Congenital heart disease is the most common cause of infant death in the United States. In most children, serious heart disease presents during the first month of life. Thus, care for newborns demands an awareness of the prevalence of cardiac defects and the ability to screen appropriately to differentiate infants with cardiac disease from other critically ill newborns. In 2000, the prevalence of congenital heart disease found by a population study in Quebec was 11.89 per 1000 among children and 5.78 per 1000 in the general population. By contrast, the prevalence of severe congenital heart disease was 1.45 per 1000 children and accounted for 12% of all congenital cardiac lesions in children. Advances in fetal and neonatal ultrasonographic screening allow identification of an increasing number of children with congenital heart disease and, more importantly, appropriate counseling for their parents. As many as 60% of newborns admitted to large pediatric cardiac intensive care units have an established diagnosis at the time of birth. Although some studies indicate an important benefit of fetal diagnosis in terms of morbidity and mortality, the unintended result is that in some centers postnatal acute presentations of congenital heart disease are becoming more rare, and this may be detrimental by assuaging fears of congenital heart disease in symptomatic newborns and thus causing delay in care and potentially increased morbidity and mortality. In a large study of neonates in the United Kingdom, up to 25% of children with congenital heart disease were not diagnosed until after discharge from the newborn nursery, and misdiagnosis was found to occur in up to 7 per 100,000 live births in the United States.
Careful evaluation of the history, physical examination findings, laboratory data (including the response of upper and lower body blood gas concentrations and arterial oxygen saturation in an enriched oxygen environment), radiographic findings, and results of electrocardiography, echocardiography, and occasionally additional imaging such as cardiac computerized axial tomography (CAT), magnetic resonance imaging (MRI), or invasive cardiac catheterization allows the physician to delineate the specific congenital cardiac defect. When a neonate suspected of having congenital heart disease is being examined, it is important to frame physical and laboratory findings within the context of the transition from fetal to neonatal circulation. This chapter considers the physiology and pathophysiology of the fetal and neonatal cardiovascular systems in newborns with and without heart disease.
Physiology and Pathophysiology
Fetal and persoens Neonatal Circulations
Newborns undergo dramatic changes in the pulmonary and systemic circulations following birth. In the fetus, oxygen-rich blood from the placenta reaches the right atrium via the umbilical vein and shunts preferentially across the foramen ovale to the left ventricle, providing increased oxygen content to the myocardium and brain ( Fig. 15-1 ). A smaller portion of the highly oxygenated blood passes from the right atrium to the right ventricle and bypasses the lungs via the ductus arteriosus to flow to the lower body. Because of this parallel circulation, individual organs may receive blood from both ventricles, and thus the output of the fetal heart is expressed as combined ventricular output (CVO). Advances in fetal ultrasonographic techniques have enabled a more accurate determination of human fetal blood flow than was previously available using fetal lamb studies. In the normal human fetus, CVO has been documented by echocardiographic studies to be 450 mL/kg/min.

Approximately 45% (200 mL/kg/min) of this blood perfuses the placenta for oxygen uptake and returns via the umbilical veins. Approximately one half of umbilical venous return enters the inferior vena cava directly through the ductus venosus, thereby bypassing the hepatic microcirculation; the remainder passes primarily through the left lobe of the liver and enters the inferior vena cava via the left hepatic vein. Although venous returns from the lower body, ductus venosus, and hepatic veins all pass into the thoracic inferior vena cava, these streams do not mix completely. There is preferential streaming via the inferior vena cava into various cardiac chambers, with blood from the ductus venosus and left hepatic veins passing preferentially across the foramen ovale into the left atrium. Oxygenated blood crossing the foramen ovale comprises 76% of the left ventricular output and 33% of combined cardiac output supplying the cerebral and myocardial circulations. Thus, the streaming of blood from the inferior vena cava to the left heart increases the efficiency of oxygen delivery to the most actively metabolizing area of the fetus, the brain. Venous drainage from the right hepatic veins and the abdominal inferior vena cava tends to stream preferentially to the right atrium and right ventricle. Similarly, desaturated superior vena caval return from the cerebral circulation is preferentially directed to the right ventricle through the tricuspid valve. The right ventricle ejects much of this relatively undersaturated blood via the ductus arteriosus back to the placenta. The remainder of the right ventricular output passes into the pulmonary vascular bed. The proportion of CVO to the lungs increases throughout gestation by 60% from 20 to 38 weeks, with pulmonary blood flow accounting for 11% of the CVO. Thus, although the circulations are not completely separated, each ventricle primarily performs its postnatal function—the left ventricle delivers blood for oxygen utilization, the right for oxygen uptake.
The left and right ventricles do not eject similar volumes in the fetus. The right ventricle has been shown to be dominant in fetal lamb studies, ejecting almost twice the blood volume ejected by the left ventricle. In human fetuses, the stroke volume of the right ventricle is approximately 28% greater than that of the left ventricle. Left ventricular output is distributed mainly in the upper body, including the brain (approximately 20% of CVO) and the myocardium (3%); the remainder (about 10%) crosses the aortic isthmus to the lower body.
In the fetus, the ductus arteriosus is part of a specialized system of oxygen-sensitive organs and tissues in the body. Blood flow across the ductus arteriosus is 78% of the right ventricular cardiac output and 46% of the CVO. In comparison with the aorta and the pulmonary arteries, it is thicker walled, with a medial layer composed of longitudinal and spiral layers of smooth muscle fibers within concentric layers of elastic tissue and an intimal layer of smooth muscle and endothelial cells. Continued patency of the ductus arteriosus throughout gestation is controlled by the relatively low fetal oxygen tension and the inhibition of procontractile mechanisms by vasodilators. The vasodilators primarily responsible for ductal patency include prostaglandin and prostacyclin, which interact directly with ductal prostanoid receptors, and to a lesser extent carbon monoxide and nitric oxide.
Fetal Echocardiography
The increased availability and use of fetal echocardiography has significant clinical implications postnatally. All echocardiographic techniques (see the later section on echocardiography of the newborn) can be used to produce a fetal echocardiogram. A fetal study is usually performed between 18 and 22 weeks’ gestation for optimal imaging. Studies can be carried out earlier in gestation but may need to be repeated to clarify the diagnosis or follow disease progression. A fetal study can be prompted by either maternal or fetal indications ( Box 15-1 ).
Maternal indications | Fetal indications |
---|---|
Family history of congenital heart disease Metabolic disorders Exposure to teratogens Exposure to prostaglandin synthase inhibitors Rubella infection Autoimmune disease (systemic lupus erythematosus, Sjögren syndrome, etc.) Familial inherited disorders (Marfan syndrome, Noonan syndrome, etc.) In vitro fertilization | Abnormal obstetrical ultrasound screen Extracardiac abnormality Chromosomal abnormality Arrhythmia Hydrops Increased first-trimester nuchal translucency Multiple gestation and suspicion of twin-twin transfusion syndrome |
Not only can fetal echocardiography be used to diagnose cardiac anatomic defects, but analysis of fetal blood flow patterns in the ductus venosus, umbilical artery, and umbilical vein can provide information on the overall cardiovascular well-being of the fetus. Fetal echocardiography may also have prognostic implications in infants with congenital diaphragmatic hernia and twin-twin transfusion syndrome (TITS). From a cardiac standpoint, fetal echocardiography can accurately diagnose most forms of congenital heart defects and track their evolution in utero ( Fig. 15-2 ). This may improve postnatal morbidity and mortality, especially in newborns with ductal-dependent cardiac defects. The level of parental psychological stress, however, appears to be related to the severity of the cardiac defect and not to a prenatal versus postnatal diagnosis. Another recent use of fetal echocardiography is in conjunction with interventional techniques. Interventions have been reported in fetuses with hypoplastic left heart syndrome and restrictive atrial septa, severe aortic stenosis, and pulmonary stenosis or atresia. Fetal echocardiography is essential for assisting in these procedures.

Fetal Heart Failure
The parallel nature of the fetal circulation makes it uniquely equipped to tolerate most structural abnormalities of the heart that are life threatening after birth. Even in cases of significant obstruction and falling unilateral ventricular output, the unaffected side of the heart is able to increase its output and fetal blood flow is redistributed so that the unaffected ventricle can produce most, if not all, of the cardiac output. As a result, cardiac shunts, pulmonary overcirculation, and anatomic causes of systemic hypoperfusion generally do not become noteworthy until the infant is born. There are a limited number of cardiovascular causes of fetal distress that may result in the development of hydrops, including arrhythmias, myocardial disease, severe atrioventricular (AV) or semilunar valve insufficiency, and premature constriction of the ductus arteriosus with a restrictive atrial septum. All of these conditions share a final common pathway of elevated ventricular end-diastolic pressure, increased atrial and central venous pressure, and movement of fluid from the capillary bed into fetal tissue.
Sustained tachyarrhythmias (over 200 beats per minute) or bradycardia associated with congenital heart disease are not well tolerated. Tachyarrhythmias may be controlled by administering antiarrhythmic medications to the mother, including digoxin, β-blockers, sotalol, flecainide, and amiodarone. Digoxin is a first-line therapy for short ventriculoatrial supraventricular tachycardia and atrial flutter in the absence of hydrops and is associated with an 80% to 85% success rate in the treatment of fetal supraventricular tachycardia and a 60% to 65% success rate for atrial flutter. Sotalol is another first-line therapy with a 72% conversion rate. Arrhythmia control is likely to be successful if treatment is initiated before the development of hydrops. Successful treatment of supraventricular tachyarrhythmias in the presence of hydrops is more complex and typically requires the use of at least two medications and a longer therapeutic course. Incessant fetal supraventricular tachycardia warrants an attempt at conversion with intraumbilical administration of antiarrhythmic medications. Even with successful treatment, there is an 8% to 30% reported risk of fetal or neonatal mortality.
Fetal bradycardia may be associated with significant intrauterine mortality. AV block is caused by maternal autoantibodies in approximately 45% to 48% of cases and can present as early as 18 weeks’ gestation. There have been reports of successful treatment of early low-grade heart block, with improvement in outcomes, by administration of fluorinated steroids to the mother. β-Sympathomimetic agents, intravenous immunoglobulins, and direct fetal pacing are also being used as therapies for autoimmune-mediated fetal AV heart block. Another 45% to 48% of fetal AV block is associated with structural heart disease (most commonly left atrial isomerism and congenitally corrected transposition of the great arteries). Unfortunately, even with aggressive intervention, survival of infants with AV block associated with structural heart disease is particularly dismal, with a 75% to 90% incidence of fetal or neonatal demise.
Structural heart disease is the most common cause of fetal heart failure and may lead to significant mortality without intervention. Some fetuses with severe semilunar valve stenosis are candidates for an attempt at intrauterine valvuloplasty to stabilize the patient’s condition and ideally improve long-term surgical options. Early delivery with immediate intervention is often the best management strategy for fetuses who show symptoms associated with specific structural heart defects such as ductus arteriosus restriction, Ebstein anomaly of the tricuspid valve, or tricuspid valve dysplasia with significant tricuspid insufficiency.
Circulatory Changes after Birth
By the end of the third trimester, the fetal pulmonary circulation begins to demonstrate vasoreactivity and responsiveness to maternal hyperoxygenation. The onset of breathing produces a dramatic increase in pulmonary blood flow (from 11% of the CVO to a full cardiac output) and a decrease in pulmonary vascular resistance. With the onset of ventilation, air replaces intraalveolar fluid and local oxygen concentration increases markedly, both of which may directly dilate the pulmonary vascular smooth muscle or cause the release of vasodilating substances. Bradykinin, a potent pulmonary vasodilator, is released when the lungs are exposed to oxygen; bradykinin, in turn, stimulates endothelial cell production of nitric oxide, a potent vasodilator. Prostacyclin (prostaglandin I 2 ), a pulmonary vasodilator derived from the metabolism of arachidonic acid, is released when the lung is mechanically ventilated (not necessarily oxygenated) or exposed to other vasoactive substances such as bradykinin or angiotensin II. Inhibiting prostaglandin production by administering a cyclooxygenase inhibitor (such as indomethacin) attenuates the normal ventilation-induced decline in pulmonary vascular resistance, which further supports the role of these vasoactive substances in the establishment of a normal pulmonary circulation after birth. The increase in pulmonary blood flow greatly alters the venous return to the left atrium and consequently the left ventricular preload. Left ventricular cardiac output increases from an estimated 179 mL/min/kg to approximately 240 mL/min/kg within the first 2 hours after birth due to increased stroke volume. As the ductus arteriosus closes and the left-to-right shunt diminishes, left ventricular output falls to approximately 190 mL/min/kg.
The initial dramatic decrease in pulmonary vascular resistance is secondary to relaxation of the resistance vessels. There is then a slow, progressive decline over the next 2 to 6 weeks of life as these pulmonary arterioles remodel from their fetal pattern, in which a large amount of smooth muscle is present in the medial layer, to the adult pattern, with very little muscle in the media. The development of the “physiologic anemia” that normally occurs during this time decreases the viscosity of the blood perfusing the lungs, which decreases shear stress and also contributes to the overall decrease in pulmonary vascular resistance.
Closure of the Foramen Ovale
Functional closure of the foramen ovale occurs after the placenta is removed from the circulation. With clamping of the umbilical cord, blood flow through the inferior vena cava to both atria decreases dramatically. Initiation of breathing increases blood flow through the pulmonary bed to the left atrium. These changes result in a left atrial pressure that now exceeds the right atrial pressure, which causes the valvelike flap of the foramen ovale to close. Although functional closure of the foramen ovale occurs in most infants, anatomic closure is not always complete. As a result any action that raises right atrial pressure to the point that it equals or exceeds left atrial pressure can result in a right-to-left shunt across the foramen ovale. Likewise, in conditions that have large left-to-right shunts, such as a large patent ductus arteriosus (PDA) or ventricular septal defect (VSD), the left atrium may become dilated, which results in stretching of the atrial septum and incompetence of the foramen ovale and a left-to-right shunt. In many adults, despite the events leading to functional foramen closure, a probe-patent foramen may persist.
Closure of the Ductus Arteriosus
Closure of the ductus arteriosus is a more complex phenomenon. The media of the ductus arteriosus contains smooth muscle in a spiral configuration that is maintained in a relaxed state, primarily by the action of prostaglandins. Constriction and closure after birth reflect removal of the stimuli that maintain relaxation and the addition of factors that produce active constriction. Circulating prostaglandin E 2 (PGE 2 ) concentrations in the fetus are high because of the very low pulmonary blood flow. With clamping of the placenta, the source of prostaglandin E 2 is removed. With inspiration, there is a dramatic increase in pulmonary blood flow and an abrupt increase in oxygen tension that inhibits ductal smooth muscle voltage-dependent potassium channels and results in an influx of calcium and ductal constriction. In addition, the remaining prostaglandin E 2 (is almost completely metabolized as it passes through the pulmonary circulation, which results in a rapid decrease in serum levels of prostaglandin E 2 and allows active constriction of the ductus arteriosus to be unopposed. The ductus arteriosus constricts rapidly; in mature infants, functional closure generally occurs within 10 to 15 hours after birth. Permanent closure by intimal cushion formation, intimal proliferation, fibrosis, and thrombosis may take several weeks.
Myocardial Performance and Cardiac Output
Fetal myocardium differs from neonatal and adult myocardium in several important ways. The primary fuel for fetal myocardium is almost exclusively glucose in contrast to the adult heart, which can use fatty acids as a significant energy source. In addition, especially early in gestation, the fetal heart grows by hyperplasia of the myocardial cells in contrast with the newborn heart, in which the myocardium grows by hypertrophy. Fetal myocardium also functions distinctly by developing less active tension for a given stretch than does adult myocardium and therefore has less ability to contract. Thus, ventricular output can be increased only modestly by volume loading and only at relatively low atrial pressures; unlike in the adult, output increases only slightly at levels more than 2 to 4 mm Hg above baseline. Inotropic stimulation of the fetal myocardium also increases cardiac output relatively little. This inability to respond to changes in preload and in the inotropic state is related in part to immaturity of muscle structure. Early in gestation, there are relatively fewer contractile elements, an immature sarcoplasmic reticulum with lower affinity for calcium binding and a some what chaotic overall arrangement of fibers with considerable interstitial tissue. Toward term, more contractile elements are present, and these are more mature and are arranged in a more orderly fashion. Another factor limiting fetal myocardial performance is incomplete sympathetic innervation, which limits response to inotropic stimulation. The fetus is best able to increase ventricular output by increasing its heart rate. There is a linear relationship between ventricular output and heart rate up to about 250 beats per minute; thereafter, ventricular output reaches a plateau and even starts to decline. As heart rate goes below the normal range, ventricular output decreases dramatically because of the limited ability to increase stroke volume. As a result the fetal myocardium is operating under a high-volume load and responds poorly to any increase in ventricular afterload. Although adrenergic support can be supplied either by circulating catecholamines or through neural pathways, the overall quantitative response may be less than in the adult. The control of cardiac rate and the distribution of cardiac output are the major mechanisms for maintenance of circulatory function.
As discussed previously, the left ventricular cardiac output increases dramatically within the first 2 hours after birth and remains elevated for several weeks before decreasing to approximately 190 mL/min/kg. Because of the high resting values in the period immediately after birth, ventricular output can be increased only modestly by volume loading or inotropic stimulation with dopamine and dobutamine. The neonatal myocardium remains sensitive to the administration of the calcium ion and responds positively to the lusitropic effects of milrinone. After the initial newborn period, resting values decrease progressively, and ventricular output can be increased much more effectively.
Normal Physiologic Data in the Newborn
The normal physiologic values in the heart and great vessels are shown in Figure 15-3 . Pulmonary arterial pressures in the newborn are variable but generally decrease to half of systemic pressure within 8 to 12 hours and to one third of systemic pressure within a day or so. Over the next 4 weeks, there is a further slow, progressive decline to adult levels.

The oxygen saturation on the right side of the heart is approximately 60% to 70%; that on the left side of the heart is 92% to 95%. The oxygen saturations may be used to determine the direction of shunting within the heart or great vessels. For example, an increased saturation in the right atrium suggests a left-to-right shunt at the atrial level; a decreased saturation in the left atrium in a neonate with otherwise healthy lungs indicates a right-to-left shunt at the atrial level.
Physical Factors that Control Blood Flow
Flow ( Q ) through a vascular bed is governed by the resistance to flow ( R ) and the pressure decrease across the bed (Δ P ) (Ohm’s law).
Furthermore, by application of Poiseuille’s law, resistance to flow is directly related to the viscosity of the blood and inversely related to the cross-sectional area of the bed (radius).
An appreciation of the general relationship of pressure, resistance, and flow is important in understanding the pathophysiology and natural history of various congenital heart defects.
Blood flows where resistance is least.
Vascular resistance is calculated from the formula
For the systemic circulation, the Δ P (pressure decrease) is systemic arterial pressure (SAP) minus systemic venous pressure (SVP); for the pulmonary circulation, the Δ P is pulmonary arterial pressure (PAP) minus pulmonary venous pressure (PVP).
If the pressure decrease is measured in millimeters of mercury and the flow is measured in liters per minute per square meter, then the calculated vascular resistance is considered in resistance units. One resistance (or Wood) unit is equal to 80 dyne • sec/cm 5 . The maximum normal PVR is 2.5 to 3 units, and the maximum normal SVR is 15 to 20 units.
Peripheral vascular resistance is not the only type of resistance that will affect flow. For example, a narrowed valve provides more resistance to blood flow than does a wide open valve; a small VSD provides more resistance to blood flow than does a large VSD; and a thick, noncompliant ventricular chamber provides more resistance to blood flow than does a thinner, more compliant ventricular chamber.
If two similar cardiac chambers or arteries (one left-sided or systemic and the other right-sided or pulmonary) communicate with each other and the opening between them is so large that there is little or no resistance to blood flow, the defect is considered nonrestrictive. The pressures on each side of the opening are fully transmitted and approximately equal. If the opening is small ( restrictive ), there is resistance to blood flow, and the pressures are not fully transmitted. In the presence of a nonrestrictive defect, the resistances to outflow (downstream resistance) from each of the two communicating chambers determine the direction of blood flow. For example, with a large VSD in which ventricular pressures are equal, pulmonary vascular resistance is usually lower than systemic vascular resistance, and pulmonary blood flow is greater than systemic blood flow; that is, a left-to-right shunt is present ( Fig. 15-4 , A ). Because the flows and shunting pattern depend on the relationship of the downstream pulmonary and systemic vascular resistances, these are called dependent shunts. When the two resistances are equal, no shunt occurs (see Fig. 15-4 , B ). When resistance to outflow of the right ventricle exceeds that of the left ventricle (see Fig. 15-4 , C )—as might occur with the development of pulmonary vascular disease or, more commonly, when there is an associated pulmonic stenosis (tetralogy of Fallot)—right-to-left shunting is present. When there is a communication between the two sides of the heart at different anatomic levels (e.g., arteriovenous malformation, left ventricular–right atrial communication), the pressure difference between the two chambers or vessels, rather than downstream resistance, dictates the magnitude of the shunt; these are called obligatory shunts. For example, in a left ventricular–right atrial shunt, blood shunts continuously through the defect because the left ventricular pressure is always higher than right atrial pressure, regardless of the distal pulmonary and systemic vascular resistances.

It is customary to relate the cardiac outputs and pressures in each side of the heart. Thus, if there is three times as much flow into the pulmonary artery as into the aorta, there is a 3 to 1 pulmonary-to-systemic flow ratio. If the pressure in the pulmonary artery is 60 mm Hg and that in the aorta is 90 mm Hg, pulmonary hypertension is at two thirds of the systemic level.
Physical Examination
It is imperative to perform a complete physical examination of all neonates and to monitor for ongoing changes suggestive of pathologic conditions. Some studies have begun to advocate using pulse oximetry to screen all newborns. In 2009 the American Academy of Pediatrics and the American Heart Association issued a scientific statement regarding the usefulness of pulse oximetry in clinical practice. The statement authors concluded that additional studies were needed before the use of pulse oximetry in all newborns could be recommended as a standard of care. In an infant who is not transitioning in a normal fashion, however, pulse oximetry may be used in concert with physical examination findings to suggest the need for supplementary testing.
The neonate with congenital heart disease must be differentiated from infants with other acute illnesses. Likewise, an infant with known heart disease remains susceptible to other disease processes, including bacterial sepsis, anemia, and pulmonary disease. Acutely ill infants may have several simultaneous working diagnoses while caregivers are stabilizing their physiologic condition. In these instances, some additional factors may suggest cardiac abnormalities. Dysmorphic or syndromic features in any neonate should prompt the clinician to pay particular attention to the cardiovascular system as the infant transitions to postnatal life.
Cyanosis
Central cyanosis indicates a reduced arterial blood oxygen saturation and is generally visible when reduced hemoglobin in the blood exceeds 5 g/dL (saturation equal to or below 85% in patients with a normal hemoglobin level). If the lungs are functioning normally, the level of systemic arterial blood oxygen saturation depends entirely on the effective pulmonary blood flow—that is, the amount of blood oxygenated by the lungs that subsequently passes into the systemic arterial circulation. Neonates frequently experience acrocyanosis that is unrelated to heart disease. The best method of assessing for central cyanosis in an infant is to look at the tongue. Other diagnostic procedures are needed to determine the cause of the cyanosis. Comparing arterial blood oxygen saturation or P o 2 above and below the ductus arteriosus may be beneficial. In addition, the “hyperoxia” test, or ventilation with a high inspired oxygen concentration, is frequently considered a valuable diagnostic tool. An increase of more than 50 mm Hg (and often much higher) in systemic arterial P o 2 is seen in infants with primary pulmonary problems (especially because high levels of oxygen tend to dilate the pulmonary arterioles and decrease the pulmonary arterial pressures). A Pa o 2 of less than 100 mm Hg in an enriched oxygen environment indicates congenital heart disease until proven otherwise and should prompt an echocardiographic examination and the initiation of prostaglandin E 2 therapy. Frequently the practicalities adhere less to the textbook: a significant increase in systemic arterial blood oxygen tension or saturation can occur in cyanotic heart disease as long as effective pulmonary blood flow is reasonable. Therefore, a Pa o 2 of 100 to 250 mm Hg may be suggestive of congenital heart disease and warrants additional investigation. A Pa o 2 above 250 mm Hg makes life-threatening cyanotic heart disease less likely. If a hyperoxia test is performed, direct oxygen measurements should be made simultaneously in the upper and lower body to exaggerate any potential difference and thus help to delineate the presence of a right-to-left ductal shunt. The measurement of upper and lower body saturation during crying can also help to exaggerate potential differences due to ductal shunting.
Respiratory Distress
Most infants with mild arterial oxygen desaturation are tachypneic because of chemoreceptor stimulation but show little or no respiratory distress. Congenital heart disease that presents with respiratory distress is very difficult to diagnose in infants: their symptoms are usually more insidious and less dramatic than cyanosis, the differential diagnosis is broader, and the yield of heart disease is much less, which lowers one’s index of suspicion. When a physician sees a newborn lying in a crib, blue and comfortable, the diagnosis is almost always cardiac disease; when the infant is acyanotic, tachypneic, and showing retractions, it is usually not. Infants with respiratory distress usually have modest degrees of systemic blood oxygen desaturation, depending on the type of circulatory derangement and the severity of pulmonary edema. The respiratory distress is related to decreased lung compliance in these patients, and interstitial fluid is usually present. Thus, even with a normal separation of circulations, some degree of hypoxemia is present.
Cardiac Examination
Palpation
The cardiac impulse should be palpated. Obvious cardiac malposition in the right chest should be documented and warrants additional evaluation. The right ventricular impulse is dominant in a newborn.
Auscultation
Heart sounds in most newborns with significant congenital heart disease are abnormal. The rapid heart rates of most neonates can make this determination difficult even for the experienced practitioner. Multiple studies have assessed the skill of pediatric cardiologists and general pediatricians in detecting heart disease in inpatient neonatal settings and have found sensitivity rates of 80% to 83% for congenital heart disease. A single second sound after the first 12 hours may indicate pulmonary atresia or transposition of the great arteries. The presence of a pulmonary systolic ejection click may be normal in the first hours, but after that any systolic ejection click is abnormal, indicating an abnormal pulmonary or aortic valve, an enlarged pulmonary artery or aorta, or truncus arteriosus. If the infant has pulmonary disease without congenital heart disease and has a narrowly split or single second sound, then a high pulmonary vascular resistance is expected. Although systolic murmurs are more easily distinguished than the first and second heart sounds, they are common during the neonatal period and have historically been reported in up to 60% of healthy newborns. Several recent studies have demonstrated a higher incidence of congenital heart disease in infants with murmurs in the neonatal period. In a study of 7204 consecutively examined newborn infants, fewer than 1% of the neonates were found to have cardiac murmurs. All neonates with cardiac murmurs were referred for echocardiography, and an underlying cardiac malformation was diagnosed in 54% of them. A retrospective review of 20,323 live births over a 3-year period in a hospital in Israel found 170 newborns with an isolated finding of a heart murmur who were referred for echocardiography. Of those infants, 147 (86%) were found to have structural heart defects, including isolated VSD (37%), PDA (23%), and combined VSD and PDA (7%); abnormalities creating left-to-right shunts comprised 66% of the diagnoses. Thus, although any murmur noted in the neonatal period should prompt the practitioner to be suspicious of congenital heart disease, certain murmurs are nearly diagnostic. An S 4 gallop is always abnormal.
Abdominal Examination
Hepatomegaly is a nonspecific finding that may be indicative of increased central venous pressures. It can be associated with cardiac lesions that volume-load the right side of the heart (such as a systemic arteriovenous malformation, anomalous pulmonary venous return, or right-sided valve insufficiency as seen with Ebstein anomaly and absent pulmonary valve syndrome). Hepatomegaly may also be associated with low-output states such as myocarditis, cardiomyopathy, and tachyarrhythmias.
Peripheral Examination
It is imperative to palpate femoral and radial pulses in all newborns. Bounding pulses are typically associated with a widened pulse pressure and may be found in congenital abnormalities with increased diastolic pulmonary blood flow. Diminished femoral pulses or brachial-femoral delay may be associated with coarctation of the aorta, hypoplastic left heart syndrome, or an interrupted aortic arch. Inability to palpate pulses in all extremities should prompt the examiner to palpate the right carotid or temporal artery. If these pulses remain intact, the diagnosis would include an aberrant subclavian artery in addition to aortic arch obstruction. If all pulses are severely diminished, the obstruction to blood flow is at the aortic valve or within the left ventricle. Diminished femoral pulses should prompt the clinician to initiate therapy with prostaglandin infusion while awaiting confirmatory imaging or transfer to another center.
Imaging of the Neonate
Accurately diagnosing the anatomy and physiology of the cardiac defect is a cornerstone of pediatric cardiology. To accomplish these goals, multiple imaging modalities are available. Currently, the most frequently used techniques for imaging the newborn are echocardiography, CAT scans, MRI, and cardiac catheterization. The decision to perform one or more of these studies will depend on the history, clinical examination findings, and clinical course of the patient. With the use of these imaging techniques, a complete assessment of the cardiac defect can be obtained to aid in achieving an optimal outcome.
Echocardiography
Echocardiography remains the main imaging technique in pediatric cardiology. An echocardiogram is likely the first and possibly the only cardiac image that will be obtained to rule in or rule out a cardiac abnormality ( Box 15-2 ). Most clinical and surgical decisions can be made purely from the echocardiographic examination. Advantages of echocardiography include its portability, noninvasive nature, provision of real-time information, and overall ease of use. Disadvantages of echocardiography include limitations in image quality in some clinical circumstances and only fair ability to accurately diagnose certain complicated extracardiac vascular anomalies.
Syndromes Trisomy 13 Trisomy 18 Trisomy 21 CHARGE association VACTERL association DiGeorge syndrome Noonan syndrome Turner syndrome William syndrome |
Nonsyndromes Congenital diaphragmatic hernia Omphalocele Midline abnormality workup Tracheoesophageal fistula |
Other Unexplained hypoxemia Abnormal findings on cardiac examination Perinatal asphyxia Persistently positive blood culture results Genetic abnormalities not otherwise specified |
Echocardiography is the application of ultrasound to obtain an image via reflected sound waves. Higher-frequency probes, usually 5 MHz or higher, are used in the newborn since minimal penetration of the sound wave into the patient is needed and the shorter wavelengths allow clearer resolution of the images. Scatter of the ultrasonic wave, which inhibits image quality, usually occurs between interfaces of material that have different densities. Bone and air, when adjacent to soft tissue or fluid, produce significant artifacts, whereas soft tissue next to fluid produces minimal artifacts. For this reason, clinical conditions such as pneumothorax or bronchopulmonary dysplasia may reduce image quality.
A complete and standardized approach is needed for the initial echocardiographic evaluation of the neonate. In the usual examination the probe is placed just to the left of the sternum to obtain parasternal long ( Fig. 15-5 ) and short axial images ( Fig. 15-6 ), at the apex of the heart to obtain an apical four-chamber view ( Fig. 15-7 ), under the xiphoid process to obtain subcostal coronal ( Fig. 15-8 ) and sagittal images ( Fig. 15-9 ), and in the suprasternal notch to obtain suprasternal long ( Fig. 15-10 ) and short axial views ( Fig. 15-11 ). More views may be obtained depending on the complexity of the anatomy. If the heart is displaced as in the case of dextrocardia or congenital diaphragmatic hernia, adjustments of probe position are required. Numerous sweeps with application of M-mode, two-dimensional analysis, Doppler, and color Doppler allow for an inclusive examination that will give anatomic, physiologic, and functional data. For a complete review the reader is referred to recent textbooks on echocardiography in pediatric heart disease. ,





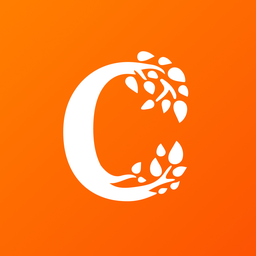
Full access? Get Clinical Tree
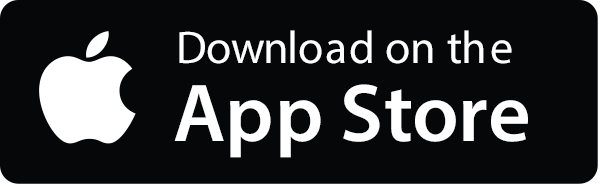
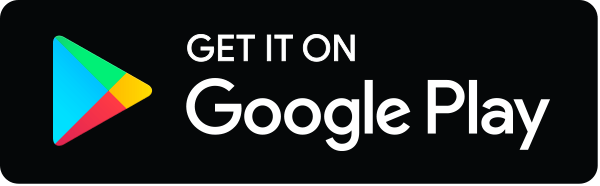