KEY TERMS
Key Terms1
-
Acoustic streaming: movement of tissue or fluid, resulting from the passage of alternating positive and negative pressures of the ultrasound wave. Can also result from movements of bubbles, as a result of changes in pressure.
-
ALARA principle: stands for As Low As Reasonably Achievable, a way to obtain the best, clinically relevant image while keeping ultrasound intensity and exposure as low as possible.
-
Cavitation: bubble activity, secondary to ultrasound insonation. The positive aspect of the ultrasound pressure wave causes compression of the bubble while the negative part, also called rarefactional, causes production of the bubbles or expansion of existing ones. Cavitation can be stable or inertial.
-
Stable cavitation: bubble activity where bubble does not collapse (see inertial cavitation) but is moving back and forth in the tissue or fluid, thus potentially causing the surrounding medium to flow (ie, stream, hence the term streaming).
-
Inertial (previously known as transient) cavitation: bubbles that are compressed and expanded but with each compressing (positive) component, causing the volume to diminish ever more, until collapse occurs. This collapse can generate tremendously elevated temperature and pressure for an extremely short time and over an extremely short space (called an adiabatic reaction). This can result in production of several more bubbles, local cell damage, and/or generation of free radicals.
-
-
Derating: action of multiplying a value measured in water with standard methods by a correction factor to account for the attenuation of the ultrasound field by the tissue traversed by the beam (usually 0.3 dB/cm/MHz).
-
Dwell time: the time during which the ultrasound beam impinges on a specific organ, body part, or entire organism.
-
Mechanical index (MI): expresses the potential for nonthermal (also known as mechanical) effects in tissues traversed by the ultrasound wave. Depends on the pressure and the frequency
.
-
Output Display Standard (ODS): actual name—Standard for Real-Time Display of Thermal and Mechanical Acoustic Indices on Diagnostic Ultrasound Equipment. Introduced to make end users aware, in real time, of the potential effects of ultrasound in tissues. See also mechanical index and thermal index.
-
Radiation force: force resulting from absorption of some of the energy of the acoustic wave by tissue and transformation into heat.
-
Scanned mode: refers to the ultrasound beam moving through the field, with energy distributed over a large volume, such as in B-mode and color-flow Doppler.
-
Thermal index (TI): expresses the potential for temperature increase in tissues traversed by the ultrasound wave. It is given by the ratio of the power emitted by the transducer to the ultrasonic power required to raise tissue temperature by 1°C for the specific exposure conditions. This is a relative indication and does not necessarily correspond to the actual temperature increase. One of three thermal indices is displayed, based on whether soft tissue (TIS, mostly first and early second trimesters), bone (TIB, late second and third trimesters), or adult cranium (TIC) is being scanned.
-
Unscanned mode: the ultrasound beam is stationary with power concentrated along a single line, such as in M-mode and spectral Doppler.
INTRODUCTION
“Is this safe for my baby?” Ultrasound practitioners hear this question almost every day in clinical practice. The answer generally given is: “Of course. Ultrasound is not x-rays, it is not invasive; it has been used for close to sixty years and is perfectly safe.” While this answer may, in fact, contain some correct facts (ultrasound is not x-rays), the concept of perfect safety is not scientifically valid, and furthermore, the level of knowledge regarding potential effects of ultrasound in tissues is, by and large, very low among end-users of this technology. Ultrasound in obstetrics is convenient, painless, and results are available immediately. The belief exists that is does not pose any risk to the pregnant patient or her fetus. Ultrasound, however, is a form of energy and, as such, has effects in biological tissues (bioeffects). The physical mechanisms responsible for these effects are nonthermal (mechanical) or thermal. The nonthermal mechanisms can further be separated into acoustic cavitation (inertial and noninertial) and noncavitational mechanisms, ie, acoustic radiation force (time-averaged force exerted by the ultrasound beam), acoustic radiation torque (producing in the insonated tissue a tendency to rotate or spin), and acoustic streaming (circulatory flow). It is the role of science to show whether any of these bioeffects may be harmful. The question has been debated since the introduction of ultrasound in clinical obstetrics, particularly as it relates to the fetal nervous system2,3 and continues to be discussed currently.4-9(1)
This chapter presents basic notions of acoustics and physics as they relate to ultrasound, examines some literature on bioeffects and the safety of ultrasound, reviews statements of various ultrasound organizations, and affords a practical approach to limit the potential risks to the fetus of exposure to diagnostic ultrasound (DUS).
BASIC PHYSICS OF ULTRASOUND
A detailed description of ultrasound physics can be found in various publications.10-12 However, certain properties of ultrasound are very important when trying to understand safety and bioeffects. Equally important are tissue characteristics, such as attenuation coefficient. A basic knowledge of instrument controls (“knobology”) is essential not only for appropriate clinical usage, but it is imperative to avoid potential harm.
Sound is a mechanical vibratory form of energy. It propagates through a medium by means of the motions of the particles in the medium, under the influence of the alternating positive and negative components of the wave. Megapascal (MPa) is the unit for pressure. Ultrasound instrumentation can generate peak pressures of 5 MPa and above. This is in comparison to the atmospheric pressure, which is 0.1 MPa. Several other characteristics define the ultrasound beam. The ultrasonic wave progresses in the insonated tissue at a velocity that is related to the sound characteristics as well as the tissue properties. For practical purposes, the average speed of sound propagation in biological tissues is estimated at 1540 ms/sec. Frequency is the number of cycles per second, measured in hertz (Hz). The limits of human hearing spans from approximately 20 to 20,000 Hz. Diagnostic ultrasound is, generally, 2 to 10 million Hz (megahertz, MHz). Wavelength is the distance between 2 corresponding points on a particular wave. It is inversely proportional to the frequency. Equipment resolution (the shortest distance between 2 objects or parts of an object to be represented by 2 separate echoes) depends on the wavelength: axial resolution ranges between 2 and 4 wavelengths. Hence, the shorter the wavelength (ie, the higher the frequency), the better the resolution (the distance between 2 points is smaller). The trade-off is that the higher the frequency (better resolution), the lower the penetration of the beam through a given tissue (Figure 1-1).
Diagnostic ultrasound is pulsed, ie, pulses of acoustic energy separated by “silent” gaps. The number of pulses occurring in 1 second is the pulse repetition frequency (PRF) and is controlled by the instrument in B-mode. In Doppler mode, it can be altered by the end user. Another important parameter is the duty factor: this is the fraction of time that the pulsed ultrasound is on. With an increase in PRF, the duty factor increases. The pulse amplitude reflects pressure and is the maximum variation from the baseline, expressed in MPa’s. Since the ultrasound wave is sinusoidal, there are periods of positive and negative pressure. When the ultrasound wave exerts pressure on the resisting insonated tissue, work is produced. The ability of the wave to do work is its energy (in joules). The rate at which the energy is transformed from one form to another is the power (in watts or milliwatts). Intensity represents the rate at which energy passes through area unit. Average intensity of a beam is expressed by the beam power (in milliwatts, mW), divided by the cross-sectional area of the beam (in cm2) and is, therefore, expressed in mW/cm2. As stated earlier, DUS is performed with a pulsed wave. The intensity is proportional to the square of the instantaneous ultrasound wave pressure. There are pulses of energy intermingled with periods where no energy is emitted. Depending on the time and location of the measurement, several parameters can be described in relation to time or space: temporal peak intensity (the greatest intensity), average intensity over time, ie, including “silent” time between pulses (temporal-average intensity), maximal intensity at a particular location (spatial-peak intensity), as well as average-spatial intensity. By combining time and space, 6 intensities can be described: spatial average–temporal average (ISATA), spatial average–pulse average (ISAPA), spatial average–temporal peak (ISATP), spatial peak–temporal average (ISPTA), spatial peak–pulse average (ISPPA), and spatial peak–temporal peak (ISPTP). The most practical, and commonly referred to, is the ISPTA.
The maximal permitted value varies by clinical application. This had been determined in 1976 by the US Food and Drug Administration (FDA),13 but was modified in 1986.14 The most recent definition dates from 1992.15 These values are shown in Table 1-1. One can observe from the table that, for fetal imaging, the ISPTA has been allowed to increase by a factor of almost 16-fold from 1976 and almost 8-fold from 1986 to 1992, yet, all epidemiological information available regarding fetal effects predates 1992. A remarkable fact is that intensity for ophthalmic examination has not changed from the original 17 mW/cm2, a value approximately 42.5 times lower than the present allowed value for fetal scanning. This will be addressed in more detail further in the chapter.
When the ultrasound wave travels through a medium, its intensity diminishes with distance.16 In completely homogeneous, idealized materials, the signal amplitude would be reduced only because the wave is spreading. Biologic tissues, however, are different and induce further weakening by absorption and scattering (an effect called attenuation) and by reflection. Many models have been described to help calculate attenuation, particularly in obstetrical scanning,17 but the most commonly used model uses an average attenuation of 0.3 dB/cm/MHz.18 It is important to note that the attenuation increases logarithmically with frequency and distance traveled. Technically, many measurements of acoustic power are performed in water, which has almost no attenuation. To apply these calculations to tissues, values are multiplied by this factor, an action called derating.19 Absorption is the sound energy being converted to other forms of energy, and scattering is the sound being reflected in directions other than its original direction of propagation. Since attenuation is proportional to the square of sound frequency, it becomes evident why higher frequency transducers have less penetration (but better resolution; see Figure 1-1). One needs, therefore, to be closer to the organ of interest, such as through transesophageal or, in obstetrics and gynecology, transvaginal scanning. Another possibility is increasing the power of the instrument, resulting in improved resolution, as depicted by the green arrow in Figure 1-1. This is seemingly simple, but instrument outputs are regulated in the United States (see The Output Display Standard section). Another important parameter is acoustic impedance, which can be described as the opposition to transmission or progression of the ultrasound wave. It is proportional to the velocity of sound in the tissue (estimated at 1540 ms/sec) and to the tissue density.
Although some publications of various instrument outputs are available,20-22 these are generally quickly outdated, since manufacturers introduce new commercial machines to the market (or modify existing ones) at a rate too fast for immediate objective evaluation. From a clinical standpoint, there is no easy way to verify the actual output of the instrument in use. In addition to the variety of instruments, each attached transducer will generate a specific output, further complicated by the different modes that may be applied.23 When comparing modes, the ISPTA increases from B-mode (34 mW/cm2, average) to M-mode to color Doppler to spectral Doppler (1180 mW/cm2). Average values of the temporal averaged intensity are 1 W/cm2 in Doppler mode but can reach 10 W/cm2.23 Therefore, caution should be exercised when applying Doppler mode, particularly in the first trimester. Color Doppler, while having higher intensities than B-mode, is still much lower than spectral Doppler. This is mainly due to the mode of operation—sequences of pulses, scanned through the region of interest (ROI or “box”). Most measurements are obtained from manufacturers’ manuals, having been derived in laboratory conditions. Real-life conditions may be different.24 Furthermore, machine controls can alter the output. If one keeps in mind that, for instance, the degree of temperature elevation is proportional to the product of the amplitude of the sound wave by the pulse length and the PRF, it becomes immediately evident why any change (augmentation) in these properties can add to the risk of elevating the temperature, a potential mechanism for bioeffects (see Thermal Effects). The 3 important parameters under end-user control are the scanning (or operating) mode, including transducer choice; the system setup and output control; and the dwell time.
-
Scanning mode: as mentioned previously, B-mode carries the lowest risk, and spectral Doppler carries the highest (with M-mode and color Doppler in between). High pulse repetition frequencies are used in pulsed Doppler techniques, generating greater temporal average intensities and powers than B- or M-mode, and hence greater heating potential. An additional risk is that since, in spectral Doppler, the beam needs to be held in relatively constant position over the vessel of interest, there may be a further increase in temporal average intensity. Naturally, transducer choice is of great consequence since it will determine frequency, penetration, resolution, and field of view.
-
System setup: starting or default output power and, particularly, mode (B-mode, Doppler, etc) control changes. A subtler element is fine tuning performed by the examiner to optimize the image and influence output but with no visible effect (except if one follows thermal index [TI] and/or mechanical index [MI] displays). Controls that regularize output include focal depth (usually with greatest power at deeper focus but occasionally, on some machines, with highest power in the near field); increasing frame rate; and limiting the field of view, for instance, by high-resolution magnification or certain zooms (Figure 1-2).
-
In Doppler mode, changing sample volume and/or velocity range (all done to optimize received signals) changes output. A very important control in every mode is receiver gain. It often has similar effects to the above controls on the recorded image but none on the output of the outgoing beam, and is therefore completely safe to manipulate (Figure 1-3). In other words, the receiver gain should be maximized before output is increased. In addition, over the years, output of instruments has increased.22, 25
-
Dwell time: is directly under the control of the examiner. Interestingly, dwell time is not taken into account in the calculation of the safety indices (thermal index, TI and mechanical index, MI,) nor, in general, until now, reported in clinical or experimental studies. However, one needs to remember that it takes only one pulse to induce cavitation, and about a minute to raise temperature to its peak. Directly related with dwell time is examiner experience: knowledge of anatomy, bioeffects, instrument controls, and scanning techniques. It can be safely assumed that the more experienced the examiner, the less scanning time will be needed to obtain the needed diagnostic images.
Figure 1-3.
A: Image obtained with 100% power (blue arrow). Note MI = 1.2 and TI + 0.1 (yellow arrow). B: Power has been reduced to 85% (blue arrow). Note MI = 0.7 and TI + 0.0 (yellow arrow). This image is less diagnostic. C: Receiver gain has been increased. Power is unchanged from B (nor are MI and TI) but image is as diagnostic as A.



A standardized method of providing the end user a parameter related to acoustic output and expressing potential for bioeffects is clearly needed; hence, the generation of the Output Display Standard, based on the 2 most likely interactions of ultrasound with tissues: thermal and nonthermal or mechanical.26
THERMAL EFFECTS
Normal core human body temperature is generally accepted to be 37°C (98.6°F) with a diurnal variation of ±0.5°C to 1.0°C, although 36.8°C ± 0.4°C (95% confidence interval) may be closer to the actual mean for large populations.27 During the entire gestation, temperature of the human embryo/fetus is higher than maternal core body temperature28 and gradually rises until the final trimester (near term). The fetal temperature generally exceeds that of the mother by 0.5°C.29 Thermally induced teratogenesis (production of congenital malformations in an embryo or fetus) has been demonstrated in many animal studies, as well as several controlled human studies.30 While elevated maternal temperature in early gestation has been associated with an increased incidence of congenital anomalies,31 the majority of these studies do not involve ultrasound-induced temperature elevation.
Edwards and others have demonstrated that hyperthermia is teratogenic for numerous animal species, including humans,32 and suggested a 1.5°C temperature elevation above the normal value as a universal threshold.33 Some scientists believe that there are, indeed, temperature thresholds for hyperthermia-induced birth defects, hence the As Low As Reasonably Achievable (ALARA) principle. There is, however, some evidence that any positive temperature differential for any period of time has some effect. In other words, that there may be no thermal threshold for hyperthermia-induced birth defects.34 From careful thermal dose determinations, derived from published literature in this area, it may be that hyperthermia-induced birth defects are produced in accordance with an Arrhenius relation for chemical rate effects, and thus have no threshold.35 Any temperature increment for any period of time has some effect. Likewise, the higher the temperature differential or the longer the temperature increment, the greater the likelihood of producing an effect. Gestational age is a vital factor: milder exposure during the preimplantation period can have similar consequences to more severe exposures during embryonic and fetal development and can result in prenatal death and abortion or a wide range of structural and functional defects.
The organ at greatest risk is the central nervous system (CNS) due to a lack of compensatory growth of damaged neuroblasts. In experimental animals the most common defects are of the neural tube, microphthalmia, cataract, and microencephaly, with associated functional and behavioral problems.32 Defects of craniofacial development including clefts,36 the axial and appendicular skeleton,37 the body wall, teeth, and heart38 are also commonly found. Hyperthermia in utero (due to maternal influenza) has been described as a risk factor for congenital anomalies39,40 and subsequent childhood psychological/behavioral disturbances41 and, more particularly, schizophrenia.42 Nearly all these defects have been found in human epidemiological studies following maternal fever or hyperthermia during pregnancy. It should be emphasized that these investigations have not involved ultrasound-induced hyperthermia effects. Yet, there are data on the effects of hyperthermia and measurements of in vivo temperature induced by pulsed ultrasound, but not in human beings.43-46 These data have been widely reviewed.32,35,47-49 There is, however, a serious lack of data that examine the effects of ultrasound while rigorously excluding other confounding factors. Two widely accepted facts are that ultrasound has the potential to elevate the temperature of the tissues being scanned,50-53 and elevated maternal temperature, whether from illness or exposure to heat, can produce teratologic effects.31,32,35,54-56 The major question is, therefore, whether DUS can induce a harmful rise in temperature in the fetus.57-59 Some believe that this temperature rise is, in fact, a major mechanism for ultrasound bioeffects.30,35 Temperature elevation in the insonated tissue can be calculated and estimated fairly accurately if the field is sufficiently well characterized.60,61 For prolonged exposures, temperature elevations of up to 5°C have been obtained.57 Temperature change in insonated tissues depends on the balance between heat production and heat loss. A particular tissue property that strongly influences the amount of heat transported is local perfusion, which very clearly diminishes the risk, if present. Similar experimental conditions caused a 30% to 40% lower maximal temperature increase in live versus dead sheep fetuses exposed in the near field,45 while in guinea pig fetuses exposed at the focus the difference was approximately 10%.46 These findings were estimated to be secondary to vascular perfusion in live animals. A significant cooling effect of vascular perfusion was observed only when the guinea pig fetuses reached the stage of late gestation near term, when the cerebral vessels were well developed. In the midterm, there was no significant difference when guinea pig fetal brains were exposed, alive (perfused) or postmortem (nonperfused), in the focal region of the ultrasound beam.46
In early pregnancy, under 6 weeks gestation, there appears to be minimal maternal-fetal circulation, that is, minimal fetal perfusion, which may potentially reduce heat dispersion.62 The lack of perfusion is one reason why the spatial peak-temporal average intensity (ISPTA) for ophthalmic applications has been kept very low, in fact much lower than peripheral, vascular, cardiovascular, and even obstetric scanning, despite the general increase in acoustic power that was allowed after 1992 (see Table 1-1). There are some similarities in physical characteristics between the early, first-trimester embryo and the eye. Neither is perfused; they can be of similar size; and protein is present (in an increasing proportion in the fetus). As mentioned previously, one must then wonder why, from the time intensities were checked and recommended in clinical practice, ISPTA was from the beginning and has continued to be maintained at 17 mW/cm2, while for fetal imaging it was allowed to reach 720 mW/cm2, up from 46 mW/cm2. At about weeks 4 to 5, the gestational sac is about the size of the eye (2.5 cm in diameter), and by week 8 it is around 8 cm in diameter. This may allow whole-body fetal scanning (and possibly temperature increase), a concept that is generally ignored in the literature dealing with thermal effects of ultrasound (Figure 1-4).
The issue of transducer heating, which may be particularly relevant in the first trimester, specifically if performing endovaginal scanning, is also often ignored.63,64 There are additional concerns in early gestation because of minimal or lack of perfusion. Only at about weeks 10 to 11 does the embryonic circulation actually linkup with the maternal circulation.65 There may thus be some underestimation of the actual DUS-induced temperature in early gestation, mainly because of the absence of perfusion. The perfusion issue is in addition to modifications of tissue temperature due to ambient maternal and fetal temperatures. Furthermore, motions (even very small) of the examiner’s hand as well as the patient’s breathing and body movements (in the case of obstetric ultrasound, both the mother and the fetus) tend to spread through the region being heated. However, for spectral (pulsed) Doppler studies, it is necessary to have the transducer as steady as possible. This is because, in general, blood vessels are small in comparison to the general organ or body size being scanned with B-mode imaging, and hand movements while performing Doppler studies will have more undesired effects on the resulting image. As described earlier, the intensity (ISPTA) and acoustic power associated with Doppler ultrasound are the highest of all the general-use categories. Ziskin66 reported that among 15,973 Doppler ultrasound examinations, the average duration was 27 minutes (and the longest 4 hours!).
There is a mathematical/physical relation between temperature elevation and several beam characteristics. The elevation is proportional to the product of the wave amplitude, length of the pulse, and PRF. Hence, manipulating any of these via instrument controls will alter the in situ conditions. It is clear that temperature increases of 1°C are easily reached in routine scanning.67 Elevation of up to 1.5°C were obtained in the first trimester and up to 4°C in the second and third trimesters, particularly with the use of pulsed Doppler.68 There is a large body of literature on heat shock proteins (HSPs), the production of which is triggered by a core temperature increase and the function of which is to protect against hazardous effects of elevated temperature as well as to induce some thermotolerance, ie, the ability to withstand higher elevations than in the past, with no harmful results.69 While their production is activated by whole-body temperature elevation, and may be speculated in ultrasound-induced thermal effects, it has not been shown to actually occur during experimental (or clinical) insonation.
MECHANICAL EFFECTS
Ultrasound bioeffects also occur through mechanical mechanisms.70,71 These are interactions between the ultrasound wave and the tissue that do not cause a significant degree of temperature increase (less than 1°C above physiologic temperature). These include acoustic cavitation as well as radiation torque and force, and acoustic streaming secondary to propagation of the ultrasound waves. While included in this category, some effects are, in fact, the result of the mechanical interaction but are actually physical (shock wave) or chemical (release of free radicals) effects. Table 1-29 summarizes nonthermal effects described in the literature in laboratory or animal experiments—and not in humans—which may be pertinent to fetal ultrasound.
Free-radical generation Increase in cell membrane permeability Erythrocyte agglutination Growth restriction (transient decrease) DNA single-strand break Increased sister chromatid exchange Increased mutation frequency Capillary petechiae Vasoconstriction Lung microvascular hemorrhage Intestine microvascular hemorrhage Neuronal migration delay Auditory tract stimulation Tactile radiation pressure perception effect Cardiac, premature contractions |
Investigations with laboratory animals clearly indicate that nonthermal interactions of ultrasound fields with tissues can produce biological effects in vivo.71 It is interesting to note that chemical effects of ultrasound were described more than 80 years ago!72 Cavitation seems to be the major factor in mechanical effects73 as it has been demonstrated to occur in living tissues under ultrasound insonation.74,75 Two types of cavitation can be described—stable and inertial (previously defined as transient)—both of which need the presence of gas bubbles to occur. Stable cavitation indicates vibrations or small backward and forward movements with possible resulting microstreaming. Inertial cavitation indicates expansion and reduction in volume, secondary to alternating positive and negative pressures generated by the ultrasound wave. Expansion in growth is less with each cycle until collapse occurs with production of very high pressure (hundreds of atmospheres) and very elevated temperature (thousands of degrees), but on such a small area (less than 100 nm) and for such a brief time (few tens of nanoseconds) that it will not be felt and is very hard to measure (adiabatic reaction—occurring without the gain or loss of heat) but can produce microstreaming—a phenomenon that has been described also with no clear involvement of bubbles,76-78 or even release of free radicals.79,80
Acoustic streaming is easily demonstrated by watching ultrasound-induced movements of solid-matter-containing fluids in insonated cavities.
Radiation torque refers to the induction, in objects found in the acoustic field, of rotation or of the tendency to rotate. Biological effects of ultrasound in animals such as local intestinal,81 renal,82 and pulmonary83 hemorrhages have been attributed to mechanical effects, although cavitation could not always be implicated. Furthermore, since gas bubbles do not seem to be present in fetal lungs or bowels (where effects have been described in neonates or adult animals), the risk from mechanical effect secondary to cavitation appears to be minimal.84 There are several other effects that do not appear to involve cavitation such as tactile sensation of the ultrasound wave, auditory response, cell aggregation, and cell membrane alteration. Hemolysis has also been reported.85 It seems, however, that the presence of some cavitation nuclei is necessary for hemolysis to occur. At present, there is no clear clinical indication for the use of ultrasound contrast agents (a source of cavitation nuclei, when injected into the body before ultrasound examination) in fetal ultrasound, and to date, no studies have specifically investigated the interaction of ultrasound and microbubble contrast agents in fetal tissues in vivo. Nevertheless, it should be noted that in the presence of such contrast agents, fetal red blood cells are more susceptible to lysis from ultrasound exposure in vitro.86
Additionally, fetal stimulation caused by pulsed ultrasound insonation has been described, with no apparent relation to cavitation.87 This effect may be secondary to radiation forces associated with ultrasound exposures. These forces were suspected at the earliest stages of ultrasound research88 and are known to possibly stimulate auditory,89 sensory,90 and cardiac tissues.91 No harmful effects of DUS, secondary to nonthermal mechanisms, have been reported in human fetuses. A very intriguing nonthermal effect of ultrasound is acceleration of bone fractures healing in animals and humans.92 Because of these known effects of ultrasound in living tissues and the fact that pressures involved with Doppler propagation are much higher than B-mode, caution is further recommended, based on scientific evidence of potential effect, particularly in the first trimester.93
THE OUTPUT DISPLAY STANDARD
In 1992, the FDA yielded to pressure from ultrasound clinical users as well as manufacturers to increase the power output of instruments. The rationale for this request was that higher outputs would generate better images, and thus improve diagnostic accuracy. To allow clinical users of ultrasound to use their instruments at higher powers than originally intended and to reflect the two major potential biological consequences of ultrasound (mechanical and thermal, see above), the American Institute of Ultrasound in Medicine (AIUM), the National Electrical Manufacturers’ Association (NEMA), and the FDA (with representatives from the Canadian Health Protection Branch, the National Council on Radiation Protection and Measurements,94 and 14 other medical organizations30) developed a standard related to the potential for ultrasound bioeffects. The full name was the Standard for Real-Time Display of Thermal and Mechanical Indices on Diagnostic Ultrasound Equipment, generally known as the Output Display Standard or ODS.15 The importance of this document and what it describes is that it represents historically the first attempt at providing the end user with quantitative safety-related information. One important result is that the end users are able to see how manipulation of the instrument controls during an examination causes alterations in the output and, thus, on the exposure. As a consequence, for fetal imaging the output, as expressed by the ISPTA, went from a previous value of 92 to 720 mW/cm2 (see Table 1-1).
To allow the output to reach such levels, the manufacturers were requested to display, on screen and in real-time, two types of indices with the intent of making the user aware of the potential for bioeffects, as described earlier. These indices are the thermal index (TI), to provide some indication of potential temperature increase, and the mechanical index (MI), to provide indication of potential for nonthermal (ie, mechanical) effects15,30,95 (Figure 1-5). The TI is the ratio of total acoustic power to the acoustic power estimated to be required to increase tissue temperature by a maximum of 1°C. It is an estimate of the maximal temperature rise at a given exposure. There are 3 variants: for soft tissue (TIS), to be used mostly in early pregnancy when ossification is low; for bones (TIB), to be used when the ultrasound beam impinges on bone at or near the beam focus, such as late second and third trimesters of pregnancy; and for transcranial studies (TIC) when the transducer is essentially against bone, mostly for examinations in adult patients, but also in neonatal scanning, which is an area that is, generally, ignored. These indices were required to be displayed if equal to or over 0.4. It needs to be made very clear that TI does not represent an actual or an assumed temperature increase. It bears some correlation with temperature rise in degrees Celsius but in no way allows an estimate or a guess as to what that temperature change actually is in the tissue.95 The MI represents the potential for nonthermal damage in tissues but is not based on actual in-situ measurements. It is a theoretical formulation of the ratio of the pressure to the square root of the ultrasound frequency (hence, the higher the frequency, the lesser risk of mechanical effect).
Both the TI and MI can and should be followed as an indication of change in output during the clinical examination with higher values indicating the potential for higher thermal and nonthermal effects than lower values. A clear mandate in the ODS original document was education of the end user as a major part in the implementation of the indices. Attempts have been made to educate the end users,96 but, unfortunately, this aspect of the ODS does not seem to have succeeded as end users’ knowledge of bioeffects, safety, and output indices is found lacking.97,98
In a questionnaire that was distributed to ultrasound end users (82% were obstetricians) attending review courses and hospital grand rounds, only 17.7% gave the correct answer of the definition of the TI, and only 3.8% described MI properly. Almost 80% of end users did not know where to find the acoustic indices when various responses included the machine documentation, a textbook, a complicated calculation or in real time on the ultrasound monitor (the correct answer).97 Similar results were recorded in surveys abroad, performed in Europe, Asia, or the Middle East98,99,100,101 indicating that clinical end users worldwide show poor knowledge regarding safety issues of ultrasound during pregnancy.102,103 More recently, knowledge of residents in obstetrics and gynecology was also found to be grossly lacking 104 and, furthermore, similar results were obtained when surveying sonographers, with no difference in years of experience.105
Compliance with the ALARA (as low as reasonably achievable) principle by practitioners seeking credentialing for nuchal translucency (NT) measurement between 11 and 14 weeks’ gestation was evaluated. Only 5% of the providers used the correct TI type (TIb) at lower than 0.5 for all submitted images, 6% at lower than 0.7, and 12% at 1.0 or lower. A TI (TIb or TIs) higher than 1.0 was used by 19.5% of the providers. Proficiency in NT measurement and educational background (physician or sonographer) did not influence compliance with ALARA. The authors concluded that clinicians seeking credentialing in NT do not demonstrate compliance with the recommended use of the TIb in monitoring acoustic output.106
Furthermore, several assumptions were made, which prompts some questions on the clinical value of these indices. Maybe the most significant (from a clinical aspect) is the choice of the homogeneous attenuation path model (defined as the H3 model), with an attenuation coefficient of 0.3 dB/cm/MHz, as detailed previously in Tissue Characteristics. The reason to employ models of that nature is the impossibility, for obvious reasons, to perform certain measurements in pregnant women. This coefficient may be an overestimation of the attenuation in many clinical scenarios, a situation that would underestimate the actual exposure. In National Council on Radiation Protection and Measurements (NCRP) report number 140,30 there is an entire chapter (Chapter 9) indicating conditions where both indices may be inaccurate, eg, long fluid path (full bladder, amniotic fluid, ascites, or hydrocephalus) or path through increased amounts of soft tissue such as obese patients. Because of these uncertainties, the accuracy of the TI and MI may be within a factor of 2 or even 6.107 For example, an on-screen TI of 1 may correspond to an actual value of 0.5°C or 2°C if the error factor is 2, but possibly 0.33°C or 6°C, if the error factor is 6 (as previously stated, these are not actual temperature indications). A further disturbing and confusing element is that outputs reported by manufacturers are not necessarily equivalent to those calculated in the laboratory.108
Risk means the chance or the possibility of loss or bad consequence. It refers to the possibility, with a certain degree of probability, of damage to health, environment, and objects, in combination with the nature and magnitude of the damage.109 These are the 3 important characteristics of risk: probability of occurrence, and nature and magnitude of harm. It has been, specifically, applied to the use of medical instruments.110 A complicating factor that makes definition and classification difficult is that the concept of risk means various things to different people. Age, background, education, morals, religion, and many other traits will direct this evaluation and not only the absolute possible result of the activity, putting the participant at risk. For instance, in bungee jumping, rupture of the elastic cord and subsequent death may be, indisputably, the worst possible outcome, but different people evaluate this and make decisions that are not necessarily based on this absolute result. Furthermore, the reason to take a possible risk has to be taken in consideration.
Two approaches are possible in risk evaluation: how much harm is acceptable to obtain the desired results (risk-benefit ratio) or how much harm can be avoided by withholding the action or modifying it (the precautionary principle). The risk-benefit principle is what is almost universally used in medicine to justify a medical diagnostic procedure (such as ultrasound) or a therapeutic intervention. If the benefit to be obtained from the procedure in terms of diagnosis (ultrasound) or intervention (a newly discovered and not yet commercialized cancer or AIDS drug, for instance) is deemed to be sufficient, then, even if this diagnostic or interventional procedure carries some risks (recognized or presumed to be possible), the benefit overrides these risks, assuming the subject understands those risks and is willing to take them. The precautionary principle (PP) is a diametrically opposed ethical, political, and economic approach stating that if a certain action may cause severe damage to the public, in the absence of a scientific consensus that harm would not ensue, the burden of proof falls on those who would advocate taking that action.111 This principle is much less familiar to the medical field, although “first do no harm” is its direct application, but it may be extremely relevant when considering safety and risks of a procedure, such as prenatal ultrasound. The concept originated in the 19th century when John Snow, a London, UK, physician, determined that cholera was due to the extensive, common use of an unclean water supply and recommended closing of this source of water, although it was the sole one in a large vicinity.112 This may have been the first epidemiological analysis of a disease. Although the beginning of the PP was medical, it became a social idea in Germany in the 1930s as Vorsorge, “forecaring.” This later became the Vorsorgeprinzip, the forecaring or precautionary principle, in West German environmental law in the 1970s.113 The idea was adopted by decision and policy makers but, remarkably, much more extensively in Europe than in the United States. Some key concepts in the original formulation were environmental harm to a population and responsibility: “When an activity raises threats of harm to human health or the environment, precautionary measures should be taken even if some cause and effect relationships are not fully established scientifically. In this context the proponent of an activity, rather than the public, should bear the burden of proof” (the Wingspread Statement on the Precautionary Principle114). From environmental research it spread to toxicology and was first applied only recently in the United States to a clinical medical field.115 However, several medical mishaps clearly belong to the history of the development of the PP—from the diethylstilbestrol debacle116 to the thalidomide tragedy.117 While referring mostly to environmental issues, such as global warming, the PP can certainly be extended to other medical activities (such as diagnostic ultrasound) and be applied to individuals (such as fetuses). The simple enunciation of the principle, particularly in reference to diagnostic ultrasound in general, and entertainment ultrasound in particular, is that even if a particular action or procedure has not been proven to be harmful, it is better to avoid it so as not to take the risk until safety is established through clear, scientific evidence, popularly expressed as “better safe than sorry.”118 This is also the basis of the Hippocratic Oath, which includes the recommendation to first do no harm. A major difference with the risk-benefit principle is that proponents of the PP believe that public action is necessary if there is any evidence of likely or substantial harm, however limited but plausible, and the burden of proof is shifted from showing the presence of risk to demonstrating its absence.119 As such, epidemiologic research on chronic diseases and the use of surrogates for human studies (eg, animal research or tissue cultures) have been shown to be uncertain.120 There are several variations of the PP, but all have some common key elements:
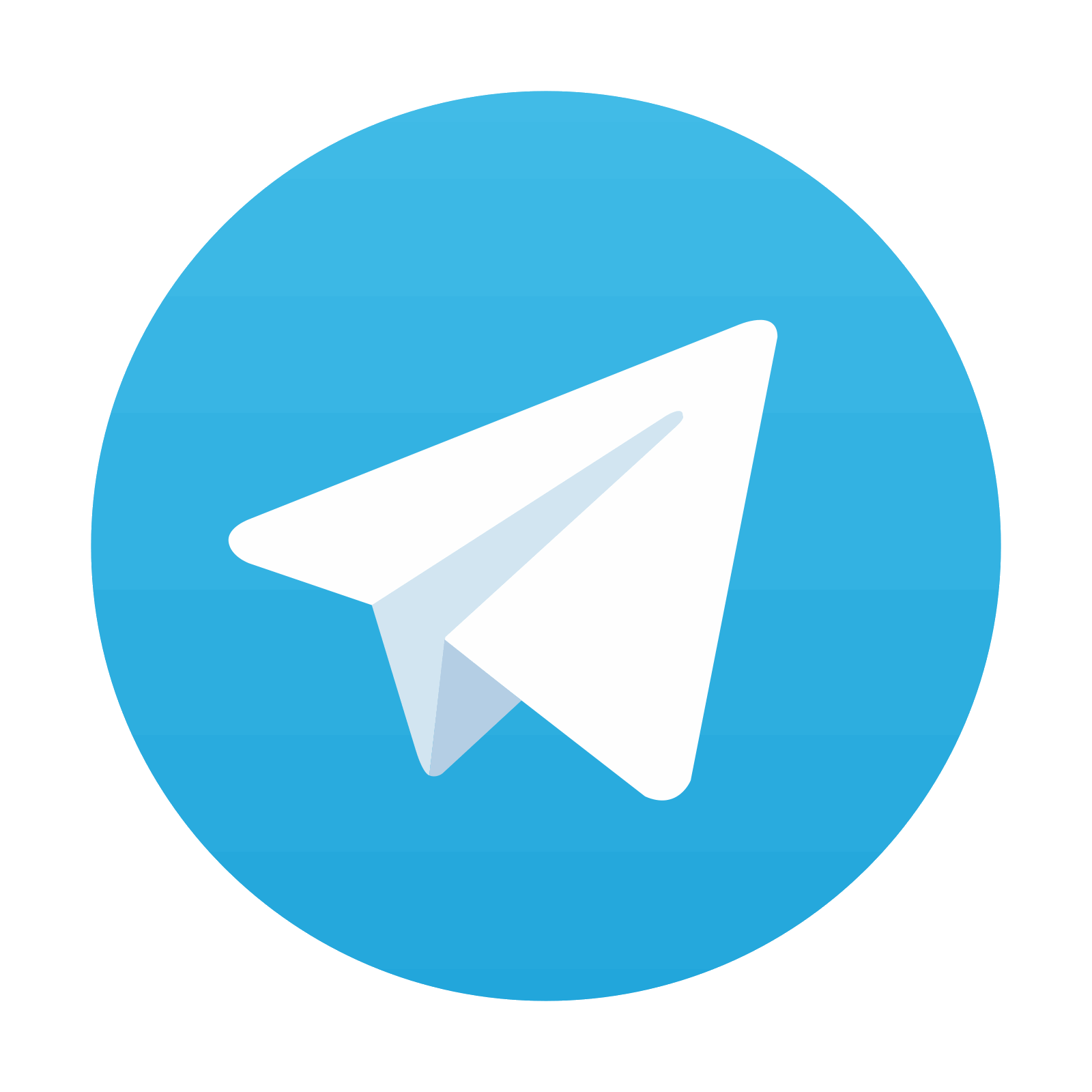
Stay updated, free articles. Join our Telegram channel
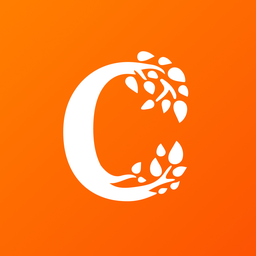
Full access? Get Clinical Tree
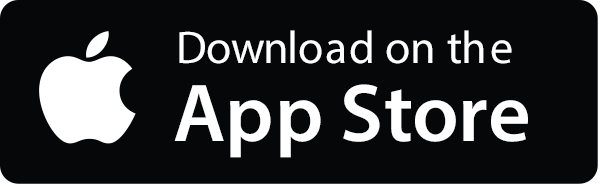
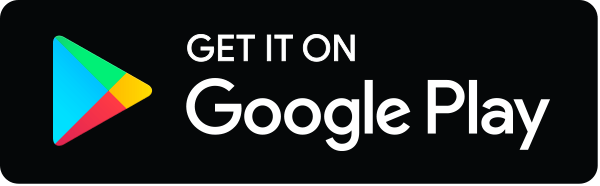