Tissue Engineering
Scientific progress has improved outcomes for patients with organ failure, but many of these therapies are temporary, expensive, and require specialized care. Historically, organ replacement has been managed with inexact tissue substitutions such as colon interposition for the esophagus, materials such as Dacron for vascular grafts, extracorporeal circuits that replace the missing organ function such as hemodialysis machines or ventilators, and organ transplantation. Each of these approaches has its own disadvantages. Tissue engineering is an ideal approach for replacing the critical organ or tissue that is lacking, perhaps with the patient’s own cells.
In 1954, Dr Joseph Murray performed the first successful human kidney transplant, introducing the world to an entire new field of medicine.1 Organ and tissue transplant is a remarkable and life-saving option for many patients. Nevertheless, it is limited by donor scarcity, need for lifelong immunosuppression, and the risk of infection and rejection.2 For these reasons, tissue engineers are working toward developing methods of growing whole organs from a patient’s own cells or banked lines of cells that can be used to replace diseased and damaged tissues.
By definition, a tissue-engineered organ must be capable of performing the key functions of the replaced organ or tissue. Also, it should be durable, self-repairing, and match the longevity of the patient. It should not require the sacrifice or prioritization of other organs as is the case for operations such as gastric pull-up, vein harvest, or a myocutaneous flap. Tissue engineering strategies often employ overlapping techniques that can be applied to a number of organ systems. These approaches include: (1) a progenitor cell source; (2) a biological or synthetic scaffold for in vivo implantation; and (3) a method of expanding cell mass via in vitro cell culture, bioreactor, or in vivo implantation. Figure 79-1 illustrates a common approach for cell isolation, seeding of a scaffold, and implantation into the host. This general concept has been adapted to multiple organ systems and animal models.
FIGURE 79-1 A representative schematic of tissue-engineered technique. In the example above, murine tissue-engineered small intestine is generated. However similar techniques have been applied to many organ systems. Tissue is obtained for the isolation of a cellular source. These cells are used to seed a scaffold which is then implanted into a host. The tissue-engineered product is harvested after a period of growth.
Cell Source
Concurrent with the expansion of regenerative medicine and tissue engineering programs in the last decade, remarkable progress has been made in understanding stem and progenitor cells. Embryonic stem cells (ESC) are harvested from the inner cell mass of the blastocyst in embryos and are considered pluripotent cells, able to develop into any cell type except for the extra-embryonic tissues. This is advantageous because they are a potential source for any tissue in the body.3 However, work performed with ESC, particularly human ESC, is subject to considerable scrutiny and ethical concerns. Moreover, ESC may possess tumorigenic potential.4 Somatic or adult stem cells can be derived from the patient who requires the tissue-engineered organ, but lack totipotency. Instead, these cells are committed toward a particular lineage, but maintain the potential for self-renewal and possess multipotency, which is the ability to differentiate into multiple, but not all cell types.3 For example, the intestinal stem cell is capable of self-renewal and producing all differentiated cells of the intestinal epithelium, but it usually does not differentiate into a hepatocyte. A central dogma is that once a cell has differentiated, it is committed to that cell line.
In 2006, a method of reprogramming somatic cells to a pluripotent state was developed.5 These cells are termed induced pluripotent stem cells (iPSC). These cells overcome many of the ethical and immunologic concerns surrounding ESC; however, one disadvantage is that they maintain tumorigenic potential.4 Many of the current techniques for reprogramming these cells require the introduction of oncogenic viral vectors, thereby limiting clinical application.6 Additional sources of pluripotent cells include mesenchymal stem cells (MSC). MSC were initially described in 1968 as a population of bone marrow derived cells capable of osteogenic differentiation.7 Since then, MSC capable of self-renewal and differentiation have also been isolated from adipose, skin, blood, synovial membrane and amniotic fluid, and other mesodermally derived tissues.8
Scaffold
Many scaffold materials have been investigated for cell delivery. These can be divided into two broad categories: synthetic and biological. Both synthetic and biological scaffolds are designed to reproduce the properties of native mesenchymal support, sometimes with the associated extracellular matrix (ECM).
Synthetic scaffolds are designed to be porous enough to allow imbibition of nutrients until vasculogenesis and angiogenesis occurs, but also rigid enough to provide structural support. Frequently used materials include polyglycolic acid (PGA) and poly(L-lactic acid) (PLLA) that are biodegradable polymers designed to be hydrolyzed and absorbed by the recipient as the engineered product grows.9 With advancements in the field of biomaterials, synthetic scaffolds have become increasingly complex. Polyhedral oliogmericsilsesquioxane bonded to poly-[carbonate-urea] urethane (POSS-PCU), for example, is biocompatible, nonreactive, nontoxic, and can be designed to fit the patient’s needs. This scaffold has already been successfully used in a tracheobronchial transplant.9 The structure of some commonly used synthetic scaffolds are demonstrated in Figure 79-2.
FIGURE 79-2 Common synthetic scaffolds for in vivo tissue engineering. Highly porous structures are necessary to support tissue growth as demonstrated by scanning electron microscopy of (A) PGA-P(CL/LA) scaffold and (B) PLLA-P(CL/LA) scaffold. The scaffolds can be seeded with cells as demonstrated in (C) PGA-P(CL/LA) and (D) PLLA-P(CL/LA). These cells survive by imbibition until neovascularization occurs. The close approximation of cells and scaffold can be further demonstrated by staining with hematoxylin and eosin for both (E) PGA-P(CL/LA) and (F) PLLA-P(CL/LA) scaffolds. PGA, polyglycolic acid; PLLA, poly-L-lactic acid; P(CL/LA), copolymer sealant δ-caprolactone and L-lactide. (Adapted and reproduced with permission from Roh JD, Nelson GN, Brennan MP, et al. Small-diameter biodegradable scaffolds for functional vascular tissue engineering in the mouse model. Biomaterials 2008;29:1454–63.)
Biological scaffolds composed of naturally occurring macromolecules, in particular those that formulate the ECM, have also been described. These include porous collagen lattice, chitosan, glycosaminoglycans, silk fibroin, alginate, and starch.10 More recently, in an attempt to create more complex biological scaffolds, researchers have turned toward decellularized extracellular matrices as a potential material. Decellularization is a process in which organs are treated with detergents and enzymes to remove nuclear, cytoplasmic, and antigenic material while leaving behind the ECM (Figure 79-3). This methodology preserves the macroscopic and microscopic architecture of the original tissue, including vascular inflow and outflow, facilitating future vascular anastomosis and circulation.11 Subsequent recellularization is possible using an appropriate cell source.
FIGURE 79-3 Biological scaffolds derived from decellularized organs have been attempted. (A) Scanning electron microscopy (SEM) of native intestine. During decellurization, crypt/villus structure of the extracellular matrix (ECM) is preserved after (B) one cycle, but is lost (C) after four cycles. The collagen fiber network as it appears on SEM for (D) native intestine remains intact after (E) one cycle of decellurization, but is lost (F) after four cycles. A transmission electron micrograph (TEM) of (G) native intestine demonstrates normal cellularity. TEM confirms acellularity of the biological scaffold following (H) one and (I) four cycles of decellularization. (Adapted and reproduced with permission from Totonelli G, Maghsoudlou P, Garriboli M, et al. A rat decellularized small bowel scaffold that preserves villus-crypt architecture for intestinal regeneration. Biomaterials 2012;33:3401–12.)
Cell Expansion and Growth
A bioengineered organ must be of sufficient size to adequately support function. Methods for culturing cells include one or more of the following techniques. Many scientists have designed artificial bioreactors that attempt to mimic many of the physiologic processes that occur in an organism. These include control of pH, oxygen tension, temperature, waste removal, and even the pulsatile flow of blood.12 However, no bioreactor can fully recreate the complexity of a living animal. An alternative approach is to expand cells in vivo in what can be thought of as a living bioreactor. This has the advantage of encouraging the growth of blood vessels that will ultimately be needed to support the growing engineered tissue and access to circulating factors that may be important for cell growth. A marked disadvantage is the unpredictable number of variables introduced by the host, which increases the challenge of deciphering the cellular and molecular mechanisms of growing the tissue-engineered organ. The greatest challenge facing most tissue engineers is expanding the volume of tissue growth to a clinically relevant size while maintaining specific tissue functions with this growth.
Respiratory System
Trachea and Bronchi
Tissue-engineered trachea is conceptualized for the replacement of long-segment tracheal defects. Primary disease of the trachea, such as tracheal agenesis, is rare with an incidence of less than 1 in 50,000 live births.13 However, iatrogenic injury or malignant invasion may necessitate segmental resection. Primary repair is possible if the defect is less than 5 cm in adults and one-third the tracheal length in children.14 Although an allograft transplant is possible, it is limited by the lack of a suitable vascular pedicle and the probable need for lifelong immunosuppression.15 Prosthetic conduits have also been attempted. However, these are frequently complicated by product migration, infection, erosion, and disruption.14
Implantation of a tissue-engineered airway has been performed successfully in humans.16 The human donor trachea was decellularized to remove all cells and major histocompatibility complex (MHC) antigens. The recipient’s own epithelial cells and chondrocytes were then cultured and used to seed this biological scaffold. The bioengineered trachea was then anastomosed to replace the left main stem bronchus in a 30-year old woman with end-stage bronchomalacia. Follow-up 4 months later confirmed the patient was breathing normally without the need for immunosuppressive medications.
The first long-term follow-up for a tissue-engineered tracheal replacement in a child was published in 2012.17 The authors reported the case of a 12-year-old boy born with long-segment tracheal stenosis and a pulmonary sling. After years of failed interventions, including multiple stainless steel expandable stents and emergent repair of an aortotracheal fistula, he was the recipient of a tissue-engineered trachea. Prior to the operation, MSC from the patient were isolated and a cadaveric donor trachea was decellularized. At the time of the operation, the decellularized scaffold was seeded with MSC and patches of tracheal epithelium, and anastomosed to replace a 7 cm segment of stenotic trachea. In addition, the scaffold was treated intraoperatively with erythropoietin, granulocyte colony-stimulating factor, and transforming growth factor beta (TGF-β). These factors serve to enhance angiogenesis, improve recruitment of MSC, and induce chondrogenesis, respectively. During the immediate postoperative recovery, the child required stent placement. However, 2 years later, the child was breathing comfortably and had returned to school without the need for tracheal stenting. The graft had revascularized, demonstrating an epithelial lining, and appeared normal on computed tomography (CT) and ventilation-perfusion scan.
A limiting factor in the technique described above is the need for donor trachea that is subsequently decellularized. To avoid this need, synthetic scaffolds have also been used. In nonhuman studies, PGA was tubularized and coated with sheets of cultured MSC. MSC treated with glucocorticoids and TGF-β will differentiate along a chondrogenic lineage. These bioengineered, cartilaginous tubes closely resemble human trachea.18 Other cell sources and scaffold materials have also been tried including sheep nasal chondrocytes on a PGA and silicon tube matrix, or human nasal chondrocytes on hydrogel and high-density polyethylene.19,20 Although these methods are successful in generating a cartilaginous conduit, they have not been applied in humans.
Tissue-engineered lung parenchyma has posed a significantly greater challenge. Development of a tissue-engineered lung with fully differentiated pneumocytes and clara cells has not been achieved to date.21 Current methods are unable deliver cells in the appropriate cellular distribution and architecture to participate in normal gas exchange.
Cardiovascular System
Heart
The Centers for Disease Control (CDC) reports that 40,000 infants are born with congenital heart defects each year in the USA. Among adults, heart disease is the leading cause of death. The CDC estimates the annual financial burden of cardiovascular disease approximates $444 billion. Tissue engineering cardiac replacements such as myocardial tissue and heart valves may help alleviate some of these costs. Murine ESC can be expanded in culture and differentiated into cardiomyocytes.22 Similar results have been shown with human cells.23 Ethical concerns have limited the transition to human experiments prompting increased interest in iPSC24 which can be driven towards a cardiac lineage after reprogramming.24,25
In 2001, implantation of autologous skeletal myoblasts into scar tissue was performed on a patient during coronary artery bypass grafting. Echocardiography performed five months after the operation demonstrated improved areas of myocardial contractility within the scar.26 However, in a randomized placebo-controlled study performed on 94 patients, these results could not demonstrate an improvement in heart function, partly due to the development of arrhythmic areas.27 Aiming to improve delivery and distribution of cells, laboratories are looking into seeding myoblasts on a polyurethane scaffold prior to implantation. In a rat model of myocardial infarction, such an approach prevented progression to heart failure more effectively than sham operation or unloaded scaffold implantation.28
Heart Valves
Nearly one-third of congenital heart defects involve the aortic or pulmonary valves, with current methods of valve replacement not providing optimal therapeutic solutions. Mechanical prosthetic valves are prone to thrombus and require long-term anticoagulation, increasing the risk of hemorrhage. Bovine and porcine bioprostheses may fail secondary to calcification or structural damage. All prosthetics valves can be complicated by infection or paravalvular leak. For the pediatric population, the failure of replacement valves to grow with the patient requires multiple operations into adulthood. Tissue-engineered heart valves (TEHV) offer the hypothetical advantage of autologous growth, self-repair, and remodeling with the patient.
Native heart valves have three layers, namely: fibrosa (interstitial cells and collagen), spongiosa (proteoglycans), and ventricularis (elastin sheets).29 These valves possess remarkable mechanical durability, capable of opening and closing three billion times over a lifespan.30 Decellularized porcine valves, fibrin, collagen-rich material, and PLLA have been used as scaffolds. Cells for TEHV have generally been applied by one of two methods: either directly seeded on the scaffold prior to in situ implantation, or subsequent attachment of endothelial cells from the blood stream after implantation of an empty scaffold. Bone marrow MSC, umbilical blood progenitor cells, and circulating endothelial progenitor cells have served as possible cell sources.
Expression of the glycoprotein antigen CD133 has been used as a marker for progenitor cells, and is important for the identification and isolation of hematopoietic stem cells.31 Translation to human use has already been demonstrated. Dohmen et al produced TEHV by combining cadaveric human decellularized pulmonary heart valve scaffolds that were seeded with autologous vascular endothelial cells and were implanted into 11 patients.32 At ten-year follow-up, all patients were alive with no regurgitation or accelerated velocities on transthoracic echocardiography and no structural defects on CT. These are encouraging results for the future of TEHV, but require additional validation prior to becoming standard of care.33
Blood Vessels
Vascular bypass is frequently needed as an organ preserving and life-saving operation. Autologous venous and arterial grafts are preferred over synthetic materials, but necessitate the sacrifice of the donor vessel.34 Synthetic materials are prone to infection and maintain patency rates of only 38–49% at 5 years.35 Similar to synthetic valves, synthetic blood vessels do not increase in size with a growing child. In the mid-1980s, Weinberg and Bell ushered in the dawn of tissue-engineered vascular grafts (TEVG; Fig. 79-4) when they seeded endothelial cells onto a scaffold made from bovine smooth muscle cells, cultured fibroblasts, and Dacron mesh.36 Although these grafts failed at pressures greater than 180 mmHg, this research demonstrated that generation of a TEVG is possible. Standards for producing TEVG dictate that durable replacements should have a burst pressure greater than 2000 mmHg.37,38 Since then, multiple scaffolds have been developed, including PGA and decellularized allograft vessels, that are capable of withstanding burst pressures upwards of 2100 mmHg.34
FIGURE 79-4 Treatment of human disease with TEVG. Angiography confirms that there were no stenotic, aneurysmal, or calcified lesions at (A) 5 years and (B) 4 years post implantation for these two patients. (Adapted and reproduced with permission from Hibino N, McGillicuddy E, et al. Late-term results of tissue-engineered vascular grafts in humans. The Journal of Thoracic and Cardiovasc Surg 2010;139:431–6.)
In 2001, the first TEVG was transplanted into a 4-year-old girl to replace her right intermediate pulmonary artery that had occluded following a Fontan procedure.39 A peripheral vein was harvested providing cells for culture. Once cultured to 12 × 106, they were seeded on a 2 cm long polycaprolactone-polylactic acid and PGA scaffold. This group performed a similar procedure on two additional patients, before changing the cell source to aspirated bone marrow cells. At 5.5 years of follow-up, all 25 patients were alive and there was no evidence of aneurysm, rupture, infection, or calcification. Four patients were successfully treated percutaneously for stenosis, and one patient required anticoagulation for a partial thrombus.40 Currently, another group of surgeons are conducting a phase I clinical trial for the implantation of TEVG.41 Based on these results, it is possible that TEVG will become a component in the management algorithm for the routine treatment of vascular disease in the near future.
Alimentary System
Esophagus
Esophageal lengthening via mobilization, circular myotomy, or a staged approach may close gaps up to 3 cm in babies with isolated esophageal atresia. Beyond this distance, an esophageal replacement is often needed.42 An adequate replacement conduit can be constructed via gastric pull-up or the interposition of colon or jejunum, but these approaches rely on prioritizing and sacrificing an area of the gastrointestinal tract.43–45 Tissue-engineered esophagus (TEE) has been successful in rat and dog esophageal replacement models.46,47 In dogs, an amniotic membrane was cultured with oral keratinocytes and fibroblasts. These cultured cells were fashioned into a tube on a PGA scaffold and implanted with smooth muscle tissue into the omentum of the host dog. Then, at 3 weeks, the TEE was transferred as a pedicle graft to close a 3 cm esophageal gap. More than a year later, dogs who underwent this operation were alive and healthy. Although peristalsis of the TEE segment was not evident on imaging, both solids and liquids were able to pass easily from mouth to stomach.
An earlier study in rats isolated esophageal organoid units (OU) for the production of TEE. OU are cellular constructs containing all cells necessary to generate full-thickness tissue such as progenitor cells, supportive mesenchymal cells, and differentiated epithelial cells. OUs were loaded onto a PGA/PLLA scaffold and implanted into the omentum of host rats. After 4 weeks of growth, the TEE was either harvested for histology or anastomosed as an interposition graft (n = 3) or onlay patch (n = 3). Both the interposition and patch groups had one mortality and two survivors. The surviving animals in both groups gained weight and possessed a patent TEE when evaluated with fluoroscopy. Histologic evaluation confirmed the presence of a stratified squamous epithelial lining similar to native esophagus.46
A similar technique has been demonstrated in a sheep model. The omental implantation of ovine esophageal epithelial cells seeded onto a tube of collagen produced TEE with areas of normal appearing esophageal epithelium. During the proliferative phase, the lumen was maintained by a stent that was removed at harvest. These constructs also demonstrated vascular ingrowth, a necessary feature for successful in vivo tissue engineering.48,49 A variety of cellular sources as well as synthetic and biological scaffold materials have been tested for TEE. In one study, various cell and scaffold arrangements were evaluated. It was found that human esophageal squamous cells and a porcine esophageal matrix scaffold grew TEE that most closely and reliably reproduced normal appearing esophageal morphology.50
Stomach
Conditions requiring total gastrectomy such as gastric volvulus with necrosis and gastric cancer are rare in children. However, among adults, gastric cancer is the second leading cause of cancer mortality in the world and a common indication for gastric resection.51
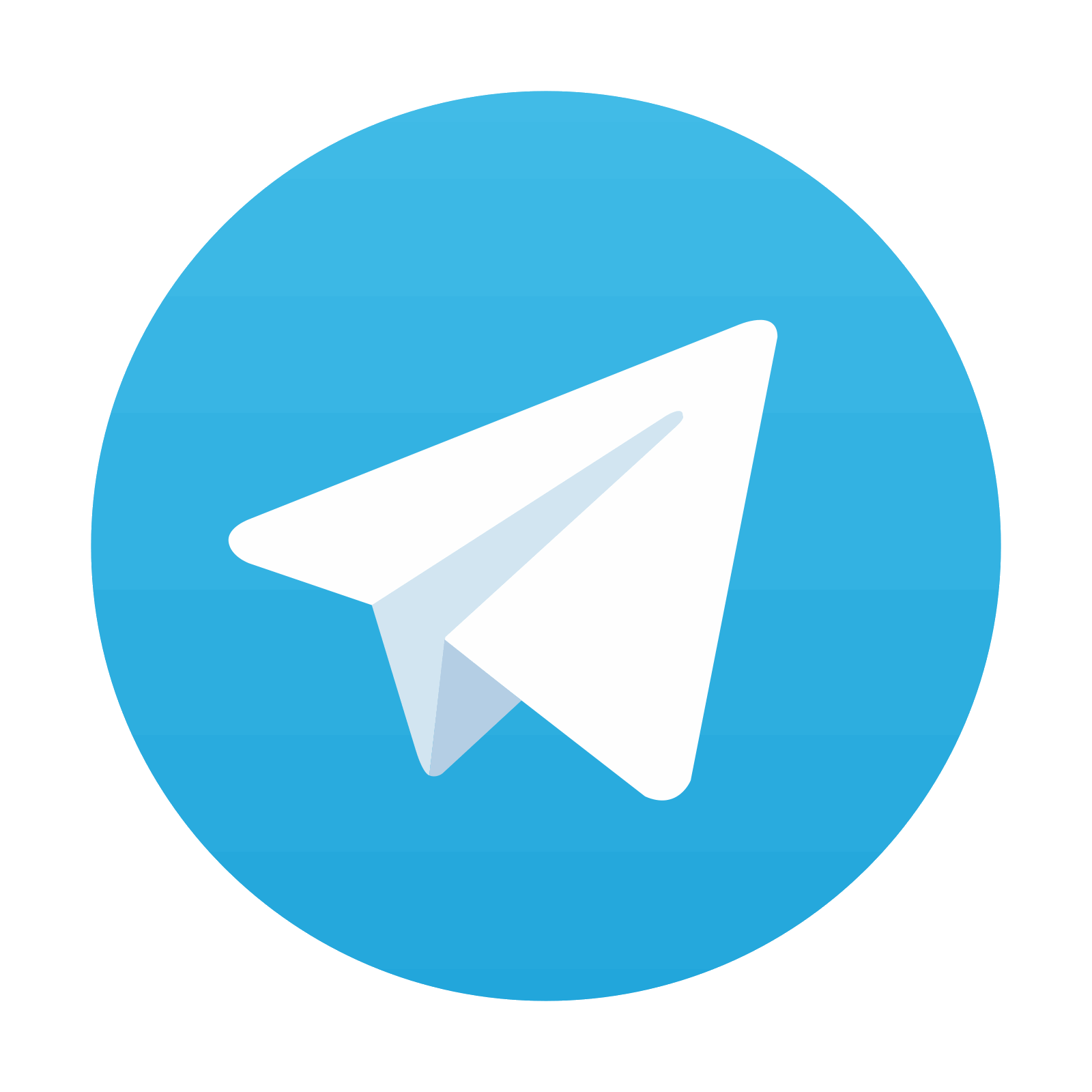
Stay updated, free articles. Join our Telegram channel
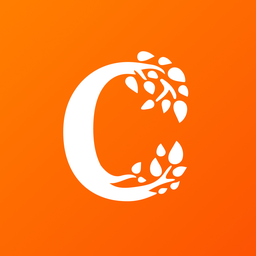
Full access? Get Clinical Tree
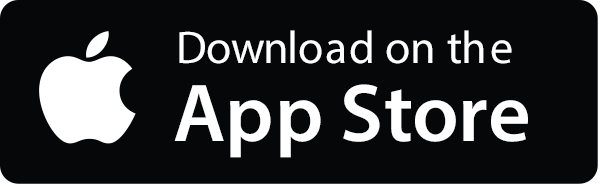
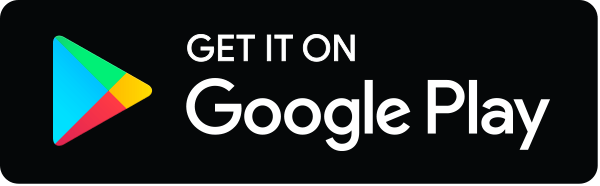