Single-gene disorders can cause perinatal mortality or severe permanent morbidity. Intrauterine gene therapy seeks to correct the genetic defect in the early stages of pathogenesis through delivery of a vector system expressing the therapeutic transgene to the fetus. Advantages of intrauterine gene therapy include prevention of irreversible organ damage, potentially inducing central tolerance and wider bio-distribution, including the brain after delivery of vector. Already, proof-of-cure has been demonstrated in knockout animal models for several diseases. Long-term outcomes pertaining to efficacy and durability of transgene expression and safety are under investigation in clinically relevant non-human primate models. Bystander effects in the mother from transplacental vector trafficking require further assessment. In this chapter, we discuss the candidate diseases amenable to intrauterine gene therapy, current state-of-the-art evidence, and potential clinical applications.
Intrauterine gene therapy and potential recipients
Intrauterine blood transfusion for the treatment of haemolytic disease of the newborn, pioneered by Liley et al. more than half a century ago, has revolutionised the field of fetal medicine. Similar in-utero therapeutic interventions for the purpose of correcting an anomaly or condition in which the fetus is at risk of intrauterine death or irreversible organ damage are now part of medical practice. Technological advances have facilitated interventions such as the placement of shunts to relieve fluid pressure, use of occlusive tracheal balloons to ameliorate pulmonary hypoplasia and, more recently, surgical correction of meningomyelocoele to mitigate neurological damage. These interventions have transformed outcomes in fetal medicine and have driven the agenda for early, but potentially curative, intrauterine interventions in preference to palliative postnatal care for a range of disorders. The next frontier that can potentially revolutionise fetal medicine is early correction of pathology at a molecular level using gene-transfer technology, with the initial focus being single-gene defects that collectively affect 1 in 200 births and contribute to a significant proportion of deaths globally. Success with these inherited disorders will clearly have wider and profound implications.
The intrauterine gene therapy concept
The prospect of correcting a genetic disorder at the molecular level and at a sufficiently early stage in its pathogenesis is highly desirable to reduce the risk of permanent organ damage and death. This major objective of intrauterine gene therapy (IUGT) is to deliver an engineered functional gene (‘transgene’) to the genetically abnormal cell using a vehicle (often a viral vector), with the aim of restoring normal cellular function. Intrauterine gene therapy in the pre-immune stage of development offers particular advantages, which include the possibility of inducing fetal tolerance to the transgenic protein, ease of transduction, wider biodistribution of vector, and the need for relatively low vector doses. These are discussed below.
The possibility of inducing fetal tolerance to the transgenic protein
The possibility of inducing fetal tolerance to the transgenic protein is a theoretical consideration as, in human fetuses, the recognition of self and foreign antigens develops at 12–14 weeks of gestation (0.3–0.4 G), with the emergence of regulatory T cells in the periphery, and immunity continues to mature as the fetus develops. Therefore, the ideal time for IUGT would be before 12 weeks, which would require significant planning of the pregnancy. It is unlikely that even early IUGT would lead to tolerance to the viral capsid and envelope protein, as degradation after gene transfer would prevent continued presentation of these proteins to T regulatory cells; however, this needs to be formally established.
Ease of transduction
Fetal tissue itself is more receptive to transduction than neonatal tissue. Studies in central-nervous-system-directed gene therapy carried out in rodents and non-human primates (NHP) show a marked shift in the efficacy of neuronal transduction, with fetal IUGT recipients demonstrating robust expression in neurones, whereas neonatal and juvenile recipients demonstrated comparatively less neuronal marking and predominantly glial expression, possibly related to differential receptor expression in developing tissues.
Wider biodistribution of vector
Wider biodistribution of vector occurs because protective tissue barriers such as the blood–brain barrier of the central nervous system (CNS) are more permissible to small molecule passage in early development.
The need for relatively low vector doses
Efficient transduction may be achievable at lower starting doses because of the ability with IUGT to achieve higher tissue concentrations of vector than would be possible after gene transfer at later gestation or in the postnatal period, when target organ size will have increased substantially. As expression is dependent upon the proportion of target cells transduced, the advantage here is that relatively small quantities of vector will be required for adequate protein expression, easing the pressure on vector production facilities as well as reducing the overall cost of the procedure.
Thus, IUGT may be more likely to succeed at phenotypic rescue than postnatal therapy, but this procedure raises a variety of safety and ethical issues that need to be addressed in the context of the current standards of care which, in many cases, comprise mainly palliative support because of a dearth of real therapeutic options.
The key requirements for intrauterine gene therapy
Intrauterine gene therapy demands a minimally invasive procedure to treat the fetus by a route suited to target the affected organ systems with little or no toxicity. Systemic vector delivery is preferable for disorders affecting multiple organs, as is often the cause for many congenital enzyme deficiency syndromes. In the clinical setting, this may involve ultrasound-guided administration into the umbilical vein using protocols that already exist. Specificity of vectors for target organs can be improved by exchanging capsid or envelope proteins for naturally occurring variants or engineered ones that sometimes contain tissue-targeting ligands. Expression of the transgene in specific cells can additionally be achieved by the use of tissue-specific promoters or post-transcriptional mechanism such as micro-RNA. To further limit unwanted bystander effects, IUGT can also be accomplished with direct regional or local delivery under ultrasound guidance, a useful strategy where the main pathology is restricted to a particular system, such as the CNS or the retina ; however, this may pose significant challenges in the clinical setting because of excessive manipulation of the fetus, and the potentially risk of physical damage. Protocols for delivery of therapeutic vectors to organs such as the lungs in utero already exist.
The key requirements for intrauterine gene therapy
Intrauterine gene therapy demands a minimally invasive procedure to treat the fetus by a route suited to target the affected organ systems with little or no toxicity. Systemic vector delivery is preferable for disorders affecting multiple organs, as is often the cause for many congenital enzyme deficiency syndromes. In the clinical setting, this may involve ultrasound-guided administration into the umbilical vein using protocols that already exist. Specificity of vectors for target organs can be improved by exchanging capsid or envelope proteins for naturally occurring variants or engineered ones that sometimes contain tissue-targeting ligands. Expression of the transgene in specific cells can additionally be achieved by the use of tissue-specific promoters or post-transcriptional mechanism such as micro-RNA. To further limit unwanted bystander effects, IUGT can also be accomplished with direct regional or local delivery under ultrasound guidance, a useful strategy where the main pathology is restricted to a particular system, such as the CNS or the retina ; however, this may pose significant challenges in the clinical setting because of excessive manipulation of the fetus, and the potentially risk of physical damage. Protocols for delivery of therapeutic vectors to organs such as the lungs in utero already exist.
Vectors currently available for intrauterine gene therapy
Vectors are designed to deliver the transgene to the target cell and to facilitate rapid and sustained expression. An important attribute of a potential clinical vector is the ability to limit transgene expression to either the organ responsible for producing the deficient protein (e.g. liver-directed therapy for coagulation factor deficiencies) or the system that is most affected by the disease (e.g. the CNS in mucopolysaccharidoses, Gaucher and Tay-Sachs disease), in order to minimise possible adverse effects from ectopic transgene expression. This characteristic is a function of the vector capsid or envelope proteins and influenced by the choice of promoter-driving transgene expression. A particularly important development in vector engineering is the manipulation of capsid proteins to enhance interaction with target cell-surface receptors.
Vectors available for clinical trials are integrating (e.g. gammaretroviruses and lentiviruses) or non-integrating (e.g. adenovirus and adeno-associated viral vectors). Gammaretroviruses are limited by their ability to transduce only dividing cells, as they require nuclear membrane disruption before their integration into host DNA. Lentiviruses, in contrast, are actively transported into the nucleus, giving them an ability to transduce post-mitotic cells. The ability to integrate within the host genome of stem cells such as haematopoietic stem cells (HSC) makes gammaretro- and lentiviral vectors important for IUGT strategies focused on the treatment of thalassaemias.
Adenoviruses have a large capacity for transgenes, and can transduce a variety of mitotic and post-mitotic cells with high efficiency. Older-generation adenoviral vectors evoked strong inflammatory response, limiting their utility of IUGT. This is, however, less of a problem with the new generation of helper-dependent vectors that are devoid of wild-type adenoviral genes. But, as these vectors do not integrate into host tissues, their utility for the intrauterine treatment of monogenic disorders is less clear.
Adeno-associated viral vectors (AAV) can also transduce dividing and non-dividing cells. In the clinic, their success had been limited to the transduction of post-mitotic tissues, such as the retina or the liver, which support stable long-term expression of therapeutic transgenes largely from episomally (non-integrated) maintained transgenes. As sizeable percentages of the human population are sensitised to various AAV serotypes via childhood or adolescent exposure, the use of alternate serotypes from non-human species is of interest in strategies designed to evade pre-existing host immunity and restrict tropism to the target organ. Modification of the AAV genome into a self-complementary format has resulted in improved gene-transfer efficiency. Another important limitation of recombinant AAV vectors is their small packaging capacity of less than 5.0 kb, which severely restricts the utility of this vector system, although several groups have investigated ways of delivering large genes using this vector system.
Non-viral gene transfer is carried out by transfecting cells with donor DNA using electroporation or other physical or chemical means of facilitating gene transfer, which in general is an inefficient process that results in a short duration of transgene expression. The use of zinc finger nucleases enable site-specific integration of expression cassettes delivered by non-viral methods, ensuring stable long-term expression of the transgene. Non-viral gene transfer methods have a great safety record and have been evaluated in the context of IUGT but, as transgene expression was uniformly short-lived, this method will not be discussed further in this chapter.
Candidate diseases for treatment by intrauterine gene therapy and rationale for fetal intervention
Recent gene-therapy trials with a range of vector systems show significant successes for haemophilia B, retinal blindness and severe combined immunodeficiency, signalling an new era in this field that has, until recently, failed to deliver on its promise. Candidate diseases suitable for IUGT are initially likely to be well-characterised monogenic defects that cause significant perinatal or early childhood morbidity. Others include diseases in which a precise molecular diagnosis can be made prenatally, and those for which an effective postnatal treatment is unavailable.
A large number of disorders are potential candidates for IUGT. One example includes the haemoglobinopathies, which carry a global carrier frequency of 7%, particularly alpha-thalassaemia major (Bart’s hydrops), which is fatal without treatment. Other examples include rare perinatally lethal clotting disorders such as Factors VII and X deficiencies, lysosomal storage disorders (e.g. the mucopolysaccharidoses, Gaucher, Niemann-Pick and Tay-Sachs diseases) that result in significant perinatal morbidity and mortality, and hereditary blindness, such as Leber congenital amaurosis.
Certain diseases are prevalent in specific ethnic populations, such as cystic fibrosis, which has a carrier rate of 4% among Northern European or Ashkenazi Jewish populations, with an incidence of 1:2000 to 1:3500 births in Europe and the USA. In contrast, the beta-haemoglobinopathies, the most common single-gene disorders in humans, account for a carrier rate of 5.2% globally, whereas the incidence of conceptions affected by Thalassaemia major is estimated to be 4.6 per 10,000 pregnancies (6.6 per 10,000 pregnancies in South-East Asia). With diverse intercontinental movement of at-risk populations, however, the geographical boundaries are continuously changing, and the collective burden of untreated disease is a global one. Some of these disorders can be treated with haematopoietic stem-cell transplantation postnatally if a suitable donor is available, which, in turn, is dependent on the ethnic origin of the individual. As mentioned previously, however, for most diseases, significant irreversible organ damage has already occurred at birth or treatment options are limited. The emerging evidence collectively point to a window in early gestation in which immune and anatomical barriers to gene transfer can be circumvented to achieve effective target organ transduction while promoting tolerance to the transgene.
The evidence of efficacy for intrauterine gene therapy
Evaluation of intrauterine gene therapy in mice
Rodents have been indispensable at demonstrating the safety and efficacy of IUGT because of the availability of knock-out models in which pregnancies are of short duration and polytocous, providing an opportunity to demonstrate genetic and phenotypic correction. With these models, successful cure has been established in Haemophilia B, mucopolysaccharidosis type VII, and others ( Table 1 ). Beyond perinatal disease, IUGT has demonstrated the potential to ameliorate adult-onset disorders, such as rheumatoid arthritis. Only partial success, however, has been achieved in treating other conditions, such as Duchenne muscular dystrophy.
Author | Disease model | Genetic mutation | Animal | Vector/ cell | Transgene | Main outcomes | |
---|---|---|---|---|---|---|---|
Coagulation disorders | Sabatino et al., 2007 | Haemophilia B | Human FIX | Mouse | AAV1 and AAV2 | Human FIX | Sustained Human FIX expression in the absence of an immune response. |
Waddington et al., 2004 | Mouse | Lentivirus | Human FIX | Permanent correction of phenotype in the absence of an immune response. | |||
Niiya et al., 2009 | Congenital thrombotic thrombocytopaenic purpura | A disintegrin and metalloprotease with thrombospondin 13 | Mouse | Lentivirus | ADAMTS13 | Sustained transgene expression and correction of prothrombotic phenotypes. | |
Enzyme-deficiency syndromes | Seppen et al., 2003 | Crigler–Najjar Type I | Uridine diphosphate- glucuronosyl transferase 1A1 | Rat | Lentivirus | Human glucuronosyltransferase 1A1 | 45% decrease in serum bilirubin; clinically relevant for converting severe Type I to benign Type II. |
Karolewski and Wolfe, 2006 | Muco–polysaccaridosis VII | GUSB | Mouse | AAV1 | GUSB | Widespread symmetrical central nervous system transduction after intracerebral injection resulting in therapeutic levels of GUSB and prevention of neurodegeneration. | |
Roybal et al., 2011 | Wilson’s disease | Human Atp7b | Mouse | Lentivirus | Human Atp7b | Decreased hepatic copper content and restoration of normal morphology. | |
Sugano et al., 2011 | Hypophosphatasia | ALPL | Mouse | AAV9 | ALPL | Normal growth and seizure-free survival for 8 weeks (normal lifespan 2 weeks). | |
Hereditary blindness | Dejneka et al., 2004 | Leber congenital amaurosis | Retinal Pigment Epithelium 65 (RPE 65) | Mouse | AAV2/1 | Human RPE65 | Efficient transduction of pigmented retinal epithelium with correction of abnormal phenotype. |
Muscle and skin disorders | Reay et al., 2008 | Duchenne muscular dystrophy | Dystrophin | Mouse | Adenovirus | Dystrophin | Partial correction with improved muscle force and reduction in muscle mass; subtherapeutic level of muscle Transduction. |
Endo et al., 2011 | Junctional epidermolysis bullosa | Laminin-β3 | Mouse | Lentivirus | Laminin, beta 3 | Long-term correction of skin pathology in lethal form of junctional epidermolysis bullosa. |
The limitations of the murine model include delayed immunological development, morphologically and functionally distinct murine labyrinthine placenta, short murine gestation of 21 days, and the limited ability to establish stability of transgene expression and delayed-onset toxicity.
Delayed immunological development
The murine immune system completes its maturation in the postnatal period. Murine T and B lymphocytes first appear at 0.85 G, functional lymphocyte and natural killer cell activity is detected 3 weeks after birth, and the repertoire of expressed Ig isotypes and lymphocyte regulation is distinct. This contrasts with humans in which mitogen-responsive lymphocytes are detectable in peripheral blood by 0.3–0.4 G, and where functional natural killer cells develop at 0.7 G.
Morphologically and functionally distinct murine labyrinthine placenta
Morphologically and functionally distinct murine labyrinthine placenta is different enough from the human haemochorial placenta to restrict the ability to translate data about transplacental trafficking and maternal safety to humans.
The short murine gestation
The short murine gestation of 21 days limits assessment of gestation-dependent outcomes, and it is difficult to determine the safety and efficacy of systemic vector delivery in the equivalent of the first trimester.
Limited ability to establish stability of transgene expression and delayed-onset toxicity
The limited ability to establish stability of transgene expression and delayed-onset toxicity is caused by the short murine lifespan, and is best illustrated by the example of retroviral-mediated insertional mutagenesis leading to T-cell leukaemia in five out of 20 participants, diagnosed 2.5 to 5 years after ex-vivo gene therapy for X-linked severe combined immunodeficiency.
Assessment of intrauterine gene therapy in sheep
The fetal sheep model allowed a better understanding of the interaction between IUGT and the immune system. From ovine experiments it became clear that successful IUGT depends on route of vector administration, fetal immune maturity at the time of injection and the loading vector dose. Ovine immune ontogeny is well defined, with a pre-immune period before 0.3–0.45 G, when lymphocytes develop in the thymus and peripherally. It is, therefore, not surprising that IUGT at 0.60.8 G was associated a brisk humoral response to the vector and transgenic protein, whereas systemic IUGT at 0.2–0.4 G showed comparatively little cellular or humoral immunity despite wide distribution of vector, resulting in durable transgene expression for a period of at least 5 years. Pre-immune recipients also demonstrated central tolerance by not rejecting postnatally administered transgenic protein. The gestational age at the time of vector administration also influenced vector biodistribution. More extensive hepatic transgene expression was observed in fetuses injected intraperitoneally at 0.3–0.7 G compared with predominantly pulmonary expression seen after this window. This observation is likely to be associated with temporal changes in cell proliferation, appearance of viral-specific cell-surface receptors, or both.
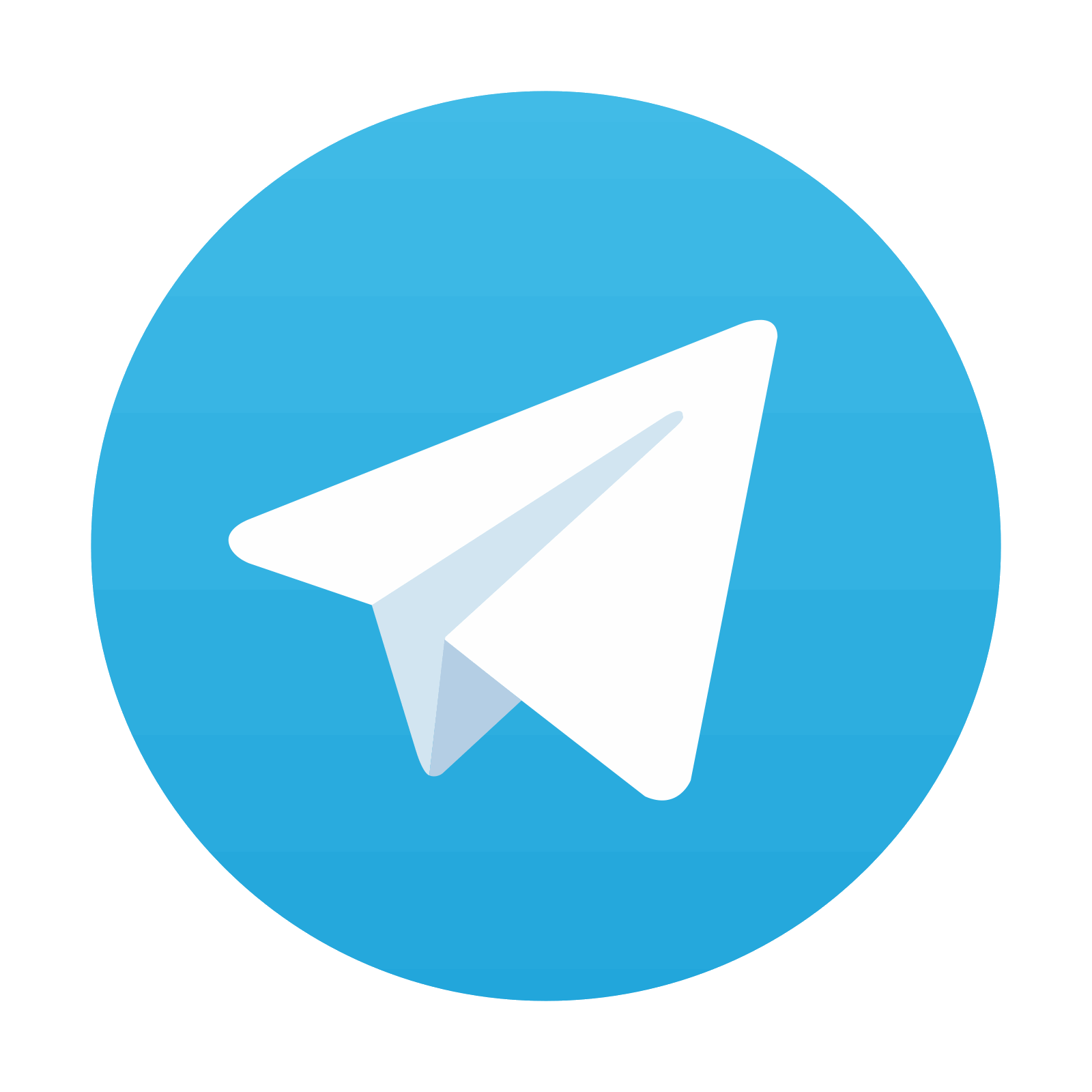
Stay updated, free articles. Join our Telegram channel
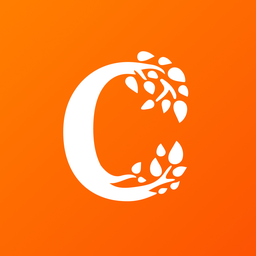
Full access? Get Clinical Tree
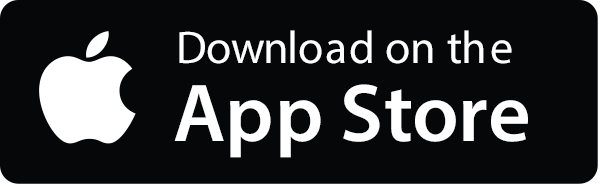
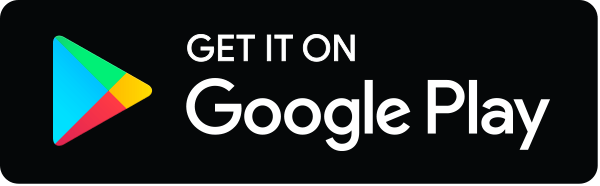