, Francis Leclerc2, Véronique Nève3, J. Jane Pillow4, 5 and Paul D. Robinson6, 7
(1)
Division of Sleep Medicine, Department of Internal Medicine, Department of Molecular Pharmacology and Physiology, and Department of Pediatrics, University of South Florida, Tampa, FL 33612, USA
(2)
Réanimation Pédiatrique, CH & U de Lille and Université Lille Nord de, Lille, France
(3)
Explorations Fonctionnelles Respiratoires, CH & U de Lille, Lille, France
(4)
School of Anatomy, Physiology and Human Biology and Centre for Neonatal Research and Education, The University of Western Australia, 35 Stirling Highway, Crawley, 6009, Western Australia
(5)
Consultant Neonatologist, King Edward Memorial Hospital, 374 Bagot Road, Subiaco, 6008, Western Australia
(6)
Department of Respiratory Medicine, The Children’s Hospital at Westmead, Locked Bag 4001, Westmead, NSW, 2145, Australia
(7)
The Discipline of Paediatrics and Child Health, The University of Sydney, Sydney, Australia
4.1 Physiology of the Upper Airway and Control in Breathing
Alastair A. Hutchison8
(8)
Division of Sleep Medicine, Department of Internal Medicine, Department of Molecular Pharmacology and Physiology, and Department of Pediatrics, University of South Florida, Tampa, FL 33612, USA
Educational Aims
-
Describe the expanded concept of breathing in terms of multiple motor functions that ensure airway protection, volume homeostasis, and ventilation.
-
Describe the upper airway structures and how their functions affect the entire airway.
-
Describe the nasal functions involved in air conditioning, in protection, in the maintenance of airway patency, and in the consequences of nasal bypass and obstruction.
-
Describe the oral cavity and pharyngeal regions and control of airway patency.
-
Describe factors involved in obstructive sleep apnea and the effects of CPAP therapy.
-
Describe the coordination of sucking, nutritive swallowing, and breathing during development and the relationship to apnea and bradycardia.
-
Describe the importance of nonnutritive swallowing in pharyngeal clearance and how this may be influenced by CPAP.
-
Describe the three major functions of the larynx involving breathing.
-
Describe the triple mechanism of laryngeal closure in airway protection.
-
Describe how flow and subglottic volume are controlled during eupnea.
-
Describe the integrative nature of the central control of laryngeal functions.
-
Describe the roles of laryngeal muscles and their coordination with pump muscles during development and in eupnea, sighs, grunting, incremental breathing, and gasping.
-
Describe how this information has been/can be applied in respiratory support.
-
Describe afferent inputs that alter laryngeal muscle functions and alter breathing and cardiovascular function.
-
Describe the impact of central behavioral state and induced central depression upon laryngeal function and the interactions with detected changes in the gaseous environment.
4.1.1 Introduction: Breathing and the Upper Airway
It is impossible for anyone to find the correct function of a part unless he is perfectly acquainted with the action of the whole instrument. Galen, AD 120–200.
Breathing consists of multiple motor acts whose mechanical effects aim to ensure that the airway is protected, has optimal supra- and subglottic volumes, and provides vital ventilation. The upper airway extends from the nose or mouth to the larynx (Seikel et al. 2005), but its functions, in the context of ventilation, extend to the entire airway. Maintenance of airway volume and ventilation is actively controlled by the centrally coordinated activities of nasal, oral, pharyngeal, and laryngeal upper airway muscles and those of the pump muscles. These act in concert with lower airway smooth muscle. Effective breathing requires that the brain coordinates ventilatory functions with cardiovascular function and with other motor acts, e.g., swallowing, postural changes, and speech. Adaptations in breathing must occur appropriately, rapidly, and constantly. Protective and mechanical changes occur in milliseconds. Changes in circulatory gas transport alter breathing within seconds. Other adjustments optimize breathing in tune with changes in growth, aging, metabolism, and the environment. This chapter addresses how each upper airway structure plays critical roles in the dynamic processes of breathing.
4.1.2 Nasal Functions in Breathing
… we would do well to keep our mouths shut and reflect on the marvelous physiology of the nose. Richard Godfrey 1994
4.1.2.1 Air Conditioning and Vascularity
Normal breathing occurs via the nose (Fig. 4.1). Inspired and expired air is conditioned by the nasal mucosa, whose surface area and blood supply are such that particles are filtered and temperature and humidity are modified. The nasal vascular system can achieve a 25 °C gradient between the nasal ostia and the nasopharynx (Godfrey 1994), a property that enables air breathing at very different environmental temperatures while maintaining a remarkable constancy of temperature and humidity in the lower airway, thereby optimizing cellular function and ciliary action. Given its vascularity, it is not surprising that the nose is a source of nitric oxide, that trauma to the nasal passages can be induced by nasal continuous positive airway pressure (NCPAP) prongs and other cannulae, and that epistaxis can require hospital admission.
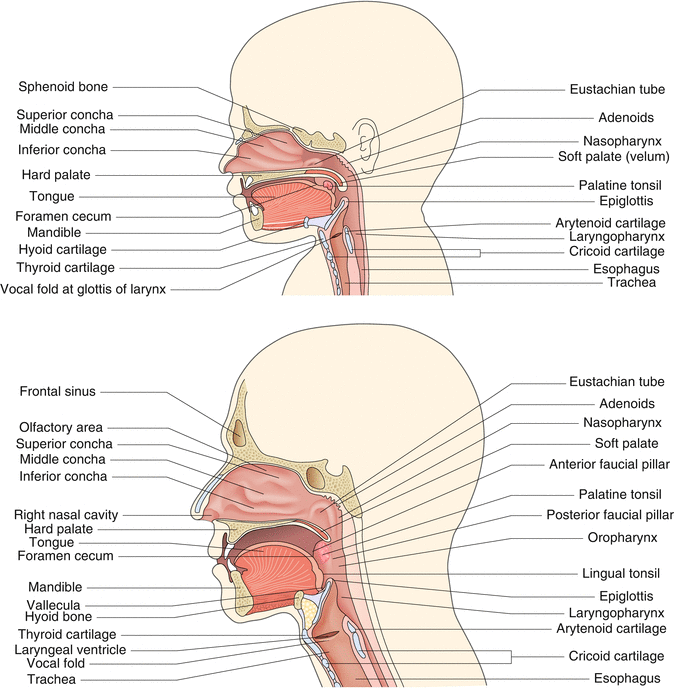
Fig. 4.1
The anatomy of the newborn (left panel) and adult (right panel) upper airways is shown
4.1.2.2 Nasal Reflexes and Protection
The nose can sniff out noxious substances and protect the airway from particles by sneezing. It can increase its resistance rapidly, switching on a watery secretion “like a tap” (Godfrey 1994), and can trigger laryngeal closure and reduced ventilatory drive (Editorial 1992). The extreme protective reflex response to nasal irritation is the dive reflex that is typified by apnea with glottic closure, bradycardia, and redistribution of blood flow to the brain, heart, and adrenals (see also Sect. 47.3.1) (de Burgh Daly 1997; Editorial 1992). The dive reflex can be triggered by nasal water, tobacco smoke, or ice applied to the trigeminal area (Angell James and de Burgh Daly 1969; de Burgh Daly 1997). Water entry into the nose is dramatic even for adults who “suffer a sense of impending suffocation and the almost impossibility of voluntarily making an inspiratory effort” (de Burgh Daly 1997). Exaggerated dive reflex responses can cause total upper airway closure and cardiac arrest and have been implicated in accidental and deliberately induced deaths (de Burgh Daly 1997).
4.1.2.3 Nasal Patency and Resistance
Nasal breathing in the newborn and infant is the predominant but not obligatory form of breathing and is related to the high position of the epiglottis (Fig. 4.1) (Seikel et al. 2005). There is a nasal cycle whereby resistance alternates from one nostril to the other every 2–4 h in 80 % of humans (Widdicombe 1986). Pressure in the axilla or on the lateral chest increases ipsilateral nasal resistance (Widdicombe 1986). Resistance is highest in the regions adjacent to the external ostia, whose dimensions are altered by the alae nasi muscles that cause nasal flaring during exercise hyperpnea and respiratory distress, especially in newborns. Newborn nasal resistance exceeds that of the adult in absolute terms but is less than that of their lower airways. Nearly half of the adult’s total airway resistance is nasal. Thus, minor increases in nasal resistance can produce major overall effects on breathing (Editorial 1992). In adult humans, nasal occlusion results in a switch to mouth breathing in <5 s (Editorial 1992). Both this switch to oral breathing and the resumption of nasal breathing following release of the occlusion are delayed by nasal and pharyngeal anesthesia. Occlusion of the nasal passages in infants results in mouth breathing in ~8 s (range <1–32 s) (Editorial 1992; Rodenstein 2004). The response is quicker with advancing age but is decreased in rapid eye movement (REM) sleep, more so at 6 weeks than in the newborn period (Editorial 1992). These observations led to a proposal that the response may be related to the sudden infant death syndrome (SIDS) (Editorial 1992). In premature infants, the change to oral breathing results in a sixfold increase in pulmonary resistance, which over time might result in fatigue with hypoventilation (Miller et al. 1987). Oral breathing places an increased burden on the lower airway to modify the temperature and humidity of inspired gas, presumably via changes in lower airway vascularity. Preventing nasal breathing can decrease gas exchange and functional residual capacity (FRC) (Editorial 1992). Postoperative nasal packing in adults can lead to arterial hypoxemia, apnea, and sleep disturbance, with the latter also occurring in normal volunteers after local anesthesia of the nasal passages (Editorial 1992). In newborn lambs, nasal obstruction also affects gas exchange, with hypoxemia, hypercapnia, and acidosis being observed. These effects are aggravated by carotid body denervation. The impact of nasal obstruction diminishes with advancing age (Editorial 1992). Blockage of the nose (choanal stenosis being an extreme example) can cause obstructive sleep apnea (OSA) (Marcus 2000). Human neonates whose nasal resistance is increased by birth trauma or trauma secondary to repeated suctioning breathe orally. They can have cyanotic episodes, stridor, and hypercapnia; these problems disappear with resolution of the nasal injury (Miller et al. 1987).
In summary, the nose provides air conditioning, and its sensory receptors initiate reflexes that alter upper airway patency and mediate extended ventilatory changes in response to a challenge.
4.1.3 Oral Cavity and Pharyngeal Functions in Breathing
…after relaxing, many sword swallowers are able to relax and breathe while swallowing a sword. From swordswallow.com 2009.
The oral cavity extends from the lips to the plane of the faucial pillars, where it joins the pharynx (Fig. 4.1). The three pharyngeal regions are the nasopharynx, from the posterior nares to the uvula; the oropharynx, from the uvula to the epiglottis; and the laryngopharynx, from the epiglottis to the esophagus. The pharynx is a conduit for air and/or food.
4.1.3.1 Oral Cavity and Pharyngeal Patencies and Obstructive Apnea
The muscles of the tongue, especially the genioglossi, maintain patency of the oral cavity. Pharyngeal patency is a function of the cavity dimensions (Rodenstein 2004) and the balance between the opening forces exerted by pharyngeal muscle activities and the collapsing ones exerted by the tissue–intrapharyngeal pressure gradient and by pharyngeal mucosal adhesion (Fig. 4.2). Patency is critically influenced by posture. Narrowing occurs with neck flexion and hyperextension and can alter cavity pressures required to achieve flow. Narrowing can also follow an inspiratory occlusion of the mouth/nose (a load) that augments diaphragmatic activity and thus negative pressure within the pharynx. Upper and lower airway afferents induce reflex compensatory change in glossopharyngeal muscles to offset the load effect (Fig. 4.2) (Bailey and Fregosi 2006).
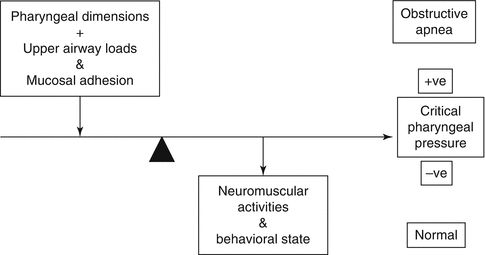
Fig. 4.2
This diagram shows the factors that determine pharyngeal patency – please see text (Modified from Patil et al. (2007))
Obstructive apnea occurs during sleep in ~1–4 % of children and adults (Marcus 2000). In children, a persistent partial form of upper airway obstruction, obstructive hypoventilation, is seen (Marcus 2000). In adults, a spectrum of decreased inspiratory airflow is seen in snoring, upper airway resistance syndrome, and OSA. Pharyngeal OSA occurs when a critical tissue pressure (P crit) exceeds the intrapharyngeal opening pressure (Fig. 4.2) (Marcus 2000; Dempsey et al. 2010) and is influenced by factors that alter pharyngeal dimensions, namely, adenotonsillar hypertrophy, obesity (less common in children than adults), low subglottic airway volume, and craniofacial structural abnormalities. The major role played by the central motor output to the oral cavity and pharyngeal muscles that modify P crit is emphasized by the fact that OSA occurs only in sleep, predominantly in REM sleep in children (Marcus 2000). The following factors also stress the importance of central control in OSA. Obstructive apnea occurring during mixed apnea in preterm newborns involves a direct central mechanism, as shown by the onset of pharyngeal and/or laryngeal closure before diaphragmatic activation (Idiong et al. 1998) (see Sect. 47.3.1). In adults pharyngeal closure can accompany central apnea (Marcus 2000). Central arousal patterns during OSA differ between children and adults (Marcus 2000). Other factors affecting the incidence of OSA are gender, a familial tendency, and racial/ethnic factors (Marcus 2000). Anti-inflammatory medications can benefit OSA, suggesting a role for inflammation in its etiology (Praud and Dorion 2008). Therapy with NCPAP improves OSA by increasing intrapharyngeal pressure and its transverse diameter (Rodenstein 2004), by augmenting lower airway volume and probably by stimulating breathing via pharyngeal pressure sensors (Angell James and de Burgh Daly 1969). In children and adults with OSA, CPAP therapy can improve their metabolism.
4.1.3.2 Sucking, Nutritive Swallowing, and Breathing
The abilities to suck, swallow, and breathe are developed during fetal life (Harding 1986). At all ages, swallowing is accompanied by laryngeal closure. Term newborns and infants up to 12–18 months of age can lock the larynx into the nasopharynx (Figs. 4.1 and 4.3). This separation of the nasopharyngeal–laryngeal air passage from the oropharyngeal nutritive passage enables the term infant to breathe and feed simultaneously but with decreased ventilatory drive. This ability is not developed in the preterm neonate whose sucking pattern is also immature (Lau 2006). Coordination of sucking–swallowing–breathing is defined by the ability to feed “by mouth with no overt signs of aspiration, oxygen desaturation, apnea, or bradycardia” and when “a ratio of 1:1:1 or 2:2:1 suck: swallow: breathe” is attained (Lau 2006). Safe swallowing occurs at end-expiration and end-inspiration (Lau 2006). In term newborns, 55 % of swallows occur at these points of the breathing cycle. In preterm infants, 55 % of swallows occur during deglutition apnea (Lau 2006).
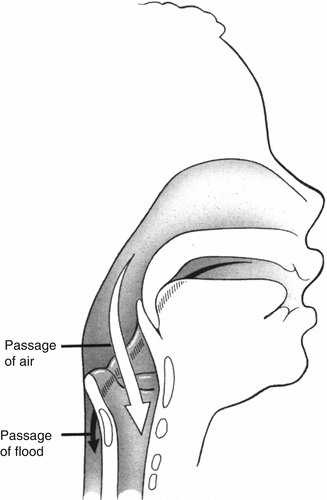
Fig. 4.3
The high position of the infantile larynx allows the epiglottis and uvula to lock, separating the nasopharyngeal–laryngeal air passage from the oropharyngeal nutritive one (From O’Connor, D. Reproduced with permission from Myer et al. (1995). Redrawn by agreement with Springer-Verlag)
4.1.3.3 Nonnutritive Swallowing, Aspiration, and Swallow Breaths
Nonnutritive swallowing (NNS) is “essential for survival,” since >2 L of oral and nasal secretions are produced daily. Without NNS, “the lungs rapidly fill with these secretions, producing death within a few days” (Thach 2005). When pharyngeal secretions reach a critical volume, neural receptors, in the interarytenoid notch, stimulate the laryngochemoreflex which triggers NNS (Thach 2005). The critical pharyngeal volume for NNS may be increased by applied positive pharyngeal pressure, providing an explanation for the decrease in NNS frequency with CPAP (Thach 2005). In adult humans NNS occurs mainly during expiration. In infants NNS occurs at any time in the respiratory cycle (Thach 2005). Volitional swallowing in adults clears the pharynx and reduces the protective laryngeal adductor reflex. During sleep, the protection provided by NNS and glottic closure is imperfect, and some aspiration is common in normal individuals (Thach 2005). Swallow breaths are short inspirations that precede a swallow, occurring when the upper pharyngeal sphincters are closed (Thach 2005). These breaths are thought to result in pharyngeal air being inhaled as opposed to being swallowed (Thach 2005). The increased gastric air found when positive pharyngeal pressure is applied suggests that CPAP can alter normal pharyngeal clearance mechanisms and infers the need to titrate the applied pressure carefully (Thach 2005). Finally, swallowing may be related to sleep apnea and be part of recovery from sleep apnea (see Sect. 47.3.1) (Thach 2005).
In summary, patency of the oral cavity and the pharynx and the coordination with swallowing and sucking are critical for breathing. The term, but not preterm, newborn can suck and breathe simultaneously, although ventilatory drive is decreased. Physiological control of breathing and swallowing involves important sensory input that limits aspiration and protects the lower airway. Intensive care therapies can alter normal protective, breathing and swallowing functions.
4.1.4 Laryngeal Functions in Breathing and Control in Breathing
Like a Swiss watch, …our vocal tract depends on the precise functioning of many structures and muscles.” Jared Diamond, 1992 (The Third Chimpanzee).
The larynx has three major functions involving breathing. It serves as a protective structure guarding the subglottic airway; as a flow controller of subglottic absolute and tidal volumes during eupnea and complex coordinated movements; and as a vibratory modulator of flow that generates speech, song, and laughter (Fig. 4.4).
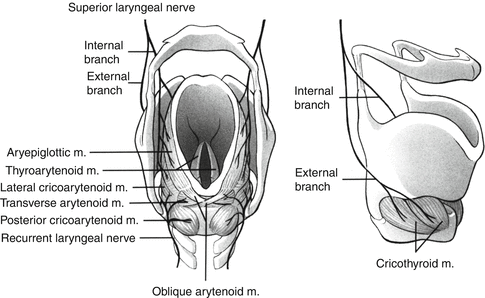
Fig. 4.4
The intrinsic laryngeal muscles and their innervation are shown. The left panel shows from bottom to top the posterior cricoarytenoids, the sole abductors of the larynx, and the abductors: the thyroarytenoids, the lateral cricoarytenoids, the transverse and oblique arytenoids, and the aryepiglottic muscles. All of these muscles are supplied by the recurrent laryngeal nerve (X). The internal branch of the SLN is sensory, while the external SLN branch is motor for the cricothyroid muscles (right panel) (From O’Connor, D. Reproduced with permission from Myer et al. (1995). Redrawn by agreement with Springer-Verlag)
4.1.4.1 Laryngeal Opening and Closure in Airway Protection and Breathing
4.1.4.1.1 Laryngeal Closure and Airway Protection
Protection of the subglottic airway is a centrally controlled major task of “breathing.” Laryngeal stimulation can trigger a clearing response, e.g., an expiration reflex or a cough, or can result in airway closure. Laryngeal closure occurs at the level of the epiglottis, at the vestibular folds, and at the vocal folds. This triple mechanism has been likened to closure of a nutcracker, an analogy whose accuracy is all too evident to intensivists faced with laryngospasm (Fink 1973).
4.1.4.1.2 Eupnea: Glottic Aperture Size and Flow/Volume Control
In adult humans the glottis opens maximally during inspiration, closes gradually to a minimum about three quarters through expiration, and then opens again in late expiration (England et al. 1982a). Glottic resistance is least at peak inspiration, increases as expiration progresses, and then decreases in late expiration to minimize inspiratory flow resistance with the onset of pump muscle activity (England et al. 1982a). There is no sensation of expiratory glottic closure in eupnea. Accurate, rapid laryngeal opening and closure maintains an optimal subglottic airway volume, ensures tidal volume changes that enable gas exchange, and enhances airway patency and surfactant secretion. The smooth tidal flow pattern, produced by laryngeal and diaphragmatic activities interacting with the mechanics of the respiratory system, avoids tissue exposure to acceleration or deceleration injuries.
4.1.4.1.3 Integrative Central Control and Effects
The same laryngeal intrinsic muscles are involved in all laryngeal functions emphasizing that the central coordinations of the motor acts of breathing are truly integrative, a fact also stressed by the impacts that laryngeal sensory inputs and the resultant vagal outputs have on the cardiovascular system. Laryngeal closure is important for other motor acts, e.g., effective lifting. Central control of breathing is linked to emotions. It is of note that in laughter the order of abductor–adductor activities seen in breathing is reversed (Luschei et al. 2006). Eastern philosophies combine breathing and vocalizations in practices aiming to augment metabolic and mental well-being.
4.1.4.2 Laryngeal Intrinsic Muscles and Control in Breathing
Understanding the actions of the laryngeal intrinsic muscles is a necessary first step in gaining insights into the nature of the central control of breathing. The posterior cricoarytenoids (PCA) are the sole abductors of the vocal cords. There are several adductors, with the thyroarytenoids (TA) being the major ones (Fig. 4.4). Some adductors, the cricothyroid and the vocalis part of TA, enhance abduction by tensing the vocal cords. This restricts vertical movement, improving flow control in the more horizontal plane. The magnitude of abductor activity exceeds that of the adductors when glottic size is increased and vice versa, consistent with relationships between glottic size and flow resistance (England et al. 1982a; Insalaco et al. 1990; Kuna et al. 1988). Animal studies indicate that, in general, glottic opening and closing are achieved by reciprocal laryngeal abductor and adductor activities (Fig. 4.5) (Bartlett 1989; Harding 1986; Hutchison et al. 1993). Concurrent actions of laryngeal abductor and adductor muscles during inspiration and expiration may occur in adult humans, but their nature is unknown (Insalaco et al. 1990; Kuna et al. 1988, 1990).
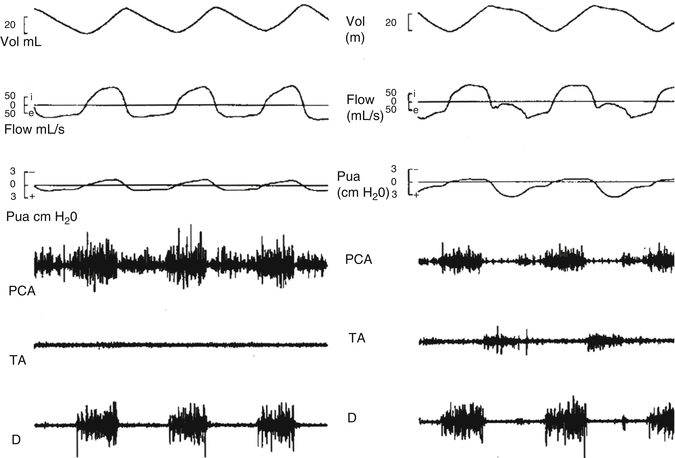
Fig. 4.5
This figure demonstrates the breathing cycles during eupnea and grunting in newborn lambs, showing the relationships between the EMGs of the posterior cricoarytenoid (PCA) muscles, those of the major adductors, the thyroarytenoid (TA) muscles, and those of the diaphragm (D), together with flow, volume, and trans-upper airway pressure changes (Reproduced with permission from Hutchison et al. (1993))
4.1.4.2.1 Laryngeal Intrinsic Muscles in Eupnea and Grunting
During eupnea the inspiratory outline of the PCA EMG resembles the flow pattern, while the ramp shape of the diaphragm EMG relates to the inspired volume trace (Hutchison et al. 1993). In animals little expiratory TA activity is seen, and trans-upper airway pressure is minimal (Fig.4.5). In eupneic expiration in adult humans, PCA activity diminishes, but some TA activity is present “rounding off” the early expiratory volume trace, but without the flow retardation or trans-upper airway resistance patterns typical of grunting (Kuna et al. 1988) (Fig. 4.5). Time delays exist between EMG and mechanical flow changes (Hutchison et al. 1993). The timing delays differ in eupnea and grunting, reflecting different mechanoreceptor feedbacks and central outputs that affect function (Fig. 4.5) (Hutchison et al. 1993).
Clinically, grunting is the expiratory noise produced when air flows through a partially closed glottis (Harrison et al. 1968). Total glottic closure is silent but sensed. Physiologically, grunting is a breathing pattern seen throughout life. It consists of a spectrum of degrees and timings of expiratory laryngeal closure, associated with volume retention and increased subglottic pressure, followed by laryngeal opening with a rapid return to baseline end-expiratory volume (EEV) (Figs. 4.5 and 4.6) (see Sect. 47.3.1) (Hutchison et al. 1993). In newborns with respiratory distress, Harrison et al. showed that when grunting was prevented by endotracheal intubation, without positive end-expiratory pressure (PEEP), their oxygenation worsened (Harrison et al. 1968). This finding established the role of airway PEEP and likely influenced the introduction of CPAP therapy. Harrison et al. also focused on venous return (Harrison et al. 1968), stressing the importance of cardiac function in grunting. The outline of the volume–time trace in grunting (Figs. 4.5 and 4.6) resembles that of a volume with a prolonged inspiratory time, as used in artificial ventilation to improve oxygenation. Pre-surfactant, this strategy reduced the required peak inspiratory pressure and decreased the incidence of bronchopulmonary dysplasia. Today, the maintenance of subglottic volume, the “open lung” approach, is a fundamental tenet of all ventilatory support. In keeping with the concept of alveolar interdependence, airway volume recruitment is achieved not by a maximal prolonged inflation but with an augmented breath (sigh) followed by an expiratory hold, mimicking the volume–time profile of grunting or of airway pressure release ventilation. Spontaneous sighs can be biphasic, with inspiration being interrupted briefly by laryngeal closure. This mechanism may reset vagal afferent feedback, avoiding a Hering–Breuer inflation reflex and possible loss of the acquired volume (see Sect. 47.3.1). In summary, human and animal data emphasize the importance of laryngeal control of expiratory subglottic airway volume. This central control strategy whereby airway pressure is dependent upon volume control has been shown by the study of bubble physics to produce a more stable mechanical system (Hildebrandt 1974). Thus, coordinated central control of laryngeal and pump muscles is a major focus in the control of breathing. This knowledge can be applied in respiratory support (Hutchison and Bignall 2008).
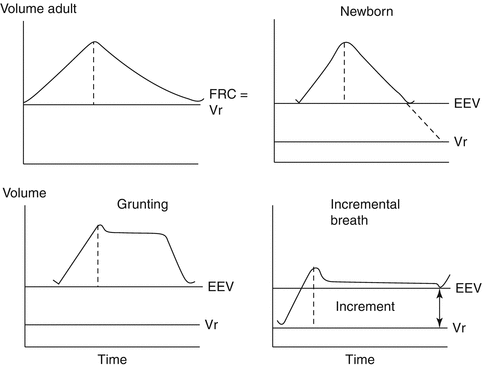
Fig. 4.6
Volume–time diagrams of adult and neonatal lung volumes are shown. The dashed line in each tidal volume separates inspiration from expiration. At rest (relaxation), the end-expiratory lung volume (EEV) of the adult (top left) is termed the functional residual capacity (FRC). It is a relaxation volume (V r). In the newborn (top right) the V r is low in comparison with that of the adult. The neonate actively maintains an EEV above V r. The grunting breathing pattern (bottom left) maintains lung volume above the EEV for variable periods of expiration before the volume returns to the EEV. The incremental pattern of breathing (bottom right) maintains lung volume above the EEV throughout expiration and, using laryngeal and diaphragmatic activities, achieves a true increment in lung volume (Reproduced with permission from Hutchison and Bignall (2008))
4.1.4.3 Laryngeal and Pump Muscle Breathing Patterns in the Fetus and Newborn
Behavioral state is a major controlling factor of breathing pattern. In fetal lambs, during the high-voltage electrocorticogram (ECoG) state, apnea (no phasic laryngeal abductor or diaphragmatic activities) and tonic laryngeal adductor activity occur. Laryngeal adductor activity can be enhanced by laryngeal contact with cooled lung liquid, by fetal movements, by “arousals,” by swallowing, by uterine contractures, and by fetal hypoxemia. In the low-voltage ECoG state, laryngeal and diaphragmatic activities are similar to those seen postnatally (Harding 1986). Hypercapnia mainly enhances fetal breathing in the low-voltage ECoG state (Harding 1986). In general, active laryngeal retardation of lung liquid egress is reported in the absence of fetal breathing movements and is related to lung growth (Harding 1986). Stimulation of the superior laryngeal nerve (SLN) produces laryngeal closure (Harding 1986) that will prevent aspiration of noxious material, e.g., meconium. The coordination of the acts of fetal swallowing (Harding 1986) and “inspiratory” activities of the respiratory muscles stresses the developmental importance of the controller’s ability to switch patterns rapidly between upper airway closure (laryngeal adduction) with lack of diaphragmatic activity (apnea) and laryngeal abductor and pump activities (see Sect. 47.3.1).
Airway volume is high in the fetus compared to the newborn. It has been speculated that during different fetal behavioral states, the brain is setting the homeostatic limits for high and low airway volume to prepare the fetus for the major needs of subglottic volume control at birth (Hutchison 2007) (see also Sect. 47.3.1). Life’s greatest airway volume challenge, the establishment and maintenance of an air-filled airway at birth, is usually overcome without a hitch by breathing patterns whose complexities exceed those used during the majority of adult life. At birth, an incremental breathing pattern retains more inspired volume than is expired (Fig. 4.6) (Hutchison et al. 1994). Incremental breathing shares features with grunting with the addition of timing changes in laryngeal and pump muscle activities at end-expiration when the onset of pump muscle activity occurs before laryngeal muscles open the glottis fully. The result is a decrease in expired volume and an increase in EEV (Hutchison et al. 1994). This incremental mechanical process is aided by the airway stability accrued from the increased end-expiratory positive subglottic pressure that limits the development of a negative airway pressure during inspiration. Given their tendency to chest wall distortion, it is not surprising that incremental breathing can occur in preterm neonates (Eichenwald et al. 1992). Incremental breathing is also seen in gasping during acute cerebral hypoxia–ischemia (Hutchison et al. 2002). Gasping is typified by brief laryngeal abductor and pump muscle activities followed by long periods of laryngeal adductor activity with apnea (Hutchison et al. 2002). Subglottic pressure is increased, maintaining airway volume and likely promoting autoresuscitation (Hutchison et al. 2002).
4.1.5 Control of Coordinated Laryngeal and Pump Muscle Activities
4.1.5.1 Laryngeal Protective, Lower Airway and Chest Wall Afferents
Nasal and laryngeal afferent inputs alter laryngeal abductor and adductor activities and thus glottic size. The laryngochemoreflex (LCR), whose afferent pathway is the SLN, results in protective responses, including swallowing, apnea, obstructed respiratory efforts, cough, hypertension, and arousal from sleep (Thach 2008). An obstructive apnea with bradycardia results if the LCR stimulus is persistent, especially in the immature or depressed brain. This LCR response alters with age – coughing becomes the major response. However, acquisition of a viral infection in infancy can rekindle its potency (Thach 2008). It is augmented by hypoxemia and anemia and has been implicated in apnea of prematurity and SIDS (Thach 2008) (see Sect. 47.3.1).
Other laryngeal SLN afferents, including those from mechanoreceptors, drive receptors, and temperature receptors, affect laryngeal intrinsic muscle activities. Although SLN section does not alter the eupneic breathing pattern, these afferents are important. Upper airway bypass can alter the inspiration-inhibition Hering–Breuer reflex. Application of acid to the larynx in animals, mimicking chronic aspiration, alters the subsequent response to an applied airway load (see Sect. 47.3.1). During noninvasive ventilation, nonsynchronous delivery of airflow to the upper airway in neural expiration results in laryngeal closure (Rodenstein 2004; Scharf et al. 1978). Thus, noninvasive ventilation and pacing of a paralyzed diaphragm should be applied synchronously with neural inspiration (Rodenstein 2004; Scharf et al. 1978).
In intact animals, compensatory glottic abduction follows single-breath total occlusion of inspiration or expiration at the mouth/nose. During the unimpeded expiration following a single inspiratory occlusive load, unopposed TA activity can produce laryngeal closure. Thus when no air enters the lung, a compensatory reflex mechanism can prevent expiratory volume loss, maintaining absolute subglottic volume. Lower airway afferent inputs affect glottic size (Bailey and Fregosi 2006). Stretch receptor discharges, induced by PEEP, decrease expiratory TA activity (Harding 1986). By contrast increased expiratory glottic adduction follows rapidly adapting receptor stimulation, e.g., with deflation or irritant stimulation secondary to a pneumothorax (Bartlett 1989), or follows C-fiber stimulation, e.g., with experimental pulmonary edema (Bartlett 1989). Direct and indirect stimulation of chest wall muscle afferents can invoke several flow patterns involving glottic closure (Bartlett 1989; Stecenko and Hutchison 1991).
While pseudoasthma can be fabricated by partial glottic closure (Rodenstein 2004), matching laryngeal control of flow pattern to maintain optimal subglottic airway volume may explain changes reported with true increased airway resistance. Subglottic inspiratory flow limitation in croup is associated with expiratory flow resistance, probably glottic in origin (Argent et al. 2008). Increased lower airway resistance in adult asthmatic patients induces a breathing pattern characterized by laryngeal expiratory flow retardation (Collett et al. 1983; Sekizawa et al. 1987). In adults, resistive loads applied at the mouth also decrease expiratory glottic size (Brancatisano et al. 1985). This change may occur immediately, suggesting that resistive loading, unlike total occlusive (elastic) loading, produces sudden flow changes that stimulate airway receptors to cause laryngeal adduction (Brancatisano et al. 1985). Therapy with CPAP reverses the expiratory laryngeal adduction in some asthmatic subjects, suggesting that airway pressure changes may alter the dynamics between stretch receptor and irritant receptor stimulations, thus changing the breathing pattern (Collett et al. 1983). In normal adults a voluntary deep breath can decrease laryngeal resistance and lower airway resistance (Sekizawa et al. 1987). By contrast, bronchoconstrictive stimulation in normal adults can result in inspiratory and expiratory glottic narrowing (Higenbottam 1980). An increased resistance to inspiratory flow may be advantageous in that more transpulmonary pressure is applied to opening a constricted peripheral airway. If lower airway volume and resistance changes affect laryngeal function, can absence of laryngeal control affect lower airway resistance? Laryngeal bypass during invasive ventilation is associated with atelectasis and with the development of increased lower airway resistance (Hutchison and Bignall 2008). The latter effect may stiffen the conducting airways and thus stabilize total airway volume but demand increased effort. In summary, the data reflect the importance of laryngeal subglottic volume control as a determinant of lower airway resistance and point to interactions between lower airway afferents and coordinated laryngeal and pump muscle activities in this dynamic process (see also Sect. 47.3.1).
4.1.5.2 Central and Chemical Control
Central control of coordinated laryngeal and diaphragmatic muscle activities is determined by developmental stage, behavioral states, metabolism (temperature), central inputs, and centrally acting chemicals. Postnatally in lambs, increased expiratory TA activity with decreased PCA activity occurs during the NREM state. By contrast, expiratory TA activity in REM is mainly absent, while PCA activity is variable (Harding 1986). In normal adult humans, expiratory TA activity is absent during stable NREM, while expiratory PCA activity decreases in NREM and is variable in REM (Kuna et al. 1988, 1990). At all ages, expiratory TA activity occurs at arousal. Thus behavioral state is a key factor in subglottic airway volume control that, in turn, is important in sleep apnea.
Increased central drive, with hyperventilation and/or hypercapnia, promotes laryngeal abduction in inspiration and expiration in adults and term newborns, in whom increased PCA, diaphragmatic and intercostal muscle activities occur (Bartlett 1989; Insalaco et al. 1990; Kuna et al. 1994; Wozniak et al. 1993). Laryngeal adduction can occur during hypercapnic hyperventilation in preterm neonates, probably due to chest wall distortion (but see also Sect. 47.3.1) (Eichenwald et al. 1993). This adduction also occurs in adults at the mechanical limits of airway volume (Brancatisano et al. 1983). In general, however, increased central drive decreases laryngeal resistance unless mechanical limitations are present.
Decreased central drive with hypocapnia diminishes expiratory glottic size and is associated with periodic breathing in adults (Kuna et al. 1993; Rodenstein 2004). Laryngeal closure has been noted in human newborns and infants with apnea or suspected apparent life-threatening events (Ruggins and Milner 1991, 1993). During central apnea and periodic breathing in lambs, expiratory TA activity is noted (Praud 1999). In depressed human infants at birth, laryngeal closure can block intubation, and, in lambs, acute cerebral hypoxia–ischemia results in expiratory TA activity with laryngeal closure (Hutchison et al. 2002). Centrally acting depressant drugs diminish central drive and, in lambs, produce laryngeal closure with apnea (Praud 1999). In former preterm infants, up to ~55–60 postmenstrual weeks, exposure to anesthesia and/or operative stress can result in apnea postoperatively (see Sect. 47.3.1). Thus, the intensivist should avoid hypocapnia during noninvasive ventilation and be aware that central depression can result in apnea with glottic closure.
Hypoxia stimulates expiratory laryngeal abduction and increases ventilation in human adults and newborn lambs (England et al. 1982b; Insalaco et al. 1990; Praud et al. 1992). In lambs, the increase in ventilation is dependent upon carotid body input – a sudden decrease in that input induces expiratory TA activity (Praud et al. 1992). However, animal and human data indicate that the effects of carotid body input vary depending upon central state and the presence/absence of other inputs (de Burgh Daly et al. 1979). If animals are vagotomized and paralyzed, direct carotid body stimulation can induce expiratory TA activity, and exposure to hypoxia after airway vagal blockade or intrathoracic vagal section results in laryngeal adduction (Bailey and Fregosi 2006). In adult humans, the degree of expiratory glottic adduction with hypoxia exceeds that during hypercapnia (England et al. 1982b). Thus, it is argued that the “pure” carotid body reflex response is laryngeal expiratory adduction that will retain airway volume and promote oxygenation. This pure response can be countered by the presence of ventilation which increases airway stretch receptor feedback that results in an overall expiratory laryngeal abduction response. In the author’s view, a unifying explanation for the diverse findings with hypoxia is that the effect of carotid body input is to augment the central coordinated output to laryngeal and pump muscles that is selected under different conditions (see Sect. 47.3.1). When central depression exists, protective inputs and/or absence of volume-related inputs produce an apneic response with glottic closure that is augmented by hypoxia. This can be seen in the “vagal” preterm infant who is sedated for a surgical procedure. At intubation laryngeal closure and apnea/bradycardia can be triggered. If hypoxemia ensues, the problems are aggravated. When tidal ventilation and airway volume feedback are restored, the impact of any ongoing hypoxemia is to augment breathing. Hering and Breuer found that their volume-related reflexes were active even with hydrogen breathing. This fits the modern focus on ventilation for resuscitation from asphyxia.
4.1.6 Conclusion
This chapter of upper airway physiology for the pediatric intensivist has focused on motor and specifically on laryngeal aspects relevant to the control of breathing patterns. The major message is that upper airway physiology is involved in all aspects of “breathing”: protection, volume homeostasis, and ventilation; its functional impact covers the entire airway, from the nose to the alveolus. Understanding upper airway physiology can guide and improve therapy, while therapy can aid a return to normal homeostasis or detract from it. Current knowledge of upper airway physiology has had major implications for the application of invasive and noninvasive ventilatory support. Much remains to be learned from the interactions between physics and biochemistry in the entire airway and how they affect breathing patterns.
Essentials to Remember
-
The upper airway plays roles in all the extended functions of breathing, namely, in airway protection, in the control of supra- and subglottic volumes, and in tidal ventilation.
-
Nasal functions include air conditioning and airway protection. Stimulation can result in profuse secretions, altered patency, and the cardiorespiratory dive reflex. Obstruction can affect gas exchange markedly; thus, a rapid switch to oral breathing is advantageous.
-
Pharyngeal patency can be altered in sleep. Nonnutritive swallowing is vital to minimize aspiration. Therapy with CPAP improves pharyngeal patency in OSA but may alter pharyngeal clearing mechanisms and increase gastric air.
-
Laryngeal closure can protect the airway rapidly. Laryngospasm can be hard to treat.
-
Controlled expiratory laryngeal closure modifies airflow and subglottic airway volume in normal breathing, at the establishment of airway volume at birth or when airway volume is threatened by mechanical, chemical, or central changes.
-
Intrinsically or extrinsically induced changes in central state alter the laryngeal motor outputs in response to mechanical or chemical inputs.
-
Understanding how laryngeal motor functions affect breathing patterns, airway pressures, and gas exchange is at the core of many technical and procedural advances in the provision of resuscitative measures and of invasive and noninvasive respiratory support.
Acknowledgements The author thanks L. S. Segers, PhD; B. G. Lindsey, PhD; and B. M. Schnapf, DO, for critical review and J.D. Carver, PhD and M-F. Hutchison, MA for editorial input.
4.2 Mechanics of the Lung, Airways, and the Chest Wall
Paul D. Robinson9, 10 and J. Jane Pillow11, 12
(9)
Department of Respiratory Medicine, The Children’s Hospital at Westmead, Locked Bag 4001, Westmead, NSW, 2145, Australia
(10)
The Discipline of Paediatrics and Child Health, The University of Sydney, Sydney, Australia
(11)
School of Anatomy, Physiology and Human Biology and Centre for Neonatal Research and Education, The University of Western Australia, 35 Stirling Highway, Crawley, 6009, Western Australia
(12)
Consultant Neonatologist, King Edward Memorial Hospital, 374 Bagot Road, Subiaco, 6008, Western Australia
Educational Aim
To describe the principal components of respiratory mechanics of the airways, lungs, and the chest wall and the developmental and environmental considerations that impact on these measurements in the neonatal and young child
4.2.1 Background
The mechanical properties of the respiratory system are a critical component of the chain of events that result in translation of the output of the respiratory rhythm generator to ventilation. A comprehensive understanding of respiratory mechanics is therefore essential to the delivery of optimized and individualized mechanical ventilation. This chapter describes the basic elements of respiratory mechanics and reviews the developmental changes in the airways, lungs, and chest wall that impact on measurement of respiratory mechanics with advancing postnatal age.
During spontaneous breathing, the forces driving inspiration are generated by the inspiratory muscles, whereas during mechanical ventilation, the forces are generated by the ventilator or by a combination of the ventilator with spontaneous inspiratory muscle contributions. The inspiratory muscle pressure needs to be sufficient to overcome three major mechanical properties of the respiratory system: elastance (E) (to overcome tissue forces required to change the lung volume (V)), resistance (R) (to overcome resistance to flow (V’)), and inertance (I) (for acceleration (V”) of gas volumes). Elastance is often considered in terms of its inverse, the compliance (C). The total pressure (P total) generated is equal to the sum of the elastic (P el, proportional to volume and 1/compliance), resistive (P res, proportional to flow and resistance), and inertive (P in, proportional to acceleration and inertance) components:

(4.1)
Although pressure losses to inertia are considerable during high-frequency ventilation, the inertive component is normally a small portion of P total at normal breathing frequencies and can be effectively neglected. Thus the equation driving flow during normal respiration can be simplified:

(4.2)
Nonetheless, the linearized relation of driving pressure to volume and flow does not fully characterize the pediatric respiratory system: Resistance and compliance are both dependent on volume, volume history, and flow, particularly in the very small or premature neonate. Further, the simplified equation does not fully incorporate the complexities of the respiratory system.
Almost all the tests used to measure respiratory mechanics in the infant and pediatric population have been developed in adults and then adapted for younger subjects. Measurements of respiratory mechanics are most appropriately corrected to lung volume, such as functional residual capacity (FRC). In the absence of a measure of FRC, measurements of respiratory mechanics made in infancy are often related to body size (e.g., height/length or weight). Measurements at the body surface are necessary to include the contributions of the chest wall. The chest wall contribution to respiratory mechanics can be isolated, by subtraction of the pleural measurements from those obtained at the body surface (e.g., airway opening).
4.2.1.1 Passive Tests of Respiratory Mechanics
The respiratory system is in a passive state when there is no inspiratory muscle activity (P mus = 0). A passive condition is present in the paralyzed patient but can also occur without paralysis following hyperventilation (Mortola 2004). Under passive conditions, resting lung volume is determined by two opposing forces. The outward recoil of the chest wall results from the elastic characteristics of the diaphragm/abdomen and of the rib cage. The outward recoil of the chest wall directly opposes the inward recoil of the lung. Inward recoil is determined by the viscoelastic properties of the lung, including the mechanical properties of the lung tissues, and the collapsing pressure generated at the alveolar air–liquid interface.
During spontaneous breathing, the passive respiratory mechanics can be measured using occlusion techniques. Occlusion of the airways at lung volumes above the resting lung volume relaxes the respiratory muscles by stimulating the slowly adapting stretch receptors and the vagally mediated Hering–Breuer inflation reflex. Passive tests assess the mechanical properties of the entire respiratory system. Simultaneous measurement of transpulmonary pressure allows measurement of respiratory system compliance (C rs) to be partitioned into lung (C L) and chest wall (C w) components. Transpulmonary pressure (P tp) is derived from the difference in pressure at the airway opening (P ao) and pleural pressure (or elastic recoil pressure, P el), measured using an esophageal balloon, liquid-filled catheter, or miniature pressure transducer.
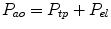
(4.3)
Other passive measurements of respiratory mechanics include forced expiratory maneuvers to provide measures of maximal flow at FRC (V′max,FRC) (Lum et al. 2006a). Partial forced expiratory flow–volume curves in infants are obtained by rapid compression of the chest through end-inspiratory inflation of a jacket placed around the chest and abdomen. During tidal breathing, this rapid thoracoabdominal compression (RTC) technique provides a V′max,FRC that is similar to forced flows at low lung volumes (e.g., maximal expiratory flow MEF25 %,FRC). Modification of the RTC technique by raising lung volume of the infant towards total lung capacity (so-called raised volume RTC or RVRTC) allows assessment of forced expiratory flows over a more extended range of volumes. Reported measures include forced vital capacity (FVC) and forced expiratory volumes after defined time periods (e.g., 0.4, 0.5, or 1 s, termed FEV0.4, FEV0.5, and FEV1, respectively) and the ratio of these values e.g., (FEVt/FVC, where t is the time period chosen).
4.2.1.2 Dynamic Tests of Respiratory Mechanics
In contrast, dynamic tests of respiratory mechanics allow the relative contributions of the airways and lung tissues to be partitioned, in addition to separately identifying the chest wall contribution to airway and tissue mechanical properties. Dynamic mechanics derive mechanical variables from analysis of dynamic waveforms obtained either during normal (tidal) breathing or via imposed oscillatory waveforms. During tidal breathing, multilinear regression is used to determine dynamic respiratory system compliance (C dyn,rs) and resistance (R rs). Kano and colleagues showed that consideration of volume dependence is important when using this approach to assess dynamic mechanics during mechanical ventilation. Consideration of volume dependence is important because ventilatory settings may “push” the pressure–volume curve onto the flattened upper portion of the curve (Kano et al. 1994). In the overdistended lung, fits obtained by multilinear regression are improved by the inclusion of a volume-dependent elastance term in the regression model:
where P ao is the airway opening pressure, V is the tidal volume, E 1 + E 2 V is the total elastance over the cycle (E 1 and E 2 V representing the volume-independent and volume-dependent portions of elastance, respectively), and the final term P A,EE represents the alveolar pressure at end-expiration.

(4.4)
An important consideration during the interpretation of dynamic respiratory mechanics is the concept of frequency dependence. Kano showed that increasing the ventilation rate from 30 to 80 breaths/min in a group of near-term newborn infants increased E rs and R rs by a mean of 8.3 and 17.5 %, respectively (Kano et al. 1994). Frequency dependence and the mechanical properties of the respiratory system under normal tidal breathing conditions are also determined using forced oscillatory techniques (FOT). These techniques are essentially noninvasive and are especially useful in infants and children as no active subject cooperation is required. The technique involves application of a pressure waveform (forcing function) to the airway opening and measurement of the resultant flow. The measured impedance reflects the complex viscoelastic resistance of the respiratory system (Z rs). Simultaneous measurement of the transpulmonary pressure and hence chest wall impedance (Z w) allows determination of the lung impedance (Z L) by subtraction. The impedance (Z) can be separated into the resistance (R rs), the impedance component in phase with flow, and reactance (X rs), representing the impedance components in phase with volume (elastance) and acceleration (inertance).
The low-frequency forced oscillation technique (LFOT) measures impedance over a range of frequencies (usually ~0.5–20 Hz). LFOT is obtained in infants by invoking the Hering–Breuer inflation reflex to inhibit temporarily any respiratory muscle activity, during which brief time (6–10 s) the LFOT signal is applied. Fitting the constant-phase model to the resultant pressure and flow data permits partitioning of respiratory mechanics into frequency-independent airway resistance (R aw) and airway inertance (I aw) and a constant-phase tissue component. This constant-phase tissue component is described by [(G − jH)/ωα, where G and H are coefficients for tissue damping and elastance, respectively, ω is angular frequency, and α determines the frequency dependence of the real (pressure change in phase with flow) and imaginary (pressure change in phase with volume) parts of the impedance] (Hantos et al. 1992a).
The interrupter technique provides a further option to assess partitioned mechanics. The interrupter technique involves measurement of changes in airway opening pressure after a mid-expiratory occlusion, which is used to calculate airway resistance (R aw). After airway occlusion at mid-expiration, there is a biphasic change in P ao: the immediate rapid rise in P ao represents the resistive pressure drop across the conducting airways and is followed by a secondary slower increase in P ao (often referred to as P dif) generally attributed to stress recovery in the respiratory tissues (lung and chest wall) and gas redistribution associated with ventilation inhomogeneity (Bates et al. 1988). A major limitation of this technique is that it requires mechanical ventilation and paralysis to obtain reliable data.
4.2.2 Developmental Considerations
Particular challenges for lung function testing in infants and children include the marked developmental changes occurring over the first months and years of life. Additional considerations include position, sleep state and sedation, and feasibility of individual tests given minimal capacity of these age groups for active cooperation and preferential nasal breathing (Stocks and Hislop 1996). Thus, in contrast to the adult and older child, tests in infants are performed using a mask instead of a mouthpiece and nose clips.
4.2.2.1 Airway and Lung Development
Lung development represents a period of considerable change in both lung architecture and volume and is affected by several factors including genetic, in utero and postnatal environmental exposures, somatic growth, puberty, changes in muscularity, and ongoing alveolarization. Our understanding of the interactions between these factors is based on a mix of pathological and physiological data, which are predominantly cross-sectional in nature. Consensus between these studies is lacking. While some of the lack of agreement is attributable to technical approaches used by different authors, a comprehensive understanding of factors influencing respiratory mechanics over time can only be answered by strong longitudinal data, which are urgently required. These tests of lung mechanics provide us with essential insight into how tissue properties change with development, and a full understanding of the changes that occur during healthy development is an important step to detect the subsequent effects of disease and response of the individual to therapeutic interventions. Measurements need to be accompanied by accurate records of height (length) and weight to facilitate interpretation of longitudinal data.
4.2.2.1.1 Dysanapsis
Over the course of development, there is considerable remodelling of all of the structural components of the respiratory system, although the exact patterns of change in airways, alveoli, and blood vessels may differ with respect to each other. The term “dysanaptic growth” was originally introduced by Green et al. (1974) and describes the disproportionate but physiologically normal growth of the lung relative to the airways. In infancy, airway size relative to lung volume is larger than in older children and adults. There is considerable debate in the pediatric literature about the degree, extent, and stage of development to which biological variability of airway size to lung volume occurs. The data outlined in this chapter suggest that dysanapsis is a feature of all stages of postnatal lung development.
Whether disproportionate growth along the length of the airway tree occurs is less clear. Increased growth of the larger central airways, in relation to peripheral generations, has been described through the first year of life, after which point adult relative proportions are achieved and maintained through further growth (Horsfield et al. 1987). Disproportionate central and peripheral airway growth, however, is not a consistent finding in the literature (Hislop et al. 1972). The most rapid period of tracheal growth appears to occur during the first 4 years of life (Wailoo and Emery 1982). An elevated ratio of peripheral airway resistance to central airway resistance (and its contribution to total airway resistance) in young children compared to adults was originally proposed by Hogg et al. (1970), based on retrograde catheter measurements. The authors described relatively little change in the contribution of the central airways (those proximal to the 12th–15th airway generations) to conductance with age but marked increase in peripheral airway conductance from the age of 5 years of age. These changes were attributed to altered linear dimensions of the peripheral airways, based on morphometric data. The ratio of central to peripheral resistance can also be examined noninvasively by comparing measured forced expiratory flows (FEF) breathing air to FEF measured breathing a less-dense gas mixture of 80 % helium/20 % oxygen (heliox). FEF measured breathing heliox is typically higher than during air breathing (e.g., 1.2–1.6 times at 50 % FVC). In older subjects with peripheral airway disease, this pattern is lost with ratios approaching parity (Cooper et al. 1983; Dosman et al. 1975). Density dependence data during infancy, in comparison to values reported in later life, appear to challenge the findings of Hogg et al. (1970). Davis et al. examined the changes in density dependence through the first 2 years of life (Davis et al. 1999). FEF 30–40 % higher were measured with heliox: although no relationship existed between density dependence and age, length, or FVC in the first 2 years of life (in keeping with Hogg et al. (1970)), the values obtained were similar to those reported across 16 other studies in older children ranging from school age to adulthood (summarized in Table 2 of the original manuscript (Davis et al. 1999)). These equivalent cross-sectional density dependence values suggest that there is no difference in the convective accelerative and turbulent pressure loss with age, supporting the notion that the ratio of resistance of the peripheral to the central airways is similar in infants, older children, and adults.
4.2.2.2 Sex Differences in Respiratory Mechanics
Sex-specific differences in respiratory mechanics are well recognized in infants and young children and are a particularly important consideration in forced expiratory maneuvers in infants. Airways are larger in females before puberty, and this difference is detectable from infancy (Tepper et al. 1986a; Hoo et al. 2002a): V′max,FRC is approximately 20 % higher in girls over the first 9 months of life (Hoo et al. 2002a). Similar sex-dependent increases in V′max,FRC are evident in preterm girls compared to preterm boys, suggesting such differences in lung function may be developmental rather than environmental in origin (Stocks et al. 1997). Larger lungs have been reported in males between the ages of 6 weeks and 14 years (Thurlbeck 1982). Presence and timing of the growth spurt is an important confounding factor, with lung growth occurring out of phase to somatic growth, especially in boys (Degroodt et al. 1986; Quanjer et al. 1989; Schrader et al. 1984). Lung development in females is almost complete following menarche but continues throughout puberty in males (Neve et al. 2002).
4.2.2.3 Periconceptional and Intrauterine Exposures
There is increasing evidence that the periconceptional and intrauterine exposures impact on development of the respiratory system and consequently impact on respiratory mechanics in infancy and childhood. Factors known to influence lung mechanics after birth include maternal smoking, nutritional deprivation and intrauterine growth restriction, infection and/or inflammation, and maternal glucocorticoids. Prenatal exposure to nicotine may cause abnormal airway branching and dimensions as well as increase airway smooth muscle and collagen deposition resulting in reduced lung function at birth that persists into early adulthood regardless of postnatal exposure (Hayatbakhsh et al. 2009; Svanes et al. 2004). Lung function irregularities include airflow limitation, reduced FEV1, and airway hyperreactivity (Sandberg et al. 2011; Wongtrakool et al. 2012). Chronic restriction of nutrients and/or oxygen in late pregnancy impairs development of the fetal small airways and lungs, including reduced numbers of alveoli that are also enlarged with increased septal wall thickness and basement membranes compared to nonexposed infants (Pike et al. 2012). Such differences in alveolar number can influence the subsequent rate of disease progression, including an accelerated rate of decline in FEV1 with advancing age (Stocks and Sonnappa 2013).
4.2.3 Mechanical Properties of the Airways, Lung, and Chest Wall in Infancy and Childhood
4.2.3.1 The Airways
Several cross-sectional studies have looked at the changes in airway resistance (reflecting airway size) during childhood. Hall and colleagues using LFOT described a quasilinear decrease in R aw with increasing length during infancy in 37 infants, including some with repeat measurements (Hall et al. 2000). Lanteri and Sly examined changes across the pediatric age range and also reported linear decreases in both R rs and R aw with increasing height (when plotted on a log–log plot) in 51 children (range 3 weeks–15 years) with healthy lungs (Fig. 4.7) (Lanteri and Sly 1993). Pooled data across the preschool age range reinforces the consistent relationship seen with increasing height (Beydon et al. 2007a). In a study of 40 subjects, across the first 5 years of life, Gerhardt et al. demonstrated an increase in lung conductance (the reciprocal of resistance), of approximately fivefold over the weight range examined (Gerhardt et al. 1987a). This increase occurred at a slower rate than the increase in FRC, which led to a rapid drop in specific conductance (Fig. 4.8b). This rapid decrease in specific airway resistance or conductance through early life has been described previously (Doershuk et al. 1974; Stocks and Godfrey 1977). Tepper et al. measured maximal FEF at FRC, from partial expiratory flow–volume curves and corrected for changes in lung volume: they reported higher flows in the neonatal range which decreased to a steady value through the 2nd year of life, similar to those reported elsewhere in older children (Tepper et al. 1986a). Differential increases in anatomic dead space volume (twofold), compared to FRC (threefold), have also been shown in the pediatric population (Wood et al. 1971).
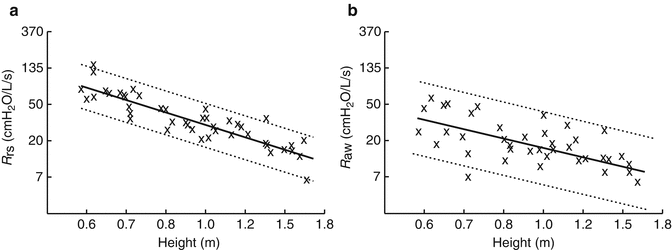
Fig. 4.7
Decreasing resistance of the respiratory system (R rs) and airway resistance (R aw) with increasing height. Cross-sectional data taken from 51 subjects (aged 3 weeks to 15 years) (Lanteri and Sly 1993). Both resistance and height are plotted as natural log values but are labeled with absolute numbers for clarity
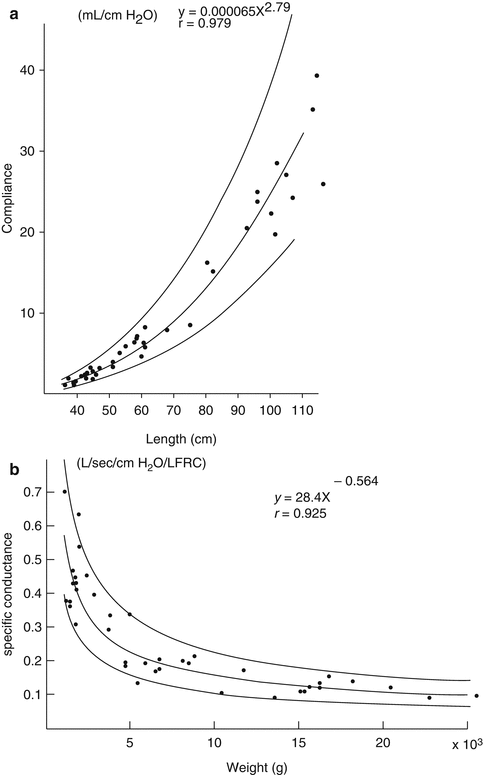
Fig. 4.8
Rapid increase in lung compliance (a) and rapid decrease in specific conductance (b), with increase in body length, based on cross-sectional data in 40 subjects in the first 5 years of life (Gerhardt et al. 1987a). The regression line (solid) and 95 % confidence interval (dotted lines) are shown (Reproduced with permission from the publisher)
4.2.3.2 The Lungs
Rapid postnatal increases in lung volume are reported widely. FRC increases by a magnitude of 40 times (from 80 mL in infants to 3,000 mL in adults) (Dunnill 1982) and over ten times in total lung weight (Polgar and Weng 1979). The rapid growth of the distal lung, in comparison to relatively steady changes in airway dimensions, is a major feature of respiratory system development beyond birth. After the appearance of primitive saccules at approximately 28 weeks gestation, mature alveoli with secondary septa appear from 34 weeks gestation onwards. Alveolar multiplication was initially thought to cease at around 2 years of age (Thurlbeck 1982). More recent estimates indicate that increase in alveolar number continues until at least 8 years (Dunnill 1982). Recent advanced imaging studies using hyperpolarized helium magnetic resonance imaging (MRI) provide evidence of ongoing alveolar multiplication in adolescence (Narayanan et al. 2012). Increase in alveolar size (expansion) is also evident during infancy and childhood, with recent epidemiology studies suggesting lung size increases into early adulthood, when chest wall development is complete (Quanjer et al. 1989; Reid 1977).
Based on cross-sectional studies, specific lung compliance falls during early infancy before remaining relatively constant from the early preschool years. Tepper et al., based on measurements of compliance from the linear portion of the static pressure–volume (PV) curve in almost 50 infants, described an increase in lung compliance over the age range studied, but decreasing specific lung compliance with increasing body length in the first 2 years of life (Tepper et al. 2001). Gerhardt et al. examined similar changes in 40 infants and children over the first 5 years of life. Lung compliance increased markedly (by ~20–25-fold) over the weight range examined (Fig. 4.8a) but in proportion to FRC, resulting in a constant-specific compliance over the first 5 years of life. The same pattern of findings was reported in 63 children aged 2–7 years (Greenough et al. 1986). This initial rapid period of alveolar growth is also supported by evidence from measurements using the interrupter technique and FOT. Lanteri and Sly showed a biphasic change in P dif with age: P dif was highest in young infants, falling rapidly over the first 12 months, before increasing again after approximately 5 years of age (Lanteri and Sly 1993). Hall and colleagues, using LFOT measures of tissue mechanics derived by fitting data to the constant-phase model, showed that both tissue parameters G and H decreased in a quasihyperbolic manner with increasing length between 7 weeks and 2 years of age (Hall et al. 2000). The difference in the pattern of change in G and H compared to the change in R aw with increasing length is further evidence of dysanapsis: tissue mechanical properties change more rapidly than airway mechanics over the first 2 years of life (Fig. 4.9).
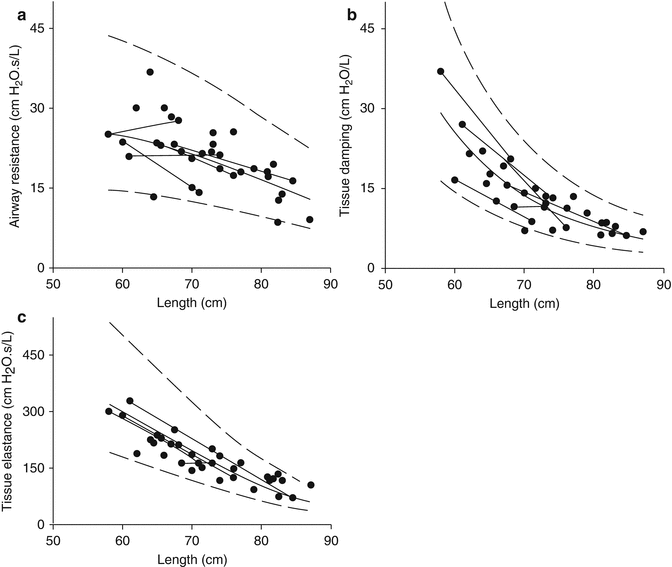
Fig. 4.9
Low-frequency FOT data plotted against length in 37 infants: airway resistance (raw, a), tissue damping (b), and tissue elastance (c). The greater rate of change suggested in tissue (b, c) compared to airway properties (a) illustrates dysanaptic lung growth (Hall et al. 2000). Reprinted with permission of the American Thoracic Society (copyright © 2014 American Thoracic Society)
4.2.3.3 The Chest Wall and Respiratory Musculature
The chest wall of the neonate and infant, and to a lesser extent the growing child, is especially compliant compared to the compliance of the chest wall in the adult subject. A highly compliant chest wall in infancy results in less opposition to the tendency of the lung to collapse, promoting a low residual lung volume and also distortion of the chest wall, resulting in a loss of volume during inspiration. The disproportionately high compliance of the chest wall compared to the compliances of the lung means that the compliance of the respiratory system (C rs) is approximately equal to the compliance of the lung (C L) during childhood, especially in neonates and infancy (Davis et al. 1988; Gerhardt et al. 1987a). Several active (dynamic) mechanisms of respiratory mechanics serve to partially compensate for the instability in FRC associated with a compliant chest wall and tendency for small airway closure during tidal breathing. Infants acutely increase their end-expiratory lung volume (EELV) and maintain it above resting lung volume by modulating (abbreviating) their expiratory time (Mortola and Saetta 1987) (Fig. 4.10a). In addition, they reduce expiratory flow through post-inspiratory activity of the diaphragm and inspiratory chest wall muscles (Kosch and Stark 1984) (Fig. 4.10b) (Lopes et al. 1981), stiffening the chest wall and by increasing laryngeal resistance (± glottic closure) briefly (Kosch et al. 1988). Such dynamic modifications of respiratory mechanics occur via neural (vagal) receptor-mediated reflexes. Restriction of lung function measurement to periods of quiet sleep is necessary to reduce the variability in measurements resulting from such dynamic processes (Henschen and Stocks 1999).
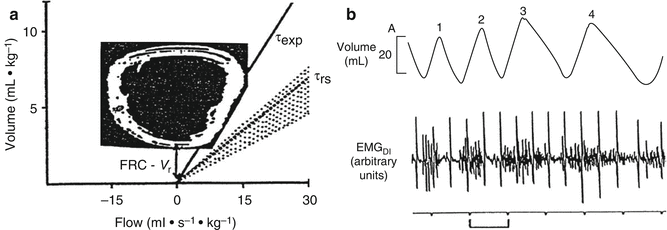
Fig. 4.10
Employment of active (dynamic) mechanisms to elevate end-expiratory lung volume (EELV) in infants. In (a), active prolongation of the respiratory system time constant (τ) is shown. The expiratory time constant (τexp) is shown as the slope of the measured expiratory limb of the tidal flow–volume curve (solid line). This is compared to the passive expiratory time constant (slope of the dotted line) measured after an end-inspiratory occlusion. The difference between the two slopes is generated due to active use of the respiratory muscles during expiration (“braking”). The magnitude of difference between the active flow–volume curve-derived EELV (FRC) and the passive relaxation volume (V r) reflects the degree of active (dynamic) mechanisms employed to elevate EELV. This active use of respiratory muscles is also illustrated in (b) from data collected in a separate infant study wherein diaphragm EMG activity of breaths three and four extends into expiration (Both figures reproduced with permission from the publishers (Mortola and Saetta 1987; Kosch and Stark 1984))
The changes in chest wall, lung, and respiratory system mechanics over the first 4 years of life were examined by Papastamelos et al., in 40 subjects (Papastamelos et al. 1995). The authors used a modified Mead–Whittenberger technique (Cook et al. 1957): manual ventilation overrode respiratory drive and relaxed the respiratory muscles, avoiding the need for intubation or induction of a Hering–Breuer reflex. In this cohort, the ratio of chest wall to lung compliance fell from a mean (SD) of 2.9 (1.1) to 1.3 (0.4) (p < 0.005). Chest wall to lung compliance ratios are even higher in preterm infants (Gerhardt and Bancalari 1980). The stiffening of the chest wall, due to rapid rib ossification, increasing musculature, and altered chest wall configuration (Bryan and Wohl 1986; Mead 1979; Openshaw et al. 1984; Leiter et al. 1986), is such that near-adult values, where lung and chest wall compliance are equal (Mittman et al. 1965), are reached after the first year of life.
Stiffening of the chest wall over the first year of life allows the infant to shift its maintenance of FRC from dynamic elevation of EELV to a more passive mechanism achieved by the balance of outward recoil of the chest wall and inward recoil of the lung (Colin et al. 1989). Stiffening of the chest wall likely also explains the increase in P dif in children after age of 5 years observed by Lanteri and Sly (Lanteri and Sly 1993). FRC as a proportion of total lung capacity (TLC) increases with age through childhood, in the majority (Engstrom et al. 1956; Mansell et al. 1977; Weng and Levison 1969), but not all (Schrader et al. 1988), studies to date. Residual volume (RV), as a proportion of TLC, also increases with age through childhood (Merkus et al. 1993), while closing capacity (measured using single-breath nitrogen washout) decreases significantly with age, as a proportion of TLC, converging towards RV (Mansell et al. 1977). This increased RV/TLC ratio suggests that increased stiffness of the chest wall is not entirely compensated for by increase in expiratory muscle force over time (Schrader et al. 1988). The study by Merkus et al. also highlighted the need to use TLC as a measure of lung growth and not FVC, due to the fact that an increasing RV/TLC with age led to errors in maximal expired flows due to measurement at progressively higher lung volumes (Merkus et al. 1993).
4.2.3.4 Summary
The postnatal period is characterized by ongoing rapid lung development, with differing rates of growth seen in the airway, lung parenchyma, and chest wall. The dysanapsis that occurs between the components of the respiratory system has important consequences for measurements of lung mechanics performed during infancy and childhood. Accounting for these developmental changes and dysanapsis is necessary to optimize disease detection and subsequent assessment of an individual’s response to interventions. Insights into these changes are largely derived from cross-sectional studies, which have identified important sources of potential error in existing methodology. Strong longitudinal data are now urgently required to confirm these apparent patterns and answer the important questions that remain.
Essentials to Remember
-
The postnatal period is characterized by ongoing rapid lung development in all parts of the respiratory system (airways, lung parenchyma, and chest wall). The physiologically normal rate of change of the lung relative to the airways is disproportionate. This dysanaptic growth pattern appears to be a feature of all stages of postnatal lung development.
-
Passive measures of lung mechanics provide information about the mechanics of the entire respiratory system.
-
Dynamic measures of lung mechanics allow partitioning of the lung and chest wall components to separately identify the chest wall contribution to airway and tissue mechanical properties.
-
Standardization of the measurement technique is essential. Results generated are corrected for either lung volume (e.g., FRC) or, in the absence of this, a measure of growth (e.g., weight).
-
A number of periconceptional, intrauterine, and postnatal factors impact on the development and mechanics of the respiratory system. Important postnatal factors include maternal smoking, nutritional deprivation and intrauterine growth restriction, infection and/or inflammation, and maternal glucocorticoids.
-
The rate of airway growth (detected by increases in conductance or decreases in resistance) is not as rapid as the rate of FRC increase with age. This greater relative increase in lung volume leads to a rapid decrease in specific resistance during early childhood.
-
Postnatal alveolarization appears to now extend into adolescence. Rapid alveolar growth occurs during infancy leading to an initial fall in specific lung compliance, which reaches a plateau during ongoing lung growth. These changes are also evidenced by the changes seen in LFOT tissue parameters and interrupter technique-derived P dif.
-
The highly compliant chest wall of the infant leads to employment of active (dynamic) measures to maintain EELV above FRC. As the ratio of chest wall to lung compliance falls and reaches the adult values of parity beyond infancy, these gradually transition to more passive mechanisms as the chest wall stiffens through early childhood.
4.3 Respiratory Mechanics in Neonatal Pathologies
J. Jane Pillow13
(13)
School of Anatomy, Physiology and Human Biology and Centre for Neonatal Research and Education, The University of Western Australia, 35 Stirling Highway, Crawley, 6009, Western Australia
Educational Aims
-
To describe flow (F), lung volumes (V), and respiratory pressure (P) measurements together with resistance (R) and compliance (C) measurements in neonatal respiratory diseases
4.3.1 Acute Neonatal Lung Disease
The predominant neonatal acute respiratory diseases include transient tachypnea of the newborn (TTN), respiratory distress syndrome (RDS) due to surfactant deficiency and/or structural immaturity, pulmonary interstitial emphysema (PIE), meconium aspiration syndrome (MAS), persistent pulmonary hypertension of the newborn, pneumonia, and congenital diaphragmatic hernia (CDH). As there are no reported measurements of lung mechanics or lung function for infants with PIE and pneumonia, they are not discussed further.
4.3.1.1 Transient Tachypnea of the Newborn
Transient tachypnea of the newborn (TTN) is characterized by delayed resorption of fetal lung fluid within the distal airspaces. The mechanical consequences of TTN include reduced lung volume (functional residual capacity, FRC) and consequently also decreased lung compliance (increased elastance), tidal volume, and respiratory rate (Benito Zaballos et al. 1989). Although increased interstitial fluid might be expected to increase lung tissue resistance, there are no reported studies of tissue resistance in infants with TTN.
4.3.1.2 Respiratory Distress Syndrome
Like TTN, RDS is predominantly a disease of the distal lung parenchyma. Surfactant deficiency promotes atelectasis, typically characterized by low lung volumes and reduced lung compliance. Lung function is also reflective of the degree of structural maturation, as surfactant deficiency is predominantly a disease of the premature infant.
In the intubated infant, lung resistance measures are highly variable and not reproducible (22–32 %, ICC <0.75), in contrast with less variable and more reproducible measurements of lung compliance (obtained from esophageal manometry). Hence, the clinical relevance of dynamic mechanics measurements of resistance in intubated newborn infants with respiratory distress is questionable (Gappa et al. 2006a).
Measurements of impedance, obtained using forced oscillatory mechanics (a quasistatic lung mechanics measurement), may provide a more reliable assessment of lung mechanics. Dorkin measured respiratory impedance in six paralyzed and intubated infants, three of whom also had pulmonary interstitial emphysema (Dorkin et al. 1983). The tracheal tube contributed to almost all the inertance and approximately 50 % of the respiratory system resistance in the intubated infant. After subtraction of the impedance of the endotracheal tube, resistance ranged from 22 to 34 cm H2O/L/s, compliance from 0.22 to 0.68 mL/cm H2O, and inertance from 0.0056 to 0.047 cm H2O/L/s (Gappa et al. 2006a; Dorkin et al. 1983).
The low-frequency forced oscillation technique (LFOT) evaluates impedance simultaneously across a range of frequencies (usually ~0.5–14 Hz). Fitting of the resultant impedance data to the constant-phase model permits estimation of partitioned mechanical variables of the airways and the parenchymal tissues (Hantos et al. 1992b). Using the LFOT, impedance measurements in naïve (surfactant-deficient) newborn preterm lambs showed that respiratory system resistance (R rs) and reactance (X rs) are markedly frequency dependent (Pillow et al. 2001a). Respiratory system resistance in the preterm infant is also dominated by the resistive properties of the tissues, contrasting sharply with the predominant airway contribution to respiratory system resistance in later life (Pillow et al. 2005). Although compliance is also low (increased elastance), the tissue resistance is disproportionately greater, resulting in mechanical uncoupling of the parenchyma and increased hysteresivity (ratio of tissue resistance to tissue elastance) (Pillow et al. 2001a, 2005). Increased surfactant pool size (Pillow et al. 2004a) and lung volume recruitment (Pillow et al. 2004b) both enhance mechanical coupling of the tissues, evident as a lowering of the hysteresivity ratio in the lung. Increased contribution of the tissues to respiratory system mechanics, and the associated increased frequency dependence, results in elevation of the resonance frequency of the lung (Pillow et al. 2001a). Frequency dependence also becomes less marked with increasing postnatal age (Fig. 4.11) (Pillow et al. 2005).
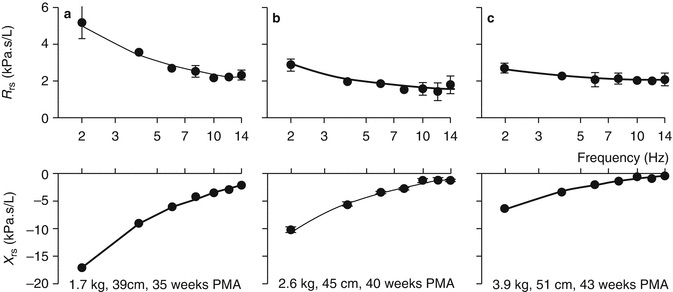
Fig. 4.11
Representative impedance spectra from two newborn infants: Panels a and b illustrate impedance spectra for a 32-week preterm infant at 35-week postmenstrual age (panel a) and on repeat measurement at 40-week PMA). Panel c shows the impedance spectra from a healthy term infant at 42-week postmenstrual age. Figure illustrates the alteration in respiratory impedance that occurs with postnatal maturation (a, b) and the marked frequency dependence of respiratory impedance in the more immature infant (a). Solid lines indicate the fit of the constant-phase model. R rs respiratory resistance (comprising frequency-dependent tissue resistance and frequency-independent airway resistance), X rs respiratory reactance (incorporating tissue elastance and airway inertance), PMA postmenstrual age. Symbols and bars are mean (SD) values from technically acceptable measurements in the same infant (Adapted from Pillow et al. (2005))
Variability in measurements of lung mechanics in newborn infants in part reflects lung maturation associated with advancing gestation: lung compliance increases 0.17 mL/cm H2O/week, to reach mean (SD) values of 2.50 (0.07) mL/cm H2O at term equivalent (Bhutani et al. 2005). Other factors include in utero fetal exposures such as placental nutrition, inflammation, antenatal steroids, and postnatal treatments including caffeine, glucocorticoids, surfactant, and ventilation modality. Early treatment with caffeine in surfactant-treated immature baboons increased C rs over the first day of life and increased ventilatory efficiency index (Yoder et al. 2005).
The initial benefit of antenatal steroids on improved lung compliance at birth fades beyond 1 week after initial exposure (McEvoy et al. 2008). There is no evidence of that antenatal steroids have a persistent effect on lung mechanics: infants born at term after exposure to up to three courses of betamethasone at gestations between 25 and 33 weeks had no difference in gas mixing or lung volume/mechanics when compared to nonexposed term controls (Hjalmarson and Sandberg 2011). Like antenatal steroids, postnatal steroids also increase respiratory system compliance (Durand et al. 1995). Low-dose and high-dose postnatal dexamethasone for CLD achieve a similar increase in C rs suggesting equal effectiveness for improvement of lung volume (McEvoy et al. 2004). Early postnatal dexamethasone may be more effective than late dexamethasone in achieving lower R aw/R rs and higher specific conductance (sG aw) (Merz et al. 1999; Vento et al. 2004), which may indicate a degree of fixed change in the airway walls in infants receiving delayed glucocorticoid treatment.
During administration, surfactant represents a fluid bolus in the airway that increases inspiratory and expiratory time constant, associated with an acute increase in respiratory system resistance. Effective delivery of surfactant to the lung thus benefits from a transient increase in the inspiratory time and potentially brief increase in the delta P (peak inspiratory pressure–positive end-expiratory pressure) to overcome the increase resistance resulting from the fluid bolus. Treatment with exogenous surfactant (da Silva et al. 1994) or increases in positive end-expiratory pressure (PEEP) during recruitment from atelectasis increases lung volume: (Dimitriou et al. 1999) lung volume increases by 61 ± 39 % within a median of 4 min after surfactant administration, with altered distribution of lung volume towards the dorsal rather than ventral compartment. The increase in lung volume after surfactant or volume recruitment maneuvers increases maximal compliance of the respiratory system, with higher tidal volumes achieved at lower pressures than required prior to treatment (Miedema et al. 2011a; McEvoy et al. 2010). Importantly, the increase in compliance also impacts on the time constant (τ = R rs × C rs) of the respiratory system. Changes in the inspiratory and expiratory time constants need to be monitored as they necessitate adjustment of the inspiratory and expiratory times and influence the maximal frequency that can be used during ventilation with passive expiration.
Compared to treatment of RDS with synchronized intermittent mandatory ventilation (SIMV), a small clinical trial showed that infants treated with high-frequency oscillatory ventilation (HFOV) have early and sustained improvement in pulmonary mechanics and higher dynamic respiratory compliance (Vento et al. 2005). The improvement in pulmonary mechanics in patients treated with HFOV is likely associated with enhanced lung volume recruitment. The neonatal lung with RDS exhibits hysteresis (Miedema et al. 2011b). Hence lung recruitment maneuvers that aim to recruit the lung and subsequently ventilate it on the most compliant part of the deflation pressure volume curve can achieve effective ventilation with minimum pressure and volume cost of ventilation. Importantly, recruitment of the lung by increasing mean distending pressure during HFOV is also associated with changes in compliance and the time constant for volume delivery during HFOV (Pillow 2012). Consequently, volume delivery can vary substantially over the time course of an HFOV volume recruitment maneuver (Miedema et al. 2012) and potentially result in rapid fluxes of the partial pressure of arterial carbon dioxide. The effect of compliance on volume delivery during HFOV is more evident at lower frequencies (Pillow 2012; Pillow et al. 2001b). The development of HFOV ventilators that incorporate volume guarantee during HFOV will provide protection from such rapid changes in ventilation during HFOV.
Infants with RDS often have their clinical course complicated by the persistence of a patent ductus arteriosus (PDA), which presents as a left–right shunt. Ligation of a PDA (left–right shunt) increased dynamic compliance by 77 % in 16 newborn infants measured before and after ligation, but did not influence dynamic R rs or mean airway pressure (Szymankiewicz et al. 2004a).
4.3.1.3 Meconium Aspiration Syndrome
Although meconium aspiration syndrome (MAS) is often considered as a high airway resistance disease due to the presence of meconium in the airways, studies in rabbits show that the acute aspiration of meconium also causes a significant reduction in the lung–thorax compliance (Sun et al. 1993). Impaired compliance after MAS is responsive to standard surfactant instillation (Sun et al. 1993). Larger volume (15 mL/kg) surfactant lung lavage is an emerging treatment for MAS: following surfactant lung lavage, dynamic compliance approximately doubled, and airway resistance nearly halved in a group of MAS infants on mechanical ventilation (Szymankiewicz et al. 2004b). These changes in dynamic respiratory mechanics were associated with a decrease in mean (SD) airway pressure from 12.4 ± 3.6 to 5.4 ± 2.1 cm H2O within 48 h after surfactant lung lavage.
Meconium appears to have a differential effect on hyperreactivity of the airways and the lung tissue. Whereas tracheal smooth muscle reactivity increases with increasing meconium concentration in response to histamine and acetylcholine, a negative correlation was observed in the lung tissue (Mokry et al. 2007). Airway hyperresponsiveness 2 weeks after birth (approximately 5 days after cessation of ECMO) is responsive to bronchodilator treatment (Koumbourlis et al. 1995) as evidenced by percent change in MEF25 and the MEF25/FVC ratio. Airway hyperreactivity after MAS is also reduced by budesonide (Mokry et al. 2007).
Limited information is available for the long-term lung function outcomes after MAS. Neonates treated with ECMO for meconium aspiration have better long-term lung function outcomes than infants with diaphragmatic hernia treated with ECMO in the neonatal period (Spoel et al. 2012a), most likely reflecting differences in lung capacity and structure. At least 50 % of children who had MAS in the neonatal period have evidence of trapped air on lung function during mid-late childhood. There are no published reports evaluating responsiveness to bronchodilators beyond infancy after neonatal MAS.
4.3.1.4 Persistent Pulmonary Hypertension of the Newborn
Persistent pulmonary hypertension of the newborn (PPHN) presents with profound hypoxia either in the absence of significant lung disease (primary PPHN) or hypoxia out of proportion with the degree of respiratory disease (secondary PPHN – complicating RDS, meconium aspiration, pneumonia, etc.). Lung function in 1-year-old infants who had PPHN and who were treated with inhaled nitric oxide (iNO) but not extracorporeal membrane oxygenation (ECMO) was compared with lung function outcomes of infants who were randomized to receive either ECMO or conventional management as part of the UK ECMO trial. V′max,FRC was lower than predicted in all three groups (p < 0.001). There were no statistical differences between the three groups in the Z-scores for V′max,FRC (Hoskote et al. 2008). Nonetheless, only 26 % of iNO-treated infants had V′max,FRC Z-scores below normal compared to 37 and 56 %, respectively, for ECMO and CM groups. A prospective evaluation of lung function in infants with PPHN treated with/without iNO was reported by Dobyns and colleagues (1999): compared to healthy control infants of the same age, there were no differences in lung volume or passive respiratory mechanics for infants with PPHN, nor was there any effect of iNO on later pulmonary function in the PPHN group. Together, these results suggest that iNO treatment does not worsen outcome compared to ECMO or conventional management.
4.3.1.5 Congenital Diaphragmatic Hernia
Infants with congenital diaphragmatic hernia have impaired airway function in the first year of life: maximal expiratory flows at FRC were a mean Z-score of −1.5 lower than healthy term infants (i.e., 1.5 standard deviations below the mean FRC for healthy term infants) with no evidence of significant change between 6 and 12 months of age (Spoel et al. 2012b). Conversely, and perhaps surprisingly, given the usual association of CDH with pulmonary hypoplasia in at least one lung, Spoel noted that measured values of functional residual capacity were relatively high (47 % fell above the normal range) (Spoel et al. 2012b). As expected, mean Z-score for FEV1 and FVC was negatively influenced by the presence of chronic lung disease, the duration of ventilation, and ECMO support (Spoel et al. 2012a). Patients with CDH develop hyperinflation, with elevated plethysmographic FRC at 1 year (Hofhuis et al. 2011) that is still evident at 8–12 years (Spoel et al. 2012a; Hamutcu et al. 2004; Majaesic et al. 2007).
Spoel showed that greater impairment was evident in infants with CDH who required extracorporeal membrane oxygenation (Spoel et al. 2012b). However, poorer outcome in ECMO-treated CDH may reflect the initial severity of disease rather than ECMO: Beardsmore showed that ECMO per se did not worsen respiratory function at 1 year of age compared to conventionally ventilated controls (Beardsmore et al. 2000).
Spoel and colleagues also noted deterioration in lung function over time (5–12 years) in patients with CDH who were treated with ECMO in the neonatal period (see Table 4.1) (Spoel et al. 2012a): mean (SE) SDS score for FEV1 after bronchodilation was higher at 5 years (−0.71 (0.40)) than at 8 years (−2.27 (0.36)) and 12 years (−2.73 (0.61)). Deterioration over time may be related to maldevelopment of the alveoli and pulmonary vessels with disturbed lung growth. It is also possible that increased susceptibility to recurrent respiratory tract infections may contribute to deterioration in lung function over time. An active lifestyle and healthy eating pattern may be especially important for children with CDH to counteract the deterioration in lung function over time, given the known positive effect of participation in sports and the negative interaction of BMI with exercise capacity (van der Cammen-van Zijp et al. 2011). Similarly, it may be especially important to treat recurrent infections of the respiratory tract and actively manage asthma to avoid limitation of exercise capacity and deterioration of lung function. Prolonged follow-up of children with CDH is warranted.
Table 4.1
Longitudinal results of spirometry after neonatal ECMO after bronchodilation
Age (year) |
5 |
8 |
12 | |||
---|---|---|---|---|---|---|
Subjects, n |
72 |
77 |
42 | |||
Bronchodilation |
Pre |
Post |
Pre |
Post |
Pre |
Post |
SDS FEV1 | ||||||
MAS |
−0.16 (−0.51, 0.19) |
0.49 (0.16, 0.82)** |
−0.49 (−0.77, −0.22)* |
0.12 (−0.16, 0.40) |
−0.69 (−1.1, −0.28)* |
0.01 (−0.45, 0.24) |
CDH |
−1.7 (−2.3, −1.2)* |
0.01 (−0.53, 0.47) |
−2.5 (−3.0, −2.0)* |
−2.3 (−3.0, −1.5)*** |
−3.2 (−4.4, −2.6) * |
−2.7 (−3.9, −1.5)* |
4.3.2 Chronic Neonatal Lung Disease
4.3.2.1 Bronchopulmonary Dysplasia
BPD is most commonly considered as the chronic respiratory condition complicating extreme prematurity and/or prolonged neonatal mechanical ventilation. Factors that independently influence the tidal flow–volume-derived indices include variations in disease severity, degree of alteration to airway and lung mechanics, and also the requirement for oxygen supplementation (Baldwin et al. 2006). There are, nonetheless, quantitative differences in tidal breathing function measured in BPD infants when compared with measurements obtained from healthy term and preterm controls. BPD infants are consistently more tachypneic than their healthy counterparts (Baldwin et al. 2006), but differences in other lung function variables are less consistent. Tidal volume (V T) is either less than (Paetow et al. 1999) or similar to (Patzak et al. 1999; Schmalisch et al. 2003) values obtained in healthy infants: V T relative to healthy infants may also decrease with advancing postnatal age (Tepper et al. 1986b), likely reflecting the altered maturational progression in lung structure and function. BPD infants have increased minute ventilation (MV). Increased MV is primarily due to increased respiratory rate rather than increases in V, in part reflecting increased dead space ventilation.
Infants with BPD have an increased work of breathing. Within the tidal flow–volume loop, increased work of breathing is evident from linear or concave expiratory limb morphology. BPD infants also have a less variable shape to the tidal flow–volume waveform, indicating that they are functioning near their maximum respiratory capability with minimal reserve capacity to adapt to changes in intrinsic (e.g., airway obstruction, infection, or aspiration) or extrinsic (e.g., cold stress) environment (Baldwin et al. 2006). Tidal flow indices are difficult to interpret in part due to opposing effects of neurorespiratory control and lung mechanics on the morphology of the tidal flow waveform. Whereas BPD infants have higher respiratory drive (quantified from the mean inspiratory flow: V T/t I where t I is inspiratory time), the ratio of the time to peak expiratory flow relative to expiratory time (t PTEF:t E) after methacholine-induced airway obstruction can either increase or decrease (Clarke et al. 1994). Normal fluctuations in V T, t I, and MV seen in healthy infants in response to alternate hypoxic–normoxic breath testing are not evident in BPD infants, indicating reduced chemoreceptor sensitivity to hypoxic stimulus (Calder et al. 1994).
Lung and chest wall mechanics of infants with bronchopulmonary dysplasia (BPD) were extensively reviewed by Gappa and colleagues (2006b). Changes are reflective of maldevelopment of the lungs and airways and remodelling of the lung parenchyma and airway walls associated with increased collagen content. In preterm infants, respiratory system compliance (C rs) increases relative to body weight over the first 2 years of life, while an initially high respiratory system resistance (R rs) decreases over the same period (Baraldi et al. 1997a). Airway walls of preterm infants are more compliant than term infants as evidenced through measurements obtained using the high-speed interrupter technique (HIT) (Henschen et al. 2006).
Following the NICHD consensus conference in 2001 (Jobe and Bancalari 2001), some investigators evaluated differences in lung function according to whether infants had mild, moderate, or severe chronic lung disease. Using the single occlusion technique, Hjalmarson and Sandberg showed that infants with severe bronchopulmonary dysplasia (born at mean 25 weeks GA) had increased specific conductance and decreased specific compliance (sC rs) compared to healthy preterm infants (born at mean 29 weeks gestation) (Hjalmarson and Sandberg 2005). There were no differences in lung mechanics between the healthy preterm infants and those with mild or moderate bronchopulmonary dysplasia. The data published by Tortorolo et al. indicate a similar trend towards lower C rs at day 7 in infants who later went on to develop mild BPD and to a greater extent in severe BPD (Tortorolo et al. 2002). Their results contrast with the findings by Shao and colleagues in infants of similar gestations that infants with BPD had decreased C rs (10.8 vs 16.0 mL/kPa/Kg), but no difference in specific sC rs (0.69 vs 0.75 mL/kPa/kg) or R rs (6.6 vs 7.3 cm H2O/mL/s) compared to non-ventilated preterm infants. Infants with BPD do not show an improvement in airway resistance (R aw, measured by plethysmography) over a 4-month period (Shao et al. 1998).
Several authors have attempted to use lung function as a predictor of BPD. The value of initial R rs for prediction of lung disease is unclear: some have found increased initial R rs is associated with respiratory outcome at 1 year (Choukroun et al. 2003; Lui et al. 2000; Snepvangers et al. 2004), whereas others observed that R rs is not predictive of BPD (Tortorolo et al. 2002). Both Lui (Lui et al. 2000) and Merth (Merth et al. 1997) showed that early RDS is not an important determinant of later lung function. Serial measurements of compliance, however, show that lower C rs values after day 5 were evident in infants who later developed severe BPD. Merth observed that BPD infants have reduced C rs at 1 year of age irrespective of RDS (Merth et al. 1997).
V′max,FRC obtained using the tidal rapid thoracic compression technique is reported consistently as being lower than healthy controls throughout the first 3 years of life, regardless of the BPD era or treatment strategies (Lum et al. 2006b). Reduced expiratory flows are indicative of abnormal structural and functional development of the airways (Fig. 4.12). Serial lung function measurements in infants with both BPD and “healthy” unsedated preterm infants indicate a decline over the first year of life, suggesting that factors other than BPD may contribute to abnormal airway function in preterm infants (Hoo et al. 2002b). Raised volume rapid thoracic compression (RVRTC) measurements show similar mild–moderately severe airflow obstruction. However the predominance of airflow obstruction primarily at low lung volumes indicates the pathology is more likely to involve the small peripheral rather than larger central airways (Lum et al. 2006b). Concurrent increased residual volume (RV), FRC, and RV/TLC (total lung capacity) ratios were more marked in infants with recurrent wheeze, indicative of a degree of hyperinflation and gas trapping. Intermediate values (between healthy controls and wheezy BPD infants) were observed for RV, FRC, and RV/TLC in non-wheezy preterm infants (Robin et al. 2004), likely reflective of abnormal airway growth or the effects of preterm delivery. Forced deflation from FRC showed that preterm infants with developing BPD have severe lower airway obstruction as early as 3–4 weeks postnatal age. Increased flows and FVC following bronchodilation indicate that reopening of obstructed airways is achieved with bronchodilators (Motoyama et al. 1987).
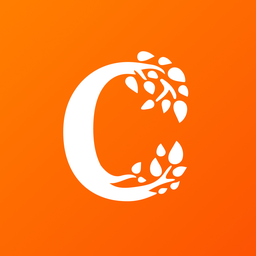
Full access? Get Clinical Tree
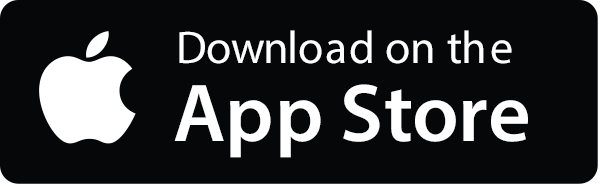
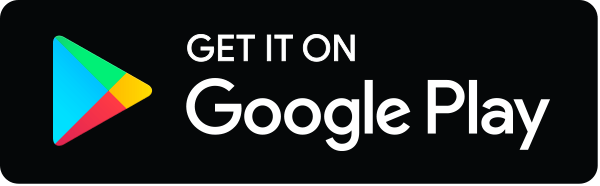