Introduction
The female pelvis is comprised of a complex, interconnected network of connective tissues and muscles that collectively work together to provide support to the pelvic organs. The vagina also provides support to the pelvic organs by supporting the urethra and bladder anteriorly, the uterus apically, and the rectum posteriorly. In the event that support to the pelvic organs is lost, via damage to or abnormalities in the connective tissues, muscles, and/or vagina, the pelvic organs unnaturally descend from their normal anatomic position into the vaginal canal, resulting in pelvic organ prolapse (POP).
To surgically restore support to the pelvic organs, a native tissue repair can be performed in which a patient’s own connective tissues and muscles are used. Given that the integrity of the existing connective tissues and muscles are likely compromised in women with prolapse, it is perhaps not surprising that native tissue repairs are associated with high failure rates: 40% by 2 years and 70% by 5 years ( ; ). As a result, surgeons began to use synthetic meshes and biologic grafts to reinforce repairs and improve patient outcomes.
The methods by which synthetic meshes and biologic grafts restore support to the pelvic organs vary. Synthetic meshes are permanent—they are designed to last for the lifetime of the patient. Support is therefore dependent on the strength of the synthetic mesh and how well the mesh integrates with the host tissue. Chemically crosslinked biologic materials were intended to act similarly to permanent materials with modifications designed to prevent host degradation. Unfortunately, these products were associated with similar or increased rates of complications relative to synthetic meshes. More recently, biologic grafts have been designed to intentionally degrade over time and to facilitate the rebuilding of damaged or compromised tissues; hence, biologic grafts act as an initial reinforcement, and following degradation and then repair by host immune cells, support is provided by the newly formed tissue.
Since the introduction of the first transvaginal prolapse mesh “kit” in 2002 ( ), synthetic meshes have been widely used in the surgical repair of prolapse. The increased use of mesh was also paralleled with a surge in the number of complications reported, which led the FDA to issue two consecutive public health notifications (PHNs) in 2008 and 2011 warning of complications associated with transvaginal meshes and upgrading them from Class II to Class III devices in 2018. As Class III devices, industry was now required to perform small clinical trials before bringing new products to the market. In 2019, the FDA mandated that manufacturers of transvaginal prolapse meshes stop the sale and distribution of their products, citing a failure to demonstrate reasonable assurance of safety and effectiveness. This series of events has prompted surgeons and their patients to reconsider the use of biologic grafts in prolapse and incontinence surgeries. Fortunately, mechanistic studies to define the mechanism of mesh and biologic graft complications have increased over the past 10 years and have identified factors contributing to complications.
The overall goal of this chapter is to provide the reader with a review of basic science and clinical studies that have aided our understanding of how the properties and mechanics of synthetic meshes and biologic grafts impact the host response, and how these factors may contribute to complications.
Synthetic meshes
Overview of synthetic meshes
The use of synthetic meshes for the treatment of POP dates back to the 1970s ( Fig. 7.1 ). During that time, there were no FDA-approved meshes for the abdominal repair of prolapse (sacrocolpopexy). The meshes used in sacrocolpopexy were the same as those approved for hernia repair. In the 1990s, gynecologists began cutting hernia mesh products into the desired shape and utilizing these modified meshes for the transvaginal repair of prolapse and surgical treatment of stress urinary incontinence (SUI). Given the surgical demand for a mesh product in the urogynecology space, companies started remarketing their hernia products and developing products targeted for stress urinary incontinence (SUI) and prolapse.

In 1998, tension-free vaginal tape (TVT; Ethicon, Somerville, NJ, United States) was developed as one of the first commercially marketed “kits” to treat SUI. Unlike previous SUI products, TVT was placed under the midurethra, and the surgery could be performed under intravenous sedation instead of general anesthesia. The product was an immediate success, leading to a competitive market with multiple manufacturers introducing their own versions of the midurethral sling ( Table 7.1 ). The products were all polypropylene but differed in their weight, pore size, knit patterns, mesh thickness, etc.
Sling | Weight (g/m 2 ) | Pore Size (µm) | Mesh Thickness (mm) |
---|---|---|---|
American Medical Systems SPARC/Monarc | 110 | 1000 | 0.66 |
Bard Uretex | 81 | 1160 | 0.62 |
Boston Scientific Advantage/Obtryx | 100 | 1182 | 0.66 |
Caldera T-sling | 140 | 698 | 0.48 |
Gynecare TVT/TVT-O | 100 | 1379 | 0.63 |
Coloplast Aris (Mentor Aris) | 70 | 374 | 0.27 |
Based on the success of midurethral slings, transvaginal prolapse mesh kits based on a similar concept were introduced to the market. The first preconfigured mesh for prolapse repair, cleared by the FDA in 2002, was Prolift (Ethicon, Somerville, NJ, United States), a transvaginal mesh kit utilizing the prolapse mesh Gynemesh PS, which had been renamed from the hernia mesh Prolene Soft. These kits offered the potential of a procedure to repair prolapse that was technically easier relative to sacrocolpopexy and had a shorter operation time. As a result, the use of transvaginal meshes escalated in prolapse surgery such that, by 2005, mesh kits were being used in over 70% of mesh-augmented prolapse repairs, and by 2010 over one-third of the 300,000 prolapse surgeries performed annually used mesh (both transvaginal and sacrocolpopexy) ( ).
Companies were originally able to remarket their hernia products for POP and SUI as 510K devices because hernia products were in existence before the 1976 Medical Device Amendments Act. Using this mechanism, companies were able to avoid costly premarket testing of new devices. Unfortunately, this resulted in the use of products that were not specifically designed for the unique soft tissues and loading conditions within the female pelvis. Not surprisingly, the accelerated use of mesh was also paralleled by an increase in the number of complications, with pain and mesh exposure through the vaginal epithelium the most commonly reported symptoms ( ). As a result, the FDA released the first PHN in 2008 warning of complications associated with transvaginal meshes, and in 2011 released an updated notification stating that these complications are not rare, occurring in approximately 20% of cases ( ). Following this PHN, public and clinical views on the use of mesh in prolapse surgeries shifted. Specifically, the use of synthetic meshes in the transvaginal repair of prolapse declined, whereas there was no change reported for the use of synthetic mesh in transabdominal or suburethral sling procedures ( ). reported a three-fold increase in mesh sling revision surgeries at tertiary academic medical centers in the United States from 2007 to 2013. The continued rise of complications resulted in the FDA reclassifying transvaginal meshes for POP from Class II to Class III devices in 2016, and ultimately led to the halt of the sale and distribution of the devices in 2019. In time, other countries, including the United Kingdom, Ireland, Australia, and New Zealand, issued similar orders for cessation of all use of polypropylene mesh products in surgeries. Although not as severe, synthetic meshes used in sacrocolpopexy are also associated with complications, at a rate of approximately 10.5% over 7 years, and this rate increases with time ( ). The pathogenesis of mesh complications is unclear; however, research studies suggest that mesh textile properties, mesh structural properties, and the mechanical behavior of mesh are all contributing factors.
Textile properties and implications for the host response
Pore size and porosity, mesh weight, knit pattern, and filament type are all key textile properties that can significantly impact the host response. In the following sections, each of these textile properties and the studies that have shed light on their role in the host response will be discussed. Important studies performed in humans and in animal models in both the abdominal hernia and urogynecology literature will also be discussed.
Filament type.
Synthetic meshes are manufactured from individual fibers that are either monofilament or multifilament. As the name implies, a multifilament fiber consists of multiple filaments that are either braided or interwoven to create one fiber. Relative to monofilament fibers, the surface area of multifilament fibers is increased by a factor of at least 1.57 ( ), and the increased surface area likely provides more area for bacteria to adhere and proliferate. Furthermore, multifilament fibers potentially have spaces less than 10 µm in diameter within the fibers themselves (as a result of the braided or interwoven filaments) that allow for the passage of bacteria but limit the passage of macrophages or neutrophils ( ). These spaces consequently act as a harbor for bacterial proliferation ( ). Indeed, synthetic meshes with multifilament fibers are associated with higher bacterial colonization ( ). Consequently, current meshes are manufactured from monofilament fibers, and multifilament fibers are avoided.
Knit pattern.
The knit pattern describes the way that the fibers are combined during the manufacturing process of the mesh, and governs the behavior of the mesh. Knitting and weaving are two of the most common knit patterns. To knit a mesh, a single fiber is continually looped into a warp-lock, interlock, or circular knit style. Conversely, a woven mesh is constructed from the interlacing of two sets of fibers that run perpendicular to one another. Relative to knitted meshes, woven meshes have superior shape memory and mechanical strength. However, they conform poorly to underlying tissues/structures and are susceptible to fraying when cut ( ). Knitted meshes, on the other hand, have increased flexibility, which affords them high conformity to underlying tissues/structures and greater porosity than woven meshes. The larger pores of knitted meshes reduce the risk of infection ( ; ; ), which will be discussed in more detail in the next section. Given these advantages, knitting is the preferred manufacturing technique for current urogynecologic meshes.
Pore size and porosity.
Pore size and porosity (the amount of void space relative to the total area of the mesh) are key textile properties that can significantly influence the host response to mesh. As alluded to in the previous section, large pores decrease the risk of bacterial colonization and increase vascular penetration relative to small pores. Additionally, large pores allow for the ingrowth of tissue, which can improve the mechanical integrity of the resulting mesh-tissue complex ( ). Similar to the small pores within multifilament fibers, mesh pores smaller than 10 µm are also conducive to bacterial proliferation and persistent infection ( ). Furthermore, small-pore, low-porosity meshes are associated with increased inflammation, fibrous tissue deposition, and potential for adhesion relative to large-pore, high-porosity meshes ( ; ; ; ; ). Small-pore meshes are also at an increased risk of undergoing “bridging fibrosis.”
Bridging fibrosis is a phenomenon that occurs when the perifiber inflammatory reaction around a single fiber merges or “bridges” with a neighboring fiber (or fibers in the case of a mesh knot), which results in the formation of a continuous fibrotic response or encapsulation of the mesh. This phenomenon is associated with an increase in pain, which is likely a result of tension on the surrounding tissues because of contraction of the myofibroblasts present in the capsule ( ; ; ; ; ). In addition to pore size, the thickness of the fibrous capsule is likely dependent on the polymer; more specifically in regards to how the tissue interacts with the surface of the fiber and the hydrophobicity of the polymer ( ). For polypropylene, 1 mm has been identified as the minimum pore diameter required to allow for adequate tissue ingrowth and to prevent bridging fibrosis ( ; ). However, it is important to note that 1 mm is specific to polypropylene meshes and hernia repair. The minimum pore diameter likely changes with the polymer (e.g., 630 µm is the minimal diameter for co-PVDF, a blend of polyvinylidene fluoride and hexafluorpropylene ( )) and for meshes implanted in the vagina, given that the host response to mesh is more robust in the vagina relative to the abdomen ( ; ).
Mesh weight and surface area.
In addition to filament type, knit pattern, and pore size, urogynecologic meshes are also classified into different categories based on weight, defined by the specific gravity (g/m 2 ), as lightweight (<45 g/m 2 ) or heavyweight (>45 g/m 2 ). In general, lightweight meshes tend to have larger pores, higher porosity, and decreased stiffness relative to heavyweight meshes. Consequently, lightweight meshes typically have improved biocompatibility compared with heavyweight meshes ( ). Specifically, heavyweight meshes are associated with increased apoptotic cells surrounding mesh fibers, a more pronounced and prolonged inflammatory response, and a higher turnover of the tissues surrounding the mesh fibers after 1 year of implantation ( ; ; ; ; ; ; ). Lightweight meshes, on the other hand, tend to have a reduced amount of fibrosis and inflammation, a higher potential for tissue incorporation within the mesh pores, and reduced potential for adhesion and pain ( ; ; ; ; ; ). Collectively, these results suggest that lightweight meshes are clinically more beneficial than heavyweight meshes, and it is for this reason that companies made the switch from producing heavyweight meshes to producing lightweight meshes, which is the current standard.
It is generally understood that lightweight meshes are more biocompatible than heavyweight meshes. However, contrasting results were observed in a study conducted by comparing a heavyweight (large-pore, knitted) and lightweight (small-pore, woven) polypropylene mesh implanted in the abdomen of rats. The heavyweight mesh was associated with a reduced fibrotic reaction, improved tissue integration, and significantly fewer macrophages at the implant surface than the lightweight mesh ( ). These results suggest that pore size is more important than mesh weight, and corroborate that knitted meshes are associated with a more favorable host response than woven meshes. However, it is important for the reader to keep in mind that mesh weight, pore size, and stiffness are interconnected, and that changing one property will affect the others. Thus, it is difficult to attribute the host response to a single factor, and it is vital that all aspects of urogynecologic meshes are considered when evaluating their biocompatibility. Moreover, as detailed below, the geometry of a mesh often changes according to the direction of loading. Indeed, wide-pored meshes with unstable geometries can be substantially smaller-pored and stiffer when loaded in vivo . It is therefore important to consider multiple factors when choosing a mesh.
Surface area, a measure of the amount of material that is in contact with the tissue, is another factor that can impact the host response to mesh ( ; ). Similar to heavyweight meshes, meshes with a larger surface area yield a strong and more active inflammatory response compared with meshes with a smaller area of contact with the host tissue ( ; ). Surface area is particularly problematic for multifilament meshes, as the surface area is increased by approximately 50% compared with monofilament meshes, thereby providing a greater surface for bacterial adherence and proliferation ( ).
Structural properties and implications for the host response
In the next few sections, the mechanics of meshes will be reviewed, with an emphasis placed on understanding the difference between structural and mechanical properties, the tests used to determine the structural properties of mesh, and the implications of structural properties for the host response.
Structural versus mechanical properties.
Structural and mechanical properties are two distinct properties that are evaluated through mechanical testing. Although these properties are related, the interpretation of structural and mechanical properties differs drastically. Structural properties describe how an applied force or deformation affects a material/device or collection of materials/devices (e.g., synthetic mesh). These properties are dependent on the type of material, the amount of material, the shape of the material, the direction of the applied force, and how the material is constrained and/or connected to other materials. Mechanical properties describe how an applied force or deformation affects a single material, and the response to the applied force or deformation is a result of the atomic and molecular interactions within the material (e.g., a single strand of polypropylene). Unlike structural properties, mechanical properties are not dependent on the size and shape of the material. For example, the structural properties of a large steel rod will be higher than that of a small steel rod; however, the mechanical properties will be the same because the rods are composed of the same material, steel.
Within the urogynecology literature, the following structural properties are commonly reported for meshes; ultimate load, ultimate elongation, relative elongation, stiffness, and energy absorbed. The ultimate load is the maximum amount of force that a mesh can withstand before failure, in which failure is defined as the permanent destruction of the mesh. The maximum change in length of the mesh before failure is defined as the ultimate elongation. Similarly, relative elongation is the maximum change in length of the mesh before failure, normalized to the initial length of the mesh. Stiffness describes the ability of the mesh to resist deformation and is the most commonly used term to describe urogynecologic meshes. Energy absorbed is the amount of energy that is required to bring a mesh to failure (i.e., it is a measure of toughness). As alluded to previously, the structural properties are dependent on the size and shape of the structure (i.e., mesh) being tested, whereas the mechanical properties are independent of size and shape.
In the urogynecology literature, stress, strain, tangent modulus/Young’s modulus, and strain energy density are often inappropriately reported when describing the properties of mesh. Synthetic meshes are discontinuous structures (i.e., they are filled with void spaces), and they cannot be treated as a continuum, which is a requirement of mechanical tensile tests. Thus, the size and shape of the mesh will dictate the properties, and only structural properties (e.g., load, elongation, stiffness, and energy absorbed) or normalized structural properties (e.g., relative elongation) should be reported for meshes. For additional information regarding mechanical properties, the interested reader should refer to .
Mechanical tests used to determine structural properties.
The structural properties of synthetic mesh are often determined using the same mechanical tests that are used to assess the properties of vaginal tissue. However, the applicability of the testing method, the experimental protocol, and the interpretation of the results will differ for mesh as a result of the structural differences between synthetic mesh and vaginal tissue. Synthetic meshes are highly porous, discontinuous materials, whereas vaginal tissues are continuous materials, meaning there is material at every location within the tissue, and the material is interconnected. It is important to note that the continuity of vaginal tissue is assumed at the fiber level. As stated above, the porous and discontinuous architecture of synthetic meshes restricts the use of mechanical testing that is only applicable to continuous materials. Additionally, the knit pattern restricts the type of testing that can be performed on synthetic meshes. Samples are often cut into a particular shape for a given mechanical test. Depending on the shape, cutting a synthetic mesh could disrupt the knit pattern and alter the mechanical behavior of the mesh, which will also impact the structural properties of the mesh. Lastly, the process of knitting a mesh creates interlaced fibers, which produces nonuniform and local deformations throughout the mesh structure. It is therefore important that the assumptions of a particular mechanical test and the interpretation of the results are thoroughly understood before performing any mechanical test on synthetic meshes.
Uniaxial tensile test.
The uniaxial tensile test is one of the mechanical tests that is most commonly used within the urogynecology field to evaluate the structural properties of synthetic meshes. As the name implies, a uniaxial tensile test involves loading mesh along one direction (typically the primary axis of the mesh) to measure the response of the mesh to the applied load. Unidirectional loading is similar to the loading conditions experienced by mesh implanted via a sacrocolpopexy. Specifically, mesh is clamped on opposing ends and is loaded at a constant rate until it fails, referred to as a “load to failure” test ( Fig. 7.2 A). The load and elongation are recorded and then used to create a load-elongation curve, which typically has a toe region (large amount of elongation with small changes in force) followed by a linear region (the relationship between load and elongation is relatively linear) and then a failure region (permanent damage to the mesh has occurred) ( Fig. 7.2 B). The load-elongation curve is then used to determine the structural properties: ultimate load, ultimate elongation, stiffness, and energy absorbed ( Fig. 7.2 C). For bilinear curves, the stiffness is often reported as low or high. The interested reader should refer to for a more in-depth explanation of the methodology and caveats to uniaxial tensile testing.

Ball-burst test.
The structural properties of synthetic mesh can also be obtained using ball-burst testing, which is also referred to as biaxial tensiometry. During ball-burst testing, a piece of mesh (typically square or circular) is securely sandwiched between two clamps (typically circular), and a steel ball head is pushed through the mesh at a constant rate until rupture (failure) ( Fig. 7.3 A). Similar to uniaxial tensile testing, the load and displacement are recorded and used to generate a load-extension curve, which has the characteristic toe, linear, and failure regions. The load-extension curve is then used to determine the ultimate load, ultimate extension, stiffness, and energy absorbed ( Fig. 7.3 B). The interested reader should refer to for a more in-depth explanation of the methodology and caveats to ball-burst testing.

Structural properties.
To date, all synthetic meshes are type I polypropylene (pore size >75 µm) meshes. They are knitted, have wide pores (most >1 mm, porosity >55%), and are lightweight (<45 g/m 2 ). However, the size and shape of the pores, as well as the knit pattern for each mesh, are distinct ( Fig. 7.4 ), which contributes to the differing structural properties of the mesh. The structural and textile properties are not independent of one another, as the stiffness, ultimate load, and energy absorbed, as determined by ball-burst testing, are shown to correlate with specific weight and porosity ( ). When comparing the structural properties of synthetic meshes, it is important to note that the structural properties obtained from a ball-burst test are higher than those obtained from a uniaxial test ( Table 7.2 ). This is a direct result of the boundary conditions imposed on the mesh. During a ball-burst test, the mesh is constrained along the entire perimeter, and the testing area of the mesh is small, which artificially increases the stiffness of the mesh. It is therefore important that the testing method used is considered when comparing the structural properties of different meshes.

STRUCTURAL PROPERTIES VIA UNIAXIAL TENSILE TEST | STRUCTURAL PROPERTIES VIA BALL-BURST TEST | ||||
---|---|---|---|---|---|
Synthetic Mesh | Low Stiffness (N/mm) | High Stiffness (N/mm) | Load at Mesh Failure (N) | Stiffness (N/mm) | Load at Mesh Failure (N) |
Boston Scientific Polyform | 0.130 ± 0.01 | 1.42 ± 0.11 | 53.8 ± 4.8 | 28 ± 0.43 | 108 ± 5.7 |
Coloplast NovaSilk | 0.072 ± 0.05 | 0.508 ± 0.09 | 19.6 ± 4.5 | 16 ± 5.5 | 54 ± 19 |
Ethicon Gynemesh PS (Prolift) | 0.286 ± 0.02 | 1.37 ± 0.09 | 46.3 ± 2.6 | 28 ± 2.7 | 108 ± 8.6 |
Ethicon Ultrapro (Prolift + M) | 0.009 ± 0.00 | 0.236 ± 0.02 | 7.83 ± 0.7 | 22 ± 2.8 | 76 ±12 |
Coloplast Restorelle (Mpathy Medical Smartmesh, Minimesh) | 0.178 ± 0.03 | 0.592 ± 0.04 | 22.7 ± 1.8 | 11 ± 0.89 | 45 ± 3.8 |
The structural properties of slings obtained via uniaxial tensile testing also differ ( Table 7.3 ) ( ). Similar to synthetic meshes, the differing structural properties occur as a result of the distinct knit pattern, as well as the size and shape of the pores within each device. It is important for the interested reader to note that uniaxial tensile testing is preferred over ball-burst testing to determine the structural properties of slings, given the dimensions (aspect ratio) of slings.
STRUCTURAL PROPERTIES VIA UNIAXIAL TENSILE TEST | ||||
---|---|---|---|---|
Sling | Low Stiffness (N/mm) | High Stiffness (N/mm) | Load at Sling Failure (N) | Relative Elongation at Sling Failure (%) |
American Medical Systems SPARC/Monarc | 0.09 ± 0.03 | 1.7 ± 0.2 | 79.2 ± 5.5 | 115.2 ± 7.4 |
Bard Uretex | 0.16 ± 0.07 | 1.2 ± 0.1 | 59.8 ± 5.1 | 92.3 ± 6.7 |
Boston Scientific Advantage/Obtryx | 0.05 ± 0.03 | 1.9 ± 0.1 | 69.6 ± 8.3 | 107.3 ± 10.0 |
Caldera T-sling | 1.2 ± 0.3 | N/A | 82.0 ± 15.0 | 105.9 ± 4.9 |
Gynecare TVT/TVT-O | 0.09 ± 0.01 | 2.0 ± 0.3 | 73.5 ± 11.8 | 108.1 ± 4.5 |
Mentor Aris | 1.5 ± 0.4 | N/A | 56.3 ± 7.0 | 42.7 ± 5.5 |
Stiffness and the host response.
From a functional perspective, the ideal synthetic mesh should be strong and stiff enough to withstand and maintain its architectural geometry and structure in response to in vivo loads. As one can imagine, a mesh that is not strong enough could break (mesh failure), which would result in prolapse recurrence. Similarly, prolapse recurrence could occur if the mesh is not stiff enough and permanently elongates with tensioning and loading. It is also important that synthetic meshes are not so strong or so stiff that they impede normal physiologic function (e.g., the ability of the vagina to elongate, contract, etc.) or affect the normal structure of the vagina (e.g., vaginal thickness and smooth muscle morphology). Specifically, meshes that are too stiff can lead to stress shielding.
Stress shielding is a phenomenon that occurs when there are stiffness mismatches between two interconnected materials. The stiffer material bears the majority of physiologic loads, thereby “shielding” the less stiff material from experiencing the loads it normally experiences, which can lead to a maladaptive remodeling response, resulting in degeneration and atrophy ( ; ; ; ). A classic example of stress shielding is the degeneration of the surrounding hip bone after the implantation of a stiff artificial joint. Studies have shown evidence of stress shielding within the urogynecology field. The implantation of synthetic meshes with varying amounts of stiffness onto nonhuman primate (rhesus macaque) vaginas via abdominal sacrocolpopexy negatively impacted the vagina. However, the stiffest mesh was associated with the greatest negative impact on the vagina. Stress shielding led to thinning of the vaginal smooth muscle ( Fig. 7.5 ), degradation of collagen and elastin, and increased apoptosis in the area of the mesh fibers ( ). Ongoing tissue degradation was evidenced by an increase in active matrix metalloproteinases (MMPs) MMP-1, MMP-8, and MMP-13, and total MMP-2 and MMP-9 ( ). Additionally, deterioration of the mechanical properties, particularly the ability of the vagina to contract, was significantly impaired with the implantation of the stiffest mesh. Collectively, these results suggest that stress shielding induced by the implantation of polypropylene mesh leads to vaginal degeneration and atrophy.

Characterization of the ex vivo mechanical behavior of synthetic meshes and implications for the host response
The mechanical behavior of synthetic meshes is a broad term that describes how a mesh (i.e., the architectural geometry of the pores and the overall structure of the mesh) responds to various deformations, loads, and boundary conditions. Mechanical tests, as well as computational modeling, have played an instrumental role in providing insight into how mesh behavior may impact the host response to mesh.
Anisotropy.
Synthetic meshes are typically designed by manufacturers such that there is an intended orientation in which the mesh should be implanted. For some urogynecologic and hernia meshes, the structural properties will vary depending on the direction in which the mesh is deformed or loaded, a phenomenon referred to as anisotropy ( ; ). UltraPro (marketed as Prolift+M by Ethicon, Somerville, NJ, United States) is an example of a mesh that is anisotropic. The structural stiffness of UltraPro when loaded perpendicular to the blue orientation lines (UltraPro perpendicular, the intended orientation of implantation) is 0.009 ± 0.002 N/mm, whereas the stiffness is orders of magnitude higher when UltraPro is loaded parallel to the blue orientation lines (UltraPro parallel, 0.258 ± 0.085 N/mm) ( ). The implantation of UltraPro in the perpendicular orientation was associated with less of a negative impact on the nonhuman primate vagina compared with the parallel orientation, owing to the decreased stiffness ( ). Given the significant impact that stiffness can have on the host response to mesh, understanding the mechanical behavior of synthetic meshes, including anisotropy, would provide surgeons with a better understanding of mesh behavior before implantation. Such knowledge would also provide guidance on the appropriate direction of implantation, leading to improved outcomes for patients.
Pore collapse via tensile loading.
Ex vivo mechanical testing and computational modeling of synthetic meshes have demonstrated that the geometry of most meshes changes substantially when loaded. In regard to planar deformation, the pores collapse, and the porosity is significantly reduced for the majority of current synthetic meshes with uniaxial loading. This behavior is observed for both transvaginal and sacrocolpopexy meshes and occurs at loads that are well within the physiologic range. Otto et al. observed significant pore collapse associated with a loss of porosity within the arms of transvaginal meshes when elongated in response to relatively small loads ( ). Similarly, observed that the pores in the arms of a transvaginal mesh, as well as those within the central body of the mesh (between the superior arms), are most susceptible to a reduction in pore size and a loss of porosity. Indeed, the arms and the central body of transvaginal meshes have been identified as problematic areas for patients experiencing mesh complications (e.g., mesh shrinkage/contraction, tenderness upon palpation of contracted mesh, pain, dyspareunia) ( ). Pore collapse, a reduction in porosity, and a complete loss in porosity (for some meshes) are also observed for sacrocolpopexy meshes ( ). Regardless of the type of mesh (vaginal or abdominal), the degree of pore collapse is driven by the pore geometry, with square pore geometry being the most stable. The amount of pore collapse is also impacted by the degree to which the mesh is elongated or loaded, with increasing elongation and load resulting in increasing pore collapse ( Fig. 7.6 ). Depending on the degree of pore collapse, the diameter of the pores can decrease to below 1 mm, which would make urogynecologic meshes more susceptible to poor tissue integration, bridging fibrosis, encapsulation, and pain. Clinically, mesh explants removed from patients experiencing mesh complications often exhibit mesh pore collapse with little to no tissue integration between the pores ( Fig. 7.7 ). Collectively, these results and observations suggest that maintaining an open pore geometry is critical and future studies are needed to substantiate that pore collapse leads to mesh complications.
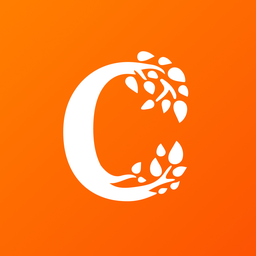
Full access? Get Clinical Tree
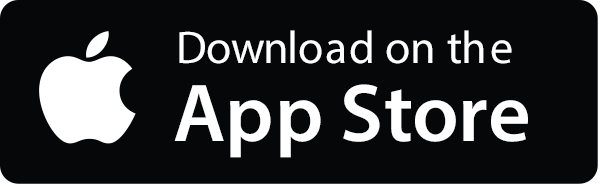
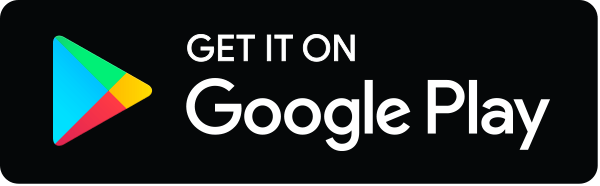