardiovascular function, the specific changes in cardiac output, systemic vascular resistance, and heart rate vary from agent to agent. Isoflurane and desflurane have limited effects on inotropic function and primarily result in vasodilatation with a decrease in systemic vascular resistance and MAP. The vasodilatation results in reflex tachycardia. Rapid increases in the inspired concentration of desflurane may also stimulate the sympathetic nervous system and thereby further increase heart rate. A decrease in heart rate is commonly seen with sevoflurane administered at lower inspired concentrations (0.5–1 MAC), while inspired concentrations greater than 1 MAC may decrease SVR and result in a reflex tachycardia. However, the reflex tachycardia is generally not to the extent seen with isoflurane and desflurane. Halothane on the other hand had little or no effect on SVR and resulted primarily in direct negative chronotropic and inotropic effects resulting in a decreased heart rate and decreased cardiac output.
As the primary hemodynamic effects of isoflurane and desflurane are peripheral vasodilatation, there is a decrease in afterload (SVR) which results in an increase in cardiac output as opposed to the decrease in cardiac output that occurs with sevoflurane, halothane, or enflurane. The reflex tachycardia which frequently occurs with isoflurane and desflurane can increase myocardial oxygen demand, while vasodilatation may lower diastolic blood pressure, thereby reducing myocardial perfusion pressure and myocardial oxygen delivery. Given the imbalance that may occur between myocardial oxygen delivery and consumption, theoretical concerns have been raised regarding the potential for myocardial ischemia in at risk patients. Additionally, with isoflurane, a coronary steal phenomenon may result as there may be vasodilatation of normal coronary vasculature with no effect in areas of fixed coronary stenosis. Due to these concerns, isoflurane should be used cautiously in patients at risk for myocardial ischemia or in patients who are unable to tolerate tachycardia and a decrease in systemic vascular resistance. This may also be a consideration in patients with residual or palliated congenital heart disease in whom alterations in the systemic and pulmonary vascular resistance may significantly affect the ratio of pulmonary to systemic blood flow. These effects on heart rate and SVR may be further aggravated by the concomitant use of other medications or comorbid disease processes.
A final issue of concern with halothane was the potential to sensitize the myocardium to arrhythmogenic effects of endogenous or exogenous catecholamines. This effect was related to its primary chemical structure and is generally not seen with the other inhalational anesthetic agents which are not in the alkane group. This effect can precipitate dysrhythmias especially when there is associated hypercarbia, when used in conjunction with other medications (aminophylline), or in the presence of high circulating catecholamine.
31.3.4 Hepatic and Renal Function
The stability and eventual metabolic fate of the inhalational anesthetic agents is determined by their chemical structure. In general, the newer inhalational anesthetic agents have been modified and developed to undergo little or no metabolism thereby limiting the potential adverse effects related to their metabolic products. The latter is especially true of the newest of these agents, desflurane. Fifteen to twenty percent of halothane is recovered as metabolites compared to 3–5 % for sevoflurane, 2–3 % for enflurane, 0.2 % for isoflurane, and less than 0.1 % for desflurane. In addition to the parent compound, metabolic products from metabolism by the P450 system may also be responsible for the toxicity of these agents. Of significant concern with many of the older inhalational anesthetic agents including halothane was the potential for the development of hepatotoxicity. Although described shortly after the introduction of these drugs into clinical practice, the mechanism of the hepatic injury was later determined to be related to an immune-mediated reaction following exposure to halothane, enflurane, isoflurane, or desflurane (Satoh et al. 1986; Kenna et al. 1988; Subcommittee on the National Halothane Study of the Committee on Anesthesia 1966; Kenna and Jones 1995; Brown and Gandolfi 1987). The metabolic product of the inhalational anesthetic agents, trifluoroacetic acid (TFA), can act as a hapten, binding to hepatocytes and inducing an immune-mediated hepatitis. Although described primarily with halothane, given its higher metabolic processing and thereby a greater production of TFA, there have been anecdotal reports of hepatitis with enflurane, isoflurane, and even desflurane (Kenna and Jones 1995; Brown and Gandolfi 1987; Pohl et al. 1988). However, although 3–5 % of sevoflurane is metabolized, its metabolic pathway is different from the other agents and does not result in the production of TFA.
Clinically, the hepatotoxicity associated with the inhalational anesthetic agents manifests in one of two ways, a mild form and a fulminant form. As mentioned previously, given its greater metabolism, hepatotoxicity occurs primarily with halothane. As such, the majority of the information regarding the inhalational anesthetic agents and hepatotoxicity data related to halothane. The mild form affects 20 % of adults who receive halothane while the fulminant form (halothane hepatitis) occurs in 1 of every 10,000 adult patients following halothane anesthesia. The fulminant form results in massive hepatic necrosis with hepatic insufficiency or failure with a mortality rate of 50–75 %. The majority of the patients (up to 95 %) who develop the fulminant form have had a prior exposure to halothane. In fact, the most important predictive factor for halothane hepatotoxicity is prior multiple anesthetic exposure at short intervals. Additional risk factors include female gender, middle age, obesity, and factors which induce the hepatic microsomal enzymes. The latter may include chronic ethanol ingestion and medications such as isoniazid and the barbiturates. Given the concerns of halothane hepatitis, there was limited use of this agent in the adult population following the introduction of isoflurane and enflurane into clinical practice, but it remained the most commonly used inhalational agent in infants and children until the early 1990s when sevoflurane was introduced. Despite its occurrence in adults, halothane hepatitis was exceedingly uncommon in children (1/200,000) (Wark 1983; Warner et al. 1984). The immunologic mechanism of halothane hepatitis was later shown to be related to an immunologic reaction against the metabolic product, TFA. TFA can act as a hapten binging to hepatocytes and inducting an autoimmune hepatic injury. Because cross-sensitization may occur, all of the inhalational anesthetic agents except sevoflurane should be avoided in patients who have an unexplained postoperative hepatic injury following exposure to an inhalational anesthetic agent. The diagnosis of hepatic injury following inhalational anesthetic agent use can be confirmed with serologic demonstration of the anti-TFA antibody in the sera of patients.
The inhalational anesthetic agents may also result in nephrotoxicity related to either release of fluoride during the metabolism of the parent compound or the production of toxic metabolic by-products. Several of the inhalational anesthetic agents are highly substituted around their carbon atoms with the halide, fluoride. As such, dependent on their metabolic fate, fluoride may be released in significant quantities especially during a prolonged anesthetic or the administration of a high concentration of the agent. Fluoride concentrations greater than 50 μmol/L can result in decreased glomerular filtration rate and renal tubular resistance to vasopressin with nephrogenic diabetes insipidus. One of the earlier of the inhalational anesthetic agents, methoxyflurane, was eventually eliminated from clinical practice due to its potential for nephrotoxicity due to fluoride release. Similar concerns were demonstrated with enflurane. Although a limited amount of enflurane is metabolized when compared with methoxyflurane, its content of fluoride is high enough that serum fluoride concentrations can increase with prolonged administration (Mazze et al. 1977).
Two separate concerns regarding the potential nephrotoxicity of sevoflurane have been raised in the literature: fluoride release during metabolism and the production of the metabolic by-product, compound A (see below). Although high levels of serum fluoride may occur following the prolonged administration of sevoflurane, clinical signs of nephrotoxicity are extremely rare. This is postulated to be the result of the low blood-gas partition coefficient of sevoflurane and its rapid elimination from the body or the fact that sevoflurane unlike methoxyflurane does not undergo metabolism in the kidney, but only in the liver. Therefore, unlike methoxyflurane, there is no local renal release of fluoride.
The second concern raised regarding potential nephrotoxicity of sevoflurane is related to the production of a unique metabolite, a vinyl ether also known as compound A. Compound A is produced during the metabolism of sevoflurane and its reaction with the CO2 soda lime in the carbon dioxide absorber of the anesthesia machine (Morio et al. 1992; Frink et al. 1992). The safe concentration of compound A is unknown in humans as is the mechanism of renal injury (Mazze 1992). Compound A concentrations are increased by several factors including a high inspired concentration of sevoflurane, low fresh gas flows through the system (less than 2 L/min), increasing temperatures of the soda lime canister, decreased water content of the CO2 absorbant, high concentrations of potassium or sodium hydroxides in the CO2 absorbant, and long duration of anesthesia Although clinical data suggest that there is no significant clinical risk of nephrotoxicity during the intraoperative administration of sevoflurane, there are no data regarding compound A concentrations during the prolonged administration of sevoflurane in the pediatric ICU setting. However, given that CO2 absorbers are not generally used when the potent inhalational anesthetic agents are administered in the ICU setting, there are likely to be no concerns regarding compound A.
31.3.5 Miscellaneous End-Organ Effects
All of the potent inhalational anesthetic agents are triggering agents for malignant hyperthermia (MH). MH is an inherited disorder of muscle metabolism with an estimated incidence of 1:15–20,000 in adults and 1:50,000 in infants and children. The primary cellular defect resides in the ryanodine calcium channel in the sarcoplasmic reticulum. Following exposure to any of the inhalational anesthetic agents or the depolarizing neuromuscular blocking agent, succinylcholine, dysfunction of this ion channel results in ongoing and exaggerated calcium release into the cytoplasm. The ongoing increase in cytoplasmic calcium results in ongoing muscle contraction and a hypermetabolic state. The clinical signs and symptoms include tachycardia, hyperthermia, hypercarbia, muscle rigidity, and rhabdomyolysis. The rhabdomyolysis results in hyperkalemia and acidosis. Treatment includes prompt recognition, removal of the triggering agent, and the administration of dantrolene. Additional therapy is aimed at the correction of the hyperkalemia and acidosis. Without appropriate therapy including the administration of dantrolene, mortality exceeds 90 %.
Additional concerns with the inhalational anesthetic agents include anecdotal reports of carbon monoxide (CO) toxicity, cost issues, and alterations of the metabolism of other medications. Reports of CO toxicity have typically occurred in a scenario that involves the first case in the operating room on a Monday morning. The release of carbon monoxide results from the interaction of the inhalational anesthetic agent (most commonly desflurane) and the CO2 absorbant, which has become desiccated because of no use over the weekend (Woehlck 1999; Baum et al. 1995). In vitro studies have demonstrated that CO can be produced by the interaction of desflurane and isoflurane, but not sevoflurane, and the CO2 absorbant (Fang et al. 1995). CO production increases with specific characteristics of the CO2 absorbant including decreased water content, increased temperature, and increased concentration of potassium hydroxide. As with compound A issues, since CO2 absorbants are not generally used in the pediatric ICU setting, this is not likely to become a clinical issue. Additionally, this problem is thought to occur only when the soda lime has become desiccated from the prolonged administration of a high gas flow.
The inhalational agents alter hepatic blood flow and may thereby affect the metabolism of other medications that are administered in the ICU including lidocaine, β-adrenergic antagonists, benzodiazepines, and local anesthetic agents (Reilly et al. 1985). These potential interactions and their clinical effects must be considered when pharmacologic agents are coadministered with the inhalational anesthetic agents.
31.4 Applications in the Pediatric ICU Setting
The inhalational anesthetic agents are administered everyday during surgical procedures worldwide to provide general anesthesia and ensure intraoperative amnesia and analgesia. The clinical characteristics of these agents which make them useful intraoperatively (e.g., rapid onset, rapid awakening upon discontinuation, and ease of control of the depth of sedation) may also make them useful in the pediatric ICU setting. The physiologic effects which include bronchodilatation and anticonvulsant properties may make them effective therapeutic agents in refractory cases of status asthmaticus and status epilepticus.
31.4.1 Procedural Sedation
In addition to their use in the operating room or off-site using standard anesthesia machines, there are limited reports regarding the administration of inhalational anesthetic agents for procedural sedation with novel delivery devices. Several studies demonstrate the efficacy of methoxyflurane as an agent for procedural sedation in various clinical scenarios (Marshall and Ozorio 1972; Babl et al. 2006; 2007). Babl et al. reported the use of methoxyflurane as a prehospital analgesic in a cohort of 105 patients who ranged in age from 15 months to 17 years (median 15 years) (Babl et al. 2006). The indication for analgesia included extremity injuries (81.9 %), abdominal pain (6.7 %), head or facial injury (4.8 %), burns (3.8 %), or multiple trauma (2.8 %). Methoxyflurane was administered by paramedics during the prehospital period using a Penthrox™ inhaler (Medical Developments International, Victoria, Australia), a tubular, handheld device which is primed with liquid methoxyflurane (3 mL) and provides 25–30 min of administration. The device has a 22-mm mouthpiece which can also be fitted with a standard resuscitation mask to aid in delivery in younger patients who cannot use the mouthpiece. The device also has a one-way valve to prevent blowback into the wick which holds the liquid methoxyflurane and an oxygen inlet for the delivery of supplemental oxygen if needed. There is also a dilutor hole which allows the delivery of varying concentrations of methoxyflurane from 0.1 to 0.2 % with the hole open to 0.3–0.4 % with hole occluded. The device is held by the patient and methoxyflurane is delivered as the patient breathes in and out through the device. Public awareness of the device has increased given its use to provide analgesia following an injury and during transport of a participant of the reality television show, Survivor, which is broadcast in the USA (http://www.cbs.com/primetime/survivor2/show/episode06/story.html). In the study of Babl et al. (2006), 96 of the patients received a single 3-mL dose and 9 received 2 doses of methoxyflurane. The inhalational anesthetic agent was administered using the Penthrox™ inhaler; however, in nine patients (generally those less than 5 years of age), a mask was attached to the inhaler instead of having the patient hold the device in their mouth. Ten patients received additional analgesia with intravenous morphine. Pain scores (using a scale of 0–10) decreased from a mean of 7.9–4.5 at 2–5 min and 3.2 at 10 min following the administration of methoxyflurane. Acceptable analgesia was achieved in 88.5 % of the patients as judged by the paramedics and 87 % of the patients as judged by self-report scores. No serious adverse effects were noted.
Although methoxyflurane was used extensively as a general anesthetic agent and for procedural sedation during the 1960s and 1970s, with the development of newer agents and recognition of the potential for nephrotoxicity with methoxyflurane as fluoride and oxalic acid are released during its metabolism, its use decreased markedly and it is no longer available for clinical use in the United States (Mazze 2006). The technique of methoxyflurane using the Penthrox™ inhaler is licensed in Australia for self-administration to conscious, hemodynamically stable patients with trauma-associated pain and for procedural sedation. In some areas of Australia, it is the most commonly used analgesia agent during the prehospital transport of patients, its advantages being not only its efficacy but also its administration via the inhalation route using relatively simple technology.
Sevoflurane has also been administered outside of the operating room setting for procedural sedation. Sury et al. administered sevoflurane via a nasal cannula to provide sedation during magnetic resonance imaging in 13 infants with a median post-conceptual gestational age of 46 weeks (range 40–70 weeks) and a median weight of 4.4 kg (range 3.3–6.5 kg) (Sury et al. 2005). Sevoflurane was delivered from a standard anesthesia machine vaporizer using a flow rate of 2 L/min and delivered via nasal cannulae. The technique was successful in 12 infants using a median maximum inspired sevoflurane concentration of 4 % (range 4–8 %). One infant desaturated to 85 % and required repositioning of the head to maintain a clear airway. With either the use of methoxyflurane using the Penthrox™ inhaler or the administration of sevoflurane via a nasal cannula, there may be concerns regarding environmental pollution and healthcare worker exposure as scavenging may not be feasible.
For procedural “sedation” you should also add a discussion on N2O (this is very common at least in Europe) – this would be a very, very lengthy topic and likely one that is not particularly relevant to the main impetus of the book (mechanical ventilation); if you disagree, please let me know and I can come up with something.
31.4.2 Sedation During Mechanical Ventilation
Although there are several options available for the provision of sedation in the ICU setting, there remains interest in the potential application of the inhalational anesthetic agents for the provision of sedation during mechanical ventilation. To date, the majority of the applications of these agents for sedation in the ICU setting have been in the adult population. In this population, the majority of the reports have outlined favorable experiences with potential advantages over commonly used agents including benzodiazepines or propofol (Kong et al. 1989; Millane et al. 1992; Meiser et al. 2003; Breheny and Kendall 1992). Although many of the studies regarding the use of the inhalational anesthetic agents for sedation are relatively recent, the technique is definitely not a novel idea. In 1969, Prys-Roberts et al. reported the use of these agents in adults to provide sedation during mechanical ventilation and to control the autonomic hyperactivity associated with tetanus (Prys-Roberts et al. 1969). More recently, there has been renewed interest with the use of the inhalational anesthetic agents to provide sedation during mechanical ventilation in the adult population and anecdotal experience reported in the pediatric-aged patient.
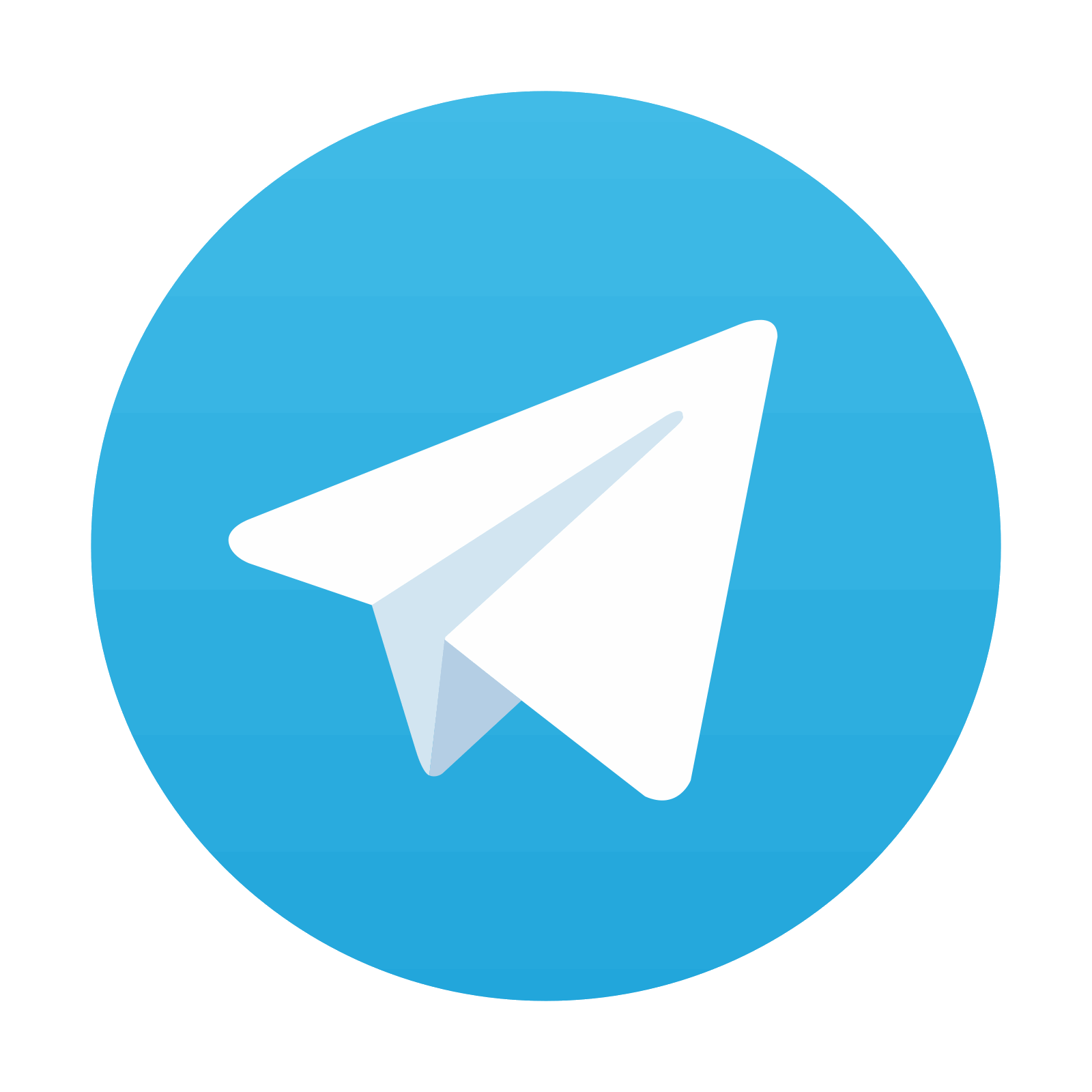
Stay updated, free articles. Join our Telegram channel
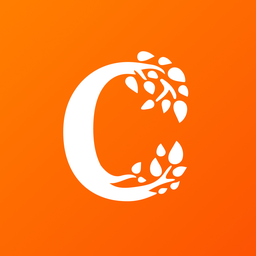
Full access? Get Clinical Tree
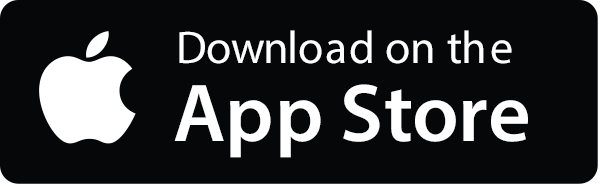
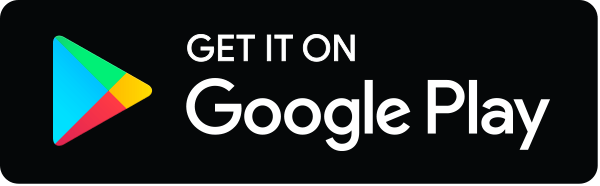