Background
Although synthetic mesh is associated with superior anatomic outcomes for the repair of pelvic organ prolapse, the benefits of mesh have been questioned because of the relatively high complication rates. To date, the mechanisms that result in such complications are poorly understood, yet the textile characteristics of mesh products are believed to play an important role. Interestingly, the pore diameter of synthetic mesh has been shown to impact the host response after hernia repair greatly, and such findings have served as design criteria for prolapse meshes, with larger pores viewed as more favorable. Although pore size and porosity are well-characterized before implantation, the changes in these textile properties after implantation are unclear; the application of mechanical forces has the potential to greatly alter pore geometries in vivo. Understanding the impact of mechanical loading on the textile properties of mesh is essential for the development of more effective devices for prolapse repair.
Objective
The objective of this study was to determine the effect of tensile loading and pore orientation on mesh porosity and pore dimensions.
Study Design
In this study, the porosity and pore diameter of 4 currently available prolapse meshes were examined in response to uniaxial tensile loads of 0.1, 5, and 10 N while mimicking clinical loading conditions. The textile properties were compared with those observed for the unloaded mesh. Meshes included Gynemesh PS (Ethicon, Somerville, NJ), UltraPro (Artisyn; Ethicon), Restorelle (Coloplast, Minneapolis, MN), and Alyte Y-mesh (Bard, Covington, GA). In addition to the various pore geometries, 3 orientations of Restorelle (0-, 5-, 45-degree offset) and 2 orientations of UltraPro (0-, 90-degree offset) were examined.
Results
In response to uniaxial loading, both porosity and pore diameter dramatically decreased for most mesh products. The application of 5 N led to reductions in porosity for nearly all groups, with values decreasing by as much as 87% ( P < .05). On loading to 10 N of force, nearly all mesh products that were tested were found to have porosities that approached 0% and 0 pores with diameters >1 mm.
Conclusion
In this study, it was shown that the pore size of current prolapse meshes dramatically decreases in response to mechanical loading. These findings suggest that prolapse meshes, which are more likely to experience tensile forces in vivo relative to hernia repair meshes, have pores that are unfavorable for tissue integration after surgical tensioning and/or loading in urogynecologic surgeries. Such decreases in pore geometry support the hypothesis that regional increases in the concentration of mesh leads to an enhanced local foreign body response. Although pore deformation in transvaginal meshes requires further characterization, the findings presented here provide a mechanical understanding that can be used to recognize potential areas of concern for complex mesh geometries. Understanding mesh mechanics in response to surgical and in vivo loading conditions may provide improved design criteria for mesh and a refinement of surgical techniques, ultimately leading to better patient outcomes.
Synthetic mesh use in the surgical repair of pelvic organ prolapse is widespread, with approximately one-third of all surgical repairs using mesh. Ideally, synthetic mesh provides structural support to the vagina to eliminate the symptoms of prolapse, restore vaginal function, and relieve the psychosocial issues that result from this disorder. Although synthetic mesh–augmented prolapse repairs boast superior anatomic outcomes relative to repairs that use native tissues, the benefit of mesh has been questioned because of complication rates that are as high as 20%, with notable rates of pain and mesh exposure ( Figure 1 , a).
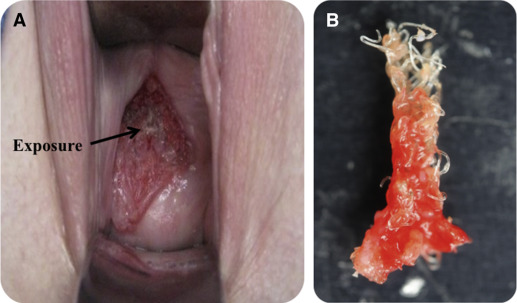
In an attempt to define the mechanism of complications, significant focus has been placed on the textile properties of mesh. Specifically, the geometry and dimensions of the mesh pores have been found to impact the biologic response to mesh directly. Indeed, greater pore sizes were found to yield mesh-tissue composites of greater strength and increased collagen deposition; smaller pores restricted vascular growth and contained less mature collagen. Notably, it has been shown that effective tissue in-growth, which is characterized by the quality of the tissue that forms between mesh fibers, occurs in mesh pores with a diameter of ≥1 mm for polypropylene mesh. Importantly, pore diameters of <1 mm are associated with an enhanced inflammatory response that accompany poor tissue in-growth and fibrotic encapsulation. Thus, it is not surprising that nearly all contemporary vaginal mesh products are constructed with initial pore diameters of >1 mm. Yet, despite this design feature, it is not uncommon for mesh to appear bunched after implantation, particularly in areas of complications ( Figure 1 , b).
The apparent deformation of prolapse meshes highlights the need to consider the mechanical environment in which mesh is placed. Specifically, both abdominal sacrocolpopexy and transvaginal procedures anchor mesh (or mesh arms) at 2 distinct locations (vagina and sacrum or vagina and pelvic sidewall, respectively). Thus, when surgically tensioned to remove the presence of a vaginal bulge or loaded by other pelvic organs and/or abdominal pressure, the mesh largely experiences unidirectional (uniaxial) tensile loads along significant regions of the device ( Figure 2 ). In response to this loading condition, one would anticipate pore geometries to deform readily as the load is increased. Such loading previously has been shown to reduce mesh porosity. Therefore, surgical tensioning and/or in vivo mechanical loading may provide a potential mechanism to explain clinical observations, often described as “mesh shrinkage.”
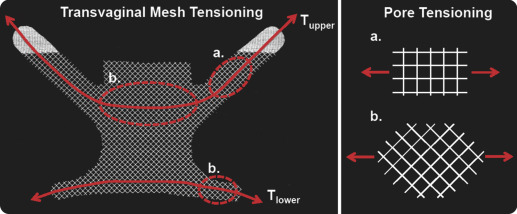
Although previous studies have performed mechanical testing of synthetic mesh, many of these report data that are related to mesh failure (ie, begins tearing apart). However, mechanical failure of synthetic mesh products is extremely rare clinically, because the typical failure properties of mesh far exceed in vivo loads and deformations. Rather, this study aims to characterize pore deformation at levels of force that occur in vivo and during surgical implantation, while considering the impact of initial pore orientation. We hypothesize that, regardless of initial pore geometry, mesh pores will become unsuitable for effective tissue ingrowth (dimensions, <1 mm) with decreased porosity on tensile loading.
Materials and Methods
Four synthetic mesh products with distinct pore geometries were considered: Gynemesh PS (Ethicon, Somerville, NJ), UltraPro (aka Artisyn; Ethicon), Restorelle (Coloplast, Minneapolis, MN), and Alyte Y-mesh (Bard, Covington, GA; Table 1 ). Each product was cut to 90×15 mm strips along their recommended implantation direction. Multiple orientations were considered for several mesh products based on pore geometry and anticipated loading conditions for current sacrocolpopexy and transvaginal meshes. Specifically, UltraPro was loaded with mesh cut at 0- and 90-degree offset from the recommended direction (labeled herein as UltraPro and UltraPro Opp , respectively). Because the initial pore geometry of Restorelle is square, porosity was not expected to change significantly in response to loading along this implantation direction. However, loading the mesh along an axis 45-degree offset to the square configuration (ie, tensioning a diamond shape pore) was expected to result in significant deformation. Therefore, Restorelle samples were cut in 3 orientations: pores offset at 0-, 5-, and 45-degrees from the horizontal axis. The 0- and 45-degree orientations were chosen based on geometry but were further justified by the anticipated loading of current transvaginal product designs (DirectFix; Figure 2 ), for which loading along these angles does occur. The 5-degree offset is clinically relevant, because small changes in orientation are likely to occur during implantation or cutting of the mesh.
Mesh | Vendor | Pore size, mm | Porosity, % |
---|---|---|---|
Gynemesh | Ethicon | 2.5 | 62 |
Restorelle | Coloplast | 1.8 | 78 |
Alyte Vaginal | Bard | 2.8 | 75 |
Alyte Stem | Bard | 2.0 | 50 |
UltraPro a | Ethicon | 3.8 | 68 |
a UltraPro (aka Artisyn) measurements made after absorbable component is absorbed.
Additionally, the intact Alyte mesh consists of 2 distinct sections; a section for vaginal attachment and a section intended for sacral attachment ( Table 1 ). Although the general architecture is similar between the 2 locations, the sacral section consists of 2 offset layers of the vaginal section, which are knitted together. This construction effectively doubles the amount of material in the sacral section. Thus, samples from the vaginal and sacral sections were considered independently and demonstrate the impact of increased material on the deformation of mesh pores. Five samples that represented each of these aforementioned groups were independently tested.
The uniaxial testing apparatus and methods used have been described previously All samples were secured in custom soft-tissue clamps on a materials testing machine (Instron 5565, Grove City, PA) such that the minimum clamp-to-clamp distance was 75 mm, which provided a minimum aspect ratio of 5. To remove slack, samples were preloaded to 0.1N at a rate of 10 mm/min. After the preload was applied, each mesh was loaded to 5N at 50 mm/min and subsequently to 10N at 50 mm/min. Neither force nor elongation measurements were zeroed between loading steps. Based on reported intraabdominal pressure and our measurements of surface area for the anterior vagina, the loads used in this protocol were within the estimated range of in vivo loads (eg, valsalva, coughing) placed on vaginal tissue. We estimated <5N of force at resting intraabdominal pressure, although load values vary with vaginal dimensions, patient-specific abdominal pressures, or level of activity (ie, resting, jumping, coughing).
After the application of each load (preload included) the mesh mid region was imaged with the use of a digital SLR camera (EOS Rebel T3; Canon, Melville, NY) that was equipped with a 60-mm macro lens (EFS f/2.8; Canon). To produce repeatable image quality, all images were taken with high aperture (F16) and low ISO settings (ISO 100). In addition, all samples were imaged before testing, which provided 4 loading states for each mesh (0, 0.1, 5, and 10N). From each image, pore geometries were analyzed with a 10×10 mm section of the mesh midregion. The dimensions used for image analysis captured the repeating unit structure (pore geometry) of each mesh used in this study, but they were small enough to minimize the influence of the fixed boundaries imposed by the testing clamps, because larger porosity values would be observed closer to the clamps. Images were scaled and cropped using ImageJ (National Institutes of Health, Bethesda, MD) and then imported into a custom Mathematica script (V9, Champaign, IL). Images were then binarized with a thresholding procedure to ensure that all mesh fibers were included in analysis ( Figure 3 ).
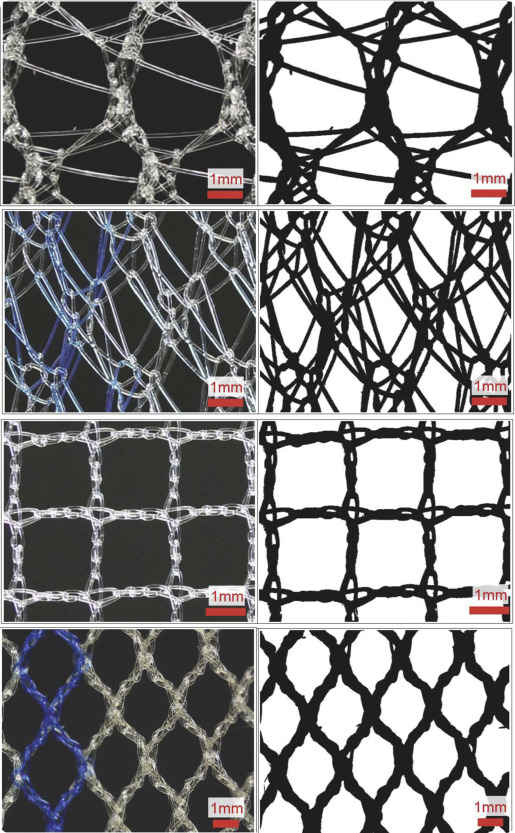
After thresholding, mesh porosity and the pore diameters were determined. To measure porosity, each image pixel was determined to be either mesh ( Figure 3 , black pixels) or void space ( Figure 3 , white pixels), which allowed for porosity to be calculated as:
Porosity = void pixels/total pixels
Porosity is a relative measure of the amount of mesh material per unit area, with a value of 0 representing a solid piece of mesh (no pores) and a value of 1 representing no mesh. To determine pore diameter, an algorithm was used to identify isolated clusters of white pixels (pores) and to calculate the centroid for each cluster. For each pore, the shortest distance, or minimum diameter (d min ), was recorded, creating a continuous distribution of d min for all pores in an image.
To display trends in pore diameter more easily, histograms were created such that d min values were grouped into 4 classes from 0-2 mm with a bin range of 0.5 mm. The contribution of each d min class to the total pore area was reported as the area fraction, where area fraction was defined as the pixel area of pores from a given diameter class divided by the total pore area in an image. Because of the lateral contraction of the mesh samples in response to tensile loading, void space outside of the mesh boundaries was not included for porosity measurements. Further, to avoid skewing of d min measurements, pores that were identified along the image perimeter were excluded from analysis.
Structural properties were computed for each mesh based on the load-elongation data that were obtained during testing. Here, elongation refers to the mesh elongation that resulted from the applied load. Stiffness measurements were made considering the nonlinearity of these curves as previously described. The stiffness measurement, defined as the low stiffness , was calculated by taking the minimum slope of the load-elongation curve with a moving window of 5% elongation; high stiffness was calculated as the maximum slope of the curve with a 5% window. During imaging at 5N, each mesh experienced stress relaxation with the observed load decreasing to approximately 3N. This stress relaxation, which likely results from small changes in the knot structure, produced large initial stiffness values for 10N trials. Given the elevated initial stiffness for 10N trials, only structural properties from 0–5N were reported.
For statistical analysis, a repeated measures analysis of variance with Bonferroni post-hoc was used to examine the effect of loading on mesh porosity. The impact of loading on d min was examined using a Kruskal-Wallis test. Finally, the structural properties were compared between groups with a 1-way analysis of variance with Bonferroni or Dunnett’s T3 post-hoc, as appropriate. Statistics were performed with SPSS software (V20; IBM Corporation, Armonk, NY), with a significance set at a probability value of ≤.05.
Results
Before being mounted in our testing system (0N), all meshes had pore sizes significantly >1 mm and porosities >50% ( Table 1 ); however, uniaxial loading dramatically altered the overall appearance of mesh with notable changes in pore geometry ( Figure 4 ). At 0N Gynemesh and Alyte’s sacral portion had the lowest porosities (60.1% and 49.7% respectively; Figure 5 ). Mounting these samples and applying a 0.1N preload resulted in small decreases in porosity for several groups that were not significant ( Figure 5 ). On the other hand, the application of 5N led to highly significant changes in pore shape and porosity for nearly all groups, with values decreasing by as much as 87% of their original porosity. Restorelle 0-degree offset (square pore) was the only mesh whose porosity was not significantly reduced on application of load ( P > .05; Figure 5 ). At 5N, Restorelle 45-degree offset, UltraPro Opp , and Alyte vaginal section saw the largest reductions in porosity, decreasing to just 9.5%, 11.7%, and 14.5% porosity, respectively. Gynemesh and Restorelle 5-degree offset experienced less reduction, approximately an 8% decrease in porosity ( P < .05). At 10N of force, all mesh groups other than Restorelle 0-degree and Restorelle 5-degree offset experienced such large pore reductions that the mesh structure grossly appeared as a solid piece of polypropylene ( Figure 4 ). The porosity values were now only 15.5%, 10.2%, 6.4%, 3.8%, and 8.6%, for Gynesmesh, UltraPro Opp , Restorelle 45-degree offset, Alyte vaginal, and Alyte sacral, respectively ( Figure 5 ).
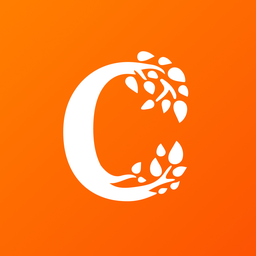
Full access? Get Clinical Tree
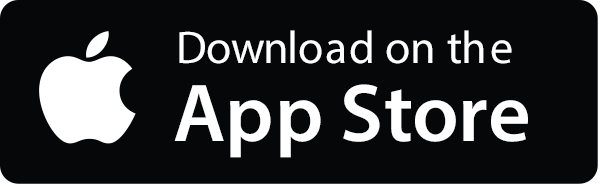
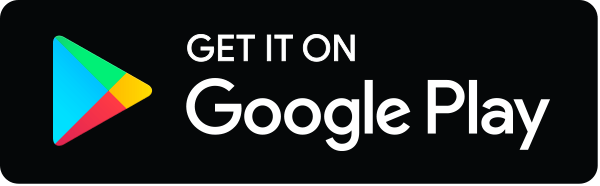