Telomeres are nucleoprotein complexes located at the distal ends of chromosomes. In adults, progressive telomere shortening occurs throughout the lifetime and is thought to contribute to progressive aging, physiological senescence, multiorgan dysfunction, and ultimately, death. As discussed in this review, multiple lines of evidence provide support for the biological plausibility that a telomere-based clock mechanism also determines the length of gestation, leading to the onset of labor (parturition). After telomere expansion at the beginning of pregnancy, the telomere lengths in the gestational tissues (ie, the placenta and fetal membranes) progressively shorten throughout the remainder of pregnancy. The rate of telomere shortening can be accelerated by conditions that affect the mother and result in oxidative stress. Preterm births in the United States are associated with multiple risk factors that are linked with increased oxidative stress. Antioxidant vitamins (ie, vitamins E and C) mitigate the effects of oxidative stress and delay or prevent telomere shortening. Clinical trials with vitamins E and C and with multivitamins started during the periconception period have been associated with reduced rates of preterm births. In the United States, African-American women have a 2–3-fold higher rate of preterm birth. African-American women have multiple risk factors for premature birth, all of which are distinct and potentially additive with regard to epigenetic telomere shortening. The “weathering effect” is the hypothesis to explain the increased rates of chronic illness, disabilities, and early death observed in African-Americans. With regard to pregnancy, accelerated weathering with the associated telomere shortening in the gestational tissues would not only explain the preterm birth disparity but could also explain why highly educated, affluent African-American women continue to have an increased rate of preterm birth. These studies suggest that the racial disparities in preterm birth are potentially mediated by telomere shortening produced by lifetime or even generational exposure to the effects of systemic racism and socioeconomic marginalization. In conclusion, this review presents multiple lines of evidence supporting a novel hypothesis regarding the biological clock mechanism that determines the length of pregnancy, and it opens the possibility of new approaches to prevent or reduce the rate of spontaneous preterm birth.
Introduction
In the United States, 9% to 14% of pregnancies end with preterm births, resulting in the delivery of over 400,000 premature infants per year; the number of preterm births worldwide is estimated to be as high as 15 million per year. , In the United States, preterm birth is one of the leading causes of infant morbidity and mortality, resulting in thousands of young lives lost and millions of dollars in medical care costs every year. Because of the magnitude of this obstetrical problem and the lack of effective pharmacologic interventions to address it, there is a need to identify and test the effectiveness of new therapeutic interventions to prevent or reduce the rate of preterm birth. On the basis of decades of research by countless researchers, much is known regarding the biochemical, endocrine, proinflammatory, anatomic, and electrophysiologic events that occur with the onset of and during spontaneous labor (parturition). However, none of this research has determined the exact nature of the biological clock that determines the length of gestation. Most preterm births occur spontaneously, resulting from the onset of cervical ripening and phasic myometrial contractions with or without the rupture of the membranes. The important difference is the premature timing for these events. A better understanding of the gestational biological clock and how the clock can be disrupted leading to premature birth will greatly facilitate the development of new, effective clinical interventions. Some of the evidence supporting the biological plausibility of a telomere-based gestational clock has been previously published by the author. , The current review will discuss this hypothesis in greater detail and provide additional evidence supporting the premise that the timing for spontaneous parturition is regulated by a telomere-based biological clock and that this clock can be disturbed by oxidative stress, leading to a premature shortening of gestational length resulting in preterm birth ( [CR] ). In addition to basic and translational science research studies, the current review provides information on the basis of multiple published clinical studies (annotated in Table 1 ) and several previously published review articles (annotated in Table 2 ).
Authors | Year | Study type | Topic |
---|---|---|---|
Hanna et al | 2009 | Observational | Short telomeres and pregnancy loss |
Thilagavathi et al | 2013 | Observational | Short telomeres and couples |
Kim | 2022 | Observational | Maternal age and PTB |
Olapeju et al | 2021 | Observational | Maternal age and PTB |
Wilcox et al | 2008 | Observational | Female line & PTB risks |
Urquia et al | 2019 | Observational | Female line and PTB risks |
Smid et al | 2017 | Observational | AA women, generations and PTB |
Page et al | 2021 | Observational | Mexican-origin women and PTB |
Wilson et al | 2016 | Observational | Short telomeres and fetal gender |
Menon et al | 2012 | Observation | Fetal telomeres and PTB |
Colatto et al | 2020 | Observational | Feral telomeres and PTB |
Lai et al | 2021 | Observational | Short telomeres, placenta and fetal membranes |
Wang et al | 2016 | Observational | Cadmium exposure and PTB |
Nishijo, et al | 2002 | Observational | Cadmium exposure and PTB |
Asefi et al | 2022 | Meta-analysis | Cadmium exposure and PTB |
Corina et al | 2019 | Randomized clinical trial | Vitamin E and telomere lengths |
Koenig et al | 2017 | Observational | Vitamin E and risk for PTB |
Zhang et al | 2017 | Case–control study | Vitamin E and PTB |
Siega-Riz et al | 2003 | Observational | Vitamin C and risk for PTB |
Myers et al | 2021 | Observational | Vitamin C and fetal telomere lengths |
Hauth et al | 2010 | Randomized clinical trial | Vitamin E and C and PTB prevention |
Abramovici et al | 2015 | Randomized clinical trial | Smoking, vitamins E and C and PTB prevention |
Bartfai et al | 2012 | Case–control study | Vitamin E and PTB prevention |
Vahratian et al | 2004 | Observational | Periconception vitamins and PTB prevention |
Catov et al | 2011 | Case–control study | Periconception vitamins and PTB prevention |
Johnston et al | 2016 | Observational | Periconception vitamins and PTB prevention |
Harville et al | 2020 | Observational | Serum antioxidants and PTB risk |
Shahin et al | 2009 | Randomized clinical trial | NAC treatment and PTB prevention |
Nasr | 2010 | Randomized clinical trial | NAC Treatment & Pregnancy Outcome |
Amin et al | 2008 | Prospective clinical trial | NAC treatment and pregnancy outcome |
Saroyo et al | 2021 | Observational | Short telomeres and term and preterm labor |
Lo et al | 1998 | Observational | Cell-free fetal DNA thru gestation |
Ariga et al | 2001 | Observational | Cell-free fetal DNA thru Gestation |
O’Campo et al | 2008 | Observational | AA women, neighborhoods and PTB |
Marchetto et al | 2016 | Observational | Prenatal stress and newborn telomeres |
Entringer et al | 2013 | Observational | Maternal psychosocial stress and newborn telomeres |
Salihu et al | 2016 | Observational | Maternal perceived stress and fetal telomeres |
Bijnens et al | 2015 | Observational | Maternal residence and placental telomeres |
Martens et al | 2017 | Observational | Prenatal pollution exposure and newborn aging |
Aliyu et al | 2010 | Observational | Intrauterine tobacco exposure and PTB |
Salihu et al | 2015 | Case–control Study | Intrauterine tobacco exposure and fetal telomeres |
Smeets et al | 2015 | Observational | Telomeres after term and preterm birth |
Papiernik et al | 1990 | Observational | Racial differences in pregnancy duration |
Jones et al | 2017 | Observational | Placental telomeres and PTB racial disparity |
Peelen et al | 2016 | Nationwide observational cohort study | Fetal gender, telomeres and risk of PTB |
Vatten and Skjaerven | 2004 | Population-based observational study | Newborn gender, telomeres and gestation |
Giurgescu et al | 2012 | Observational | PTB and AA women, environment, discrimination, and stress |
Srinivasjois et al | 2012 | Meta-analysis | Mixed-race couples and risk of PTB |
Simons et al | 2018 | Observational | Weathering and Black Americans |
Simons et al | 2021 | Observational | Weathering and aging: social adversity, discrimination, and health |
Chae et al | 2020 | Observational | Racial discrimination and telomere shortening |
Geronimus et al | 2015 | Observational | Race-ethnicity, urban stressors, and telomeres |
Ruiz et al | 2017 | Observational | Acculturation and shortened telomeres |
Collins et al | 2011 | Observational | AA women, economic mobility and PTB |
Collins et al | 2015 | Observational | White women, economic mobility and PTB |
Johnson et al | 2020 | Observational | AA women, high socioeconomic status and PTB |
Authors | Year | Topic |
---|---|---|
Phillippe and Phillippe | 2017 | Telomere gestational clock and aging |
Phillippe | 2015 | Cell-free DNA signaling parturition |
Lu et al | 2013 | Telomere structure and function |
Lenart el al | 2016 | DNA and aging |
Gomes et al | 2011 | Telomeres and longevity |
Roake et al | 2017 | Cellular aging, telomeres, and p53 |
Menon | 2014 | Oxidative stress, DNA damage, and telomeres |
Singh et al | 2018 | Cadmium, oxidative stress, and PTB |
Epel et al | 2004 | Telomere shortening and life stress |
Burton and Jauniaux | 2011 | Pregnancy, placenta and oxidative stress |
Romero et al | 2006 | Inflammation, preterm, and term labor |
Christiaens et al | 2008 | Inflammation, endocrine effect, and parturition |
Strauss et al | 2018 | Genetics and spontaneous PTB |
Williams | 1999 | Race, socioeconomic status, and health |
Culhane and Goldenberg | 2011 | Racial disparities in PTB and nativity effect |
Telomeres and pregnancy
Telomeres are nucleoprotein complexes located at the distal ends of chromosomes. They contain long, tandem, repeat DNA sequences and are crucial with regard to chromosomal stability ( Figure 1 ). , , In mammals, the double-stranded telomeres consist of hundreds of copies of the nucleotides TTAGGG and its complementary DNA sequence, followed by terminal guanine-rich, single-stranded overhangs on the 3’ strands. , Thus, telomeres span thousands of bases in length as observed in human cells, with the telomere lengths ranging from approximately 5000 to 15,000 bases (or 5–15 kilobases [kb]). , , The telomere lengths vary significantly between chromosomes, between organs in the body, and between different animal species. , Because of the biochemical inability to completely replicate one of the DNA strands during DNA replication (ie, the lagging strand), telomere sequences progressively shorten with each cell replication and division. Human somatic cells lose 50– 200 base pairs (bps) of telomere sequence with each replication. , This DNA attrition rate is not constant and can be accelerated by environmental factors such as oxidative stress, inflammation, and high rates of cell turnover. , , Progressive telomere shortening has been proposed to function as a biological clock, signaling cellular senescence and resulting in cell cycle arrest, chromosome breaks, abnormal fusion events, and/or apoptosis. , , In adults, progressive telomere shortening occurs throughout the lifetime and is thought to contribute to progressive aging, physiological senescence, multiorgan dysfunction, and ultimately, death ( Figure 2 ). Telomerase, which is a ribonucleoprotein complex enzyme that produces the de novo addition of TTAGGG sequences and the 3’ guanine-rich single-stranded overhangs, counteracts telomere shortening. However, this novel enzyme is only expressed at functionally significant levels in highly replicative cell types including germ cells, stem cells, and cancer cells. , ,


Telomere lengths seem to be a highly heritable trait, with transmission occurring across generations through both genetic and epigenetic mechanisms. The telomere lengths in mature oocytes are determined predominantly by the maternal telomere lengths, and they have been observed to be shorter than those found in maternal somatic cells. This is thought to be in part because of the oxidative stress causing telomere loss that occurs during the long periods between the initiation and completion of meiosis (ie, decades in the case of human females) and in part because of the low levels of telomerase activity found in oocytes. Women with shorter-than-average telomeres will pass these short telomeres onto their oocytes and ultimately their developing embryos. Supporting the premise that short maternal telomeres are passed to the conceptus and affect pregnancy outcomes, Hanna et al reported that women with unexplained recurrent pregnancy losses have shorter age-adjusted mean telomere lengths than controls. The fertilized embryos receive telomere contributions from both parents. Thus, pregnancies resulting from short telomeres in both parents have also been associated with adverse outcomes as reported by Thilagavathi et al. As noted previously, telomere lengths shorten progressively with adult aging. Therefore, studies demonstrating an increase in preterm births associated with advancing maternal age provide additional support for the potential role of telomeres in determining the gestational length. A 2022 publication by Kim described the results of a US Centers for Disease Control and Prevention-sponsored Pregnancy Risk Assessment Monitoring (PRAM) system analysis for women in New York City and Washington State, in which the effects of maternal age were assessed. Kim observed that the rate of preterm births (<37 weeks) for the 6344 women in this analysis increased significantly with maternal age from 18 to 35+ years for non-Hispanic White, non-Hispanic Black and Hispanic women; an effect that was especially pronounced for those women who were not married. In a 2021 publication based on a cross-sectional analysis of 8509 women enrolled in the Boston Birth Cohort study, Olapeju et al observed that after adjusting for sociodemographic factors, biomedical conditions, and behavioral factors, the rate of spontaneous preterm birth (<37 weeks) increased significantly with maternal age from less than 20 to 40+ years old. Interestingly, Olapeju et al also observed that consistent multivitamin supplement intake, higher maternal education, and being married (ie, factors that potentially reduce oxidative stress) lowered the rate of preterm birth in the women in this study.
The passage of short maternal telomeres could also explain why higher rates of preterm birth are observed in some families and racial groups, especially along female lines. Confirming the importance of the mother in the generational recurrence of preterm birth, Wilcox et al published a report containing data on over 300,000 singleton births recorded in the Medical Birth Registry of Norway for 1967 through 2004. These investigators observed that the relative risk for mothers born preterm (35–37 weeks) of giving birth to a preterm infant was significantly increased at 1.54, and if the mother was born early preterm (22–34 weeks), the relative risk increased to 1.84. In contrast, the relative risks of a preterm birth in their offspring for fathers who were born preterm and early preterm were not significant at 1.12 and 1.06, respectively. In a 2019 publication, Urquia et al utilized the maternal and offspring birth record data for over 19,000 pregnancies in the Manitoba Population Research Data Repository for 1980–2016. These investigators confirmed the increased relative risk for a subsequent spontaneous preterm birth for women who had been born preterm themselves, ie, for women born moderately preterm (32–36 weeks), the adjusted relative risk for delivering a preterm infant was 1.35, and if born very preterm (24–31 weeks), the adjusted relative risk increased to 1.76. Urquia et al also observed that in sisters discordant in the timing for their deliveries (ie, one born preterm and the other born at term), both had the same increased risk for subsequently delivering a preterm infant, thereby suggesting a multigenerational effect going back as far as the grandmother. Support for female generational transmission of preterm birth in African-American women has been provided by Smid et al ; these investigators observed that African-American women born preterm have an increased risk of preterm birth for their offspring that passes from one generation to the next. Supporting an association between maternal short telomeres and preterm birth, a report in 2021 by Page et al described a significant association between shorter-that-normal maternal telomeres and preterm birth in a cohort of 100 self-identified Mexican-origin women.
During the first several days after fertilization, the telomeres in the early embryo expand to their genetic and environmentally determined maximal lengths. Using residual embryos from women undergoing in-vitro fertilization, Turner et al reported that after some initial variation, the telomere lengths expand at the blastocyst stage (ie, about day 5 after fertilization). Using early mouse embryos, Liu et al made similar observations of telomere lengths peaking at the blastocyst stage. Telomere expansion in the early embryo is mediated by 2 mechanisms as follows: increased telomerase activity and telomere sister-chromatid exchange (a phenomenon comparable to alternative lengthening of telomeres [ALT] utilized by cancer cells to maintain and expand their telomere sequences ). ,
After telomere expansion at the beginning of pregnancy, the telomere lengths in the gestational tissues (ie, the placenta and fetal membranes) have been reported to progressively shorten throughout the remainder of pregnancy ( Figure 3 ). In contrast to the progressive telomere shortening observed in adults that occurs over 80 years or more in the case of humans, the telomeres shorten much faster in the gestational tissues, ie, over 260–270 days (approximately 38 weeks) in human pregnancies. Previously published reports have confirmed the progressive telomere shortening (ie, increases in short telomeres with a concomitant decrease in longer telomeres) throughout pregnancy in mice and humans. , , A 2021 publication by Lai et al described a significant increase in short telomeres along with an increase in telomere-dysfunction-induced DNA damage foci in term placentas and chorioamniotic membranes compared with these tissues from elective abortions obtained at 18 weeks of gestation. In addition to short telomeres in full-term pregnancies, these investigators observed short telomeres from spontaneous preterm births, especially those complicated by intraamniotic infections. In a 2012 publication, Menon et al reported shortened telomeres in the fetal blood leukocytes of women delivering after spontaneous term births and in those with preterm births complicated by premature rupture of the membranes.
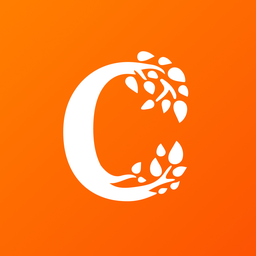
Full access? Get Clinical Tree
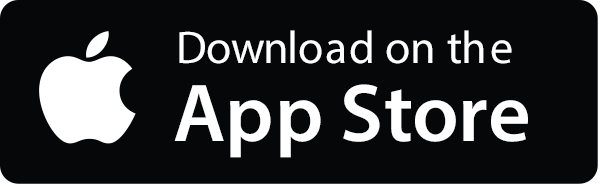
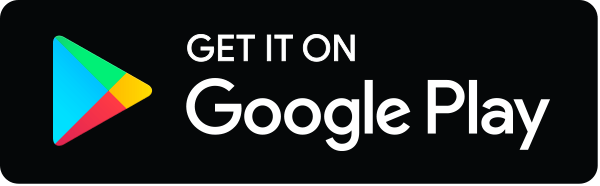