and Roger F. Soll9
(8)
Department of Neonatology, State University of New York at Buffalo and ONY, Inc., Amherst, NY, USA
(9)
Department of Pediatrics and Environmental Medicine, University of Rochester, Rochester, NY, USA
Educational Aims
-
Understand the composition, metabolism, and physiology of pulmonary surfactant
-
Understand the effect of surfactant in infants with surfactant deficiency or dysfunction
-
Understand the optimal timing and methods of surfactant administration
28.1.1 Introduction
Exogenous pulmonary surfactant, widely used in neonatal care, is one of the best-studied treatments in neonatology, and its introduction in the 1990s led to a significant improvement in neonatal outcomes in preterm infants, including a decrease in mortality. This chapter provides an overview of surfactant composition and function in health and disease and summarizes the evidence for its clinical use.
28.1.2 Surfactant Composition, Metabolism, Physiology, and Pathophysiology
28.1.2.1 Surfactant Composition and Metabolism
The alveoli of all mature mammals are lined with pulmonary surfactant, a lipoprotein that reduces surface tension and prevents alveolar collapse. The constituents of surfactant are phospholipids (80 %), neutral lipids (8 %), and proteins (20 %). Among the phospholipids, the predominant one (60 %) is dipalmitoylphosphatidylcholine (DPPC), with lesser amounts of unsaturated phosphatidylcholine compounds, phosphatidylglycerol, and phosphatidylinositol. The proteins consist of four unique surfactant-associated apoproteins. Two of these, SP-A and SP-D, are hydrophilic proteins and belong to a subgroup of mammalian lectins called collectins. The other two, SP-B and SP-C, are hydrophobic proteins.
Surfactant is produced in the type II cells of the alveoli (Fig. 28.1), which differentiate between 24 and 34 weeks of gestation in the human. It is assembled and stored in the lamellar bodies, which are concentric or parallel lamellae of phospholipid bilayers. Lamellar bodies are extruded into the fluid layer lining the alveoli by exocytosis and form long stacked tubes composed mainly of phospholipid bilayers called tubular myelin. On cross section, tubular myelin has a lattice-like structure because the corners of the component tubes appear fused. Tubular myelin enters the air–water interface quickly by adsorption and greatly reduces the surface tension of that interface (Hallman 2004). It is the major source of the monolayer surface film lining the air–liquid interface in the alveoli. In this monolayer the hydrophobic fatty acyl groups of the phospholipids extend into the air, while the hydrophilic polar head groups bind water (Possmayer et al. 1984).
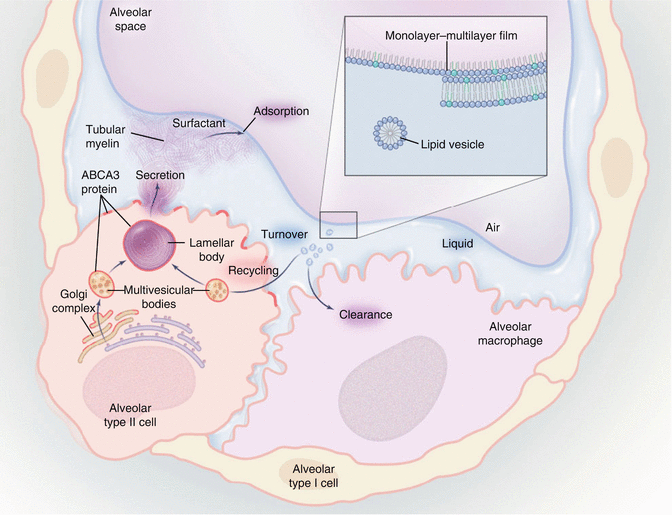
Fig. 28.1
Lung surfactant and the lamellar body. The composition of lung surfactant is critical to lung function, and the subcellular lamellar body of the type II alveolar cell has a major role in maintaining the composition of surfactant. The distribution of ATP-binding cassette (ABC) transporter A3 (ABCA3) is shown in red. It is possible that ABCA3 targets surfactant-containing vesicles to the lamellar bodies. The lamellar body is formed through the fusion of several multivesicular bodies, and its lipid bilayers are converted to tubular myelin as they are turned out into the alveolar space. Surfactant protein A, secreted primarily by a constitutive pathway that does not involve the lamellar body, is required for the formation of tubular myelin. Surfactant aggregate, such as tubular myelin, is the precursor of surfactant at the air–water interface (inset). Cationic transmembrane proteins (surfactant protein B or surfactant protein C or both (not depicted in the monolayer–multilayer film)) together with anionic phospholipids (phosphatidylglycerol or phosphatidylinositol or both (green)) facilitate the entry of dipalmitoylphosphatidylcholines (blue) into the monolayer at the interface, maintaining a low surface tension. During tidal breathing, tubular myelin surfactant is converted to smaller aggregates that are taken up by type II cells and alveolar macrophages. Arrows depict the direction of surfactant flux
The total pulmonary surfactant content can be divided into an intra-alveolar and an intracellular pool (Nkadi et al. 2009). However, the total surfactant pool size is not equivalent to the amount of active surfactant. Maintaining an adequate intra-alveolar surfactant pool is essential for lung function and is dependent on the dynamic cycle of surfactant metabolism (Nkadi et al. 2009). Surfactant pool size increases in late pregnancy, followed by a gradual decrease after birth to adult values (Zimmermann et al. 2005). During and shortly after birth, large amounts of surfactant are released into the alveolar space (Zimmermann et al. 2005). Among different species, humans have the smallest alveolar pool sizes, whereas the amount of saturated phosphatidyl choline in lung tissue is similar across species. The lower alveolar surfactant pool size makes the human lung particularly vulnerable to surfactant dysfunction in case of lung injury. The surfactant pool size in preterm infants with respiratory distress syndrome (RDS) is around 10 mg/kg or less, while healthy term infants have an estimated surfactant pool size of 100 mg/kg (Nkadi et al. 2009). The rate of synthesis of surfactant in preterm infants is also low (Zimmermann et al. 2005).
Alveolar surfactant can be cleared by different pathways. All the main components of surfactant (DPPC, PG, SP-A, SP-B, and SP-C) are recycled. The phospholipid from the monolayer eventually reenters the type II cells through endocytosis and forms multivesicular bodies, which are then either incorporated into lamellar bodies (“recycled”) or degraded in lysosomes. Degraded surfactant components are also used to synthesize new surfactant lipids or proteins. Finally, surfactant can be removed from the lung, either as intact molecules or as degraded products (Zimmermann et al. 2005; Jobe and Ikegami 1993).
Control of surfactant sroduction: Surfactant secretion can be stimulated by a number of mechanisms. Type II cells have beta-adrenergic receptors and respond to beta-agonists with increased surfactant secretion (Nkadi et al. 2009). Purines, such as adenosine triphosphate are potent stimulators of surfactant secretion and may be important for its secretion at birth. Mechanical stretch, such as lung distension and hyperventilation, has also been found to be involved in stimulating surfactant secretion (Nkadi et al. 2009). Hormones also play a role in surfactant secretion. Thyroxine accelerates type II cell differentiation while acting synergistically with glucocorticoids to enhance lung compliance and DPPC synthesis. Finally, both prenatally administered maternal glucocorticoids and exogenous surfactant administration after birth stimulate endogenous surfactant synthesis.
28.1.2.2 Surfactant Physiology
28.1.2.2.1 In the Normal Lung
Pulmonary alveoli are bubble shaped, with a high degree of curvature. The attraction between the molecules in the alveolar fluid of the moist inner surface of the alveoli generates surface tension and tends to make the alveoli collapse. Unchecked, this tendency would result in lung collapse. Surfactant greatly reduces the surface tension at the air–liquid interface in the alveoli and distal bronchioles. This prevents the alveoli from collapsing during expiration and promotes lung expansion during inspiration.
The main component responsible for decreasing the surface tension is DPPC. However it adsorbs very slowly to air–liquid interfaces and therefore requires surfactant proteins or other lipids to facilitate its adsorption. SP-B and SP-C enhance spreading of phospholipid in the airspaces. SP-B promotes phospholipid adsorption and induces the insertion of phospholipids into the monolayer, thus enhancing the formation of a stable surface film (Creuwels et al. 1997). SP-C enhances phospholipid adsorption, stimulates the insertion of phospholipids out of the subphase into the air–liquid interface, and may increase the resistance of surfactant to inhibition by serum proteins or by edema fluid (Creuwels et al. 1997; Griese 1999).
As the alveolar surface expands during inspiration, surfactant components insert from the hypophase (epithelial lining fluid) into the monolayer. At expiration the alveolar surface reduces and the monolayer is compressed, thereby squeezing out some surfactant proteins, unsaturated PC, and other lipids. By this mechanism, the monolayer comprises mainly of DPPC, the most important surface-tension-lowering component during compression (Zimmermann et al. 2005).
Surfactant also has a role in pulmonary host defense. SP-A and SP-D may play important roles in the defense against inhaled pathogens, and SP-A may have a regulatory function in the formation of the monolayer that lowers the surface tension (Creuwels et al. 1997).
28.1.2.2.2 In the Premature Lung
The preterm infant with respiratory distress syndrome (RDS) has immaturity of the lungs, especially of the type II cells, and decreased synthesis of surfactant. This results in low amounts of surfactant in the alveoli (surfactant pool size of 2–10 mg/kg) (Zimmermann et al. 2005) that contains a lower percent of disaturated phosphatidylcholine species, less phosphatidylglycerol, and less of all the surfactant proteins than surfactant from a mature lung. Minimal surface tensions are also higher for surfactant from preterm than term infants (Nkadi et al. 2009). However, preterm infants may have increased recycling of surfactant when compared to term infants (Zimmermann et al. 2005).
Shortly after birth, infants with RDS develop tachypnea, grunting, nasal flaring, use of accessory muscles of respiration, intercostal or subcostal retractions, cyanosis, poor feeding, and apnea. A chest radiograph typically shows a diffuse reticulogranular “ground glass” opacification (the result of diffuse alveolar atelectasis) with superimposed air bronchograms (Nkadi et al. 2009). The lungs of infants who die from RDS show alveolar atelectasis, alveolar and interstitial edema, and diffuse hyaline membranes in distorted small airways (Nkadi et al. 2009). RDS is one of the most common causes of death and morbidity in preterm neonates. It occurs worldwide with a slight male predominance (Nkadi et al. 2009). Prenatal corticosteroids significantly reduce the incidence, severity, and mortality associated with RDS.
28.1.2.2.3 In the Injured Neonatal Lung
Surfactant dysfunction can develop secondary to a variety of other conditions that result in lung injury in neonates, such as meconium aspiration syndrome, pulmonary hemorrhage, and pneumonia.
In meconium aspiration syndrome, the mechanisms underlying surfactant inactivation are not fully understood, but it has been shown that meconium destroys the fibrillary structure of surfactant and decreases its surface adsorption rate (Nkadi et al. 2009). In vitro studies (Moses et al. 1991; Clark et al. 1987) and animal studies (Sun et al. 1993; Davey et al. 1993) have demonstrated that meconium inhibits surfactant function and is likely to be partially responsible for alveolar collapse in meconium aspiration syndrome. Components of meconium that may contribute to altered surfactant function include cholesterol, free fatty acids, bile salts, bilirubin, and proteolytic enzymes (Moses et al. 1991; Clark et al. 1987; Sun et al. 1993; Lieberman 1966). In particular, phospholipase-A2 (PLA2) in meconium has been found to inhibit the activity of surfactant in vitro in a dose-dependent manner, through the competitive displacement of surfactant from the alveolar film (Nkadi et al. 2009). PLA2 is also known to induce hydrolysis of DPPC, releasing free fatty acids and lyso-PC which damage the alveolar–capillary membrane and induce intrapulmonary sequestration of neutrophils (Nkadi et al. 2009).
In pulmonary hemorrhage, capillary filtrate builds up in the interstitial space and can burst through the pulmonary epithelium into the airspaces. Neutrophils are released following endothelial damage and they, in turn, express proteases, oxygen free-radicals, and cytokines. These free oxygen molecules damage the type II cells that produce surfactant proteins, thus inhibiting production of the proteins (Nkadi et al. 2009). Elastase, one of these proteases, damages and degrades SP-A, thereby inhibiting SP-A-mediated surfactant lipid aggregation and adsorption in vitro (Nkadi et al. 2009).
Acute respiratory distress syndrome (ARDS) is a significant cause of morbidity and mortality in all age groups following infection (the most common cause), hemorrhage, or other forms of lung injury. It is defined as a severe form of acute lung injury (ALI) and a syndrome of acute pulmonary inflammation. ALI/ARDS is characterized by sudden onset, impaired gas exchange, decreased static compliance, and a non-hydrostatic pulmonary edema (Nkadi et al. 2009). ARDS is characterized by an increase in the permeability of the alveolar–capillary barrier due to injury to the endothelium and/or alveolar lining cells. Damage to the alveolar type I cells leads to an influx of protein-rich edema fluid into the alveoli, as well as decreased fluid clearance from the alveolar space. Neutrophils are attracted into the airways by host bacterial and chemotactic factors and express enzymes and cytokines which further damage the alveolar epithelial cells (Nkadi et al. 2009). Type II epithelial cell injury leads to a decrease in surfactant production, with resultant alveolar collapse.
28.1.2.2.4 Effect of Mechanical Ventilation on Pulmonary Surfactant
Mechanical ventilation in itself can worsen lung disease and effect surfactant function. The damage caused by mechanical ventilation can cause fluid, protein, and blood to leak into the airways, alveoli, and the lung interstitium, interfering with lung mechanics, inhibiting surfactant function, and promoting lung inflammation (Clark et al. 2001). Even a short period of mechanical ventilation can cause a decrease in lung compliance that is associated with a large influx of proteins into the alveolar space and with alterations in the pulmonary surfactant system (Veldhuizen et al. 2000). The changes of surfactant in these experiments are different from those seen in acute lung injury, indicating that they may represent an initial response to mechanical ventilation.
In the adult lung, injuries due to mechanical ventilation occur primarily when the sum of the functional residual capacity (FRC) and tidal volume approach or exceed maximal lung volume (Dreyfuss and Saumon 1993). The preterm lung is particularly susceptible to injury by mechanical ventilation because of structural immaturity, and tidal volumes considered safe for the adult may approach the maximum lung volumes in the preterm lung (Wada et al. 1997). Initiation of ventilation in preterm lambs with high volumes causes lung injury and decreases the subsequent response to surfactant treatment (Wada et al. 1997).
In premature baboons, mechanical ventilation results in abnormal surfactant metabolism (Seidner et al. 1998). In the normal lung, large lipid arrays or large-aggregate forms of surfactant are the source of the surface film (Wright 1990). Small vesicles, primarily containing lipids, reenter the hypophase for recycling or catabolism. The amount of inactive vesicular forms increases with lung injury, and this increase is associated with a deterioration in lung mechanics.
Long-term ventilation of the lungs in immature preterm infants with respiratory distress leads to ventilator-induced lung injury and chronic lung disease. Ventilation of these infants not only interferes with alveolarization but also with the surfactant system. Ventilated preterm animals accumulated very large lipid pools in tissue, but alveolar pools stay relatively low indicating decreased secretion of newly synthesized saturated phosphatidylcholine and increased catabolism. These effects of both acute and long-term ventilation leave the preterm infant particularly susceptible to problems associated with surfactant deficiency and dysfunction.
28.1.3 Exogenous Surfactant Therapy
28.1.3.1 Types of Exogenous Surfactant
There are two broad categories of exogenous surfactant available for treatment: animal-derived surfactants and synthetic surfactants.
28.1.3.1.1 Animal-Derived Surfactants
These include the following:
-
Bovine surfactant obtained by lung mince: Beractant (Survanta) and Surfactant-TA (Surfacten) are lipid extracts of bovine lung mince with added DPPC, tripalmitoylglycerol, and palmitic acid.
-
Bovine surfactant obtained by lung lavage: Calf lung surfactant extract (CLSE, calfactant, Infasurf), SF-RI1 (Alveofact), and bovine lipid extract surfactant (BLES) are bovine lung washes subjected to chloroform–methanol extraction.
-
Porcine surfactant obtained by lung mince: Poractant (Curosurf) is a porcine lung mince that has been subjected to chloroform–methanol extraction and further purified by liquid–gel chromatography. It consists of approximately 99 % polar lipids (mainly phospholipids) and 1 % hydrophobic, low molecular weight proteins (SP-B and SP-C) (Wiseman and Bryson 1994).
28.1.3.1.2 Synthetic Surfactants
These include the following:
Protein-free synthetic surfactants: There is one product in this category, colfosceril palmitate, cetyl alcohol, tyloxapol (Exosurf), and it consists of 85 % dipalmitoylphosphatidylcholine (DPPC), 9 % hexadecanol, and 6 % tyloxapol (a spreading agent). Another product in this category, pumactant (also known as artificial lung expanding compound, ALEC) is no longer manufactured (Halliday 2006) and was a 7:3 mixture of DPPC and phosphatidyl glycerol. These synthetic surfactants lack many of the components of animal-derived surfactant, particularly the hydrophobic surfactant proteins B and C.
Protein-containing synthetic surfactants: These surfactants contain synthetic phospholipids along with proteins (produced through peptide synthesis and recombinant technology) that attempt to mimic the function of either SP-B or SP-C. Of these, lucinactant (Surfaxin) contains dipalmitoylphosphatidylcholine, palmitoyl oleoylphosphatidyl glycerol, and palmitic acid (Cochrane et al. 1996, 1998) combined with a mimic of SP-B called sinapultide or KL4 peptide. KL4 is a 21-residue peptide comprised of repeated units of four hydrophobic leucine (L) residues, bounded by basic polar lysine (K) residues arranged in the following order: KLLLLKLLLLKLLLLKLLLLK. This structure resembles the repeating pattern of hydrophobic and hydrophilic residues in the C-terminal part of SP-B and stabilizes the phospholipid layer by interactions with the lipid heads and the acyl chains (Cochrane and Revak 1991). Another synthetic SP-B analog currently under testing is called dSP-B1-25,which resembles the N-terminal segment of SP-B and when combined with synthetic phospholipids has shown some efficacy in animal studies.
Another type of synthetic protein-containing surfactant is called rSP-C surfactant or lusupultide (Venticute). It contains DPPC, palmitoyl oleoylphosphatidyl glycerol, palmitic acid, and calcium chloride (Hafner and Germann 2000; Spragg et al. 2000) combined with a recombinant SP-C analog (rSP-C), which is similar to the 34-amino acid human SP-C sequence, except that it contains cysteine (in place of phenylalanine) in positions 4 and 5 and contains isoleucine (instead of methionine) in position 32.
During surfactant replacement therapy, exogenous surfactants are given at doses between 10 and 20 times the usual pool sizes found in preterm infants with RDS, which approximates the pool size in term infants (Nkadi et al. 2009). The table summarizes the individual characteristics of each of these products, including the dosage, volume, and the recommended repeat dosing interval. All these products are administered intratracheally with each dose divided into multiple aliquots and should be administered according to the manufacturers’ recommendations (Table 28.1).
Table 28.1
Products available for exogenous surfactant therapy
Name of product (pharmaceutical name) |
Source |
Phospholipid (mg/ml) |
Dose (volume) (ml/kg) |
Dose (mg/kg of phospholipid) |
Repeat dosing interval (h) |
---|---|---|---|---|---|
Animal–derived products | |||||
Beractant (Survanta) |
Lipid extract of bovine lung mince with added DPPC, tripalmitoylglycerol and palmitic acid |
25 |
4 |
100 |
6 |
Calfactant (Infasurf) |
Bovine lung wash subjected to chloroform–methanol extraction |
35 |
3 |
100 |
6–12 |
SF-RI1 (bovactant, Alveofact) |
Bovine lung wash subjected to chloroform–methanol extraction |
50 |
1–2 |
50–100 |
8 |
bLES (BLES) |
Bovine lung wash subjected to chloroform–methanol extraction |
27 |
5 |
135 |
6 |
Poractant (Curosurf) |
Porcine lung mince that has been subjected to chloroform–methanol extraction and further purified by liquid–gel chromatography |
80 |
1.25–2.5 for initial dose, 1.25 for subsequent doses |
100–200 |
12 |
Protein–free synthetic surfactants | |||||
Colfosceril palmitate, hexadecanol, tyloxapol |
85 % dipalmitoylphosphatidylcholine (85 %), hexadecanol (9 %), and tyloxapol (a spreading agent, 6 %) |
13.5 |
5 |
67.5 |
12 |
Protein–containing synthetic surfactants | |||||
Lucinactant (Surfaxin) (Moya et al. 2005) |
Synthetic phospholipids and proteins produced through peptide synthesis and recombinant technology. Contains a mimic of SP-B called sinapultide or KL4 peptide |
30 |
5.8 |
175 |
6 |
Recombinant SP-C surfactant (Venticute)a |
Synthetic phospholipids and proteins produced through peptide synthesis and recombinant technology. Contains recombinant SP-C (rSP-C) combined with DPPC, palmitoyl oleoylphosphatidyl glycerol, palmitic acid, and calcium chloride |
50 |
1–2 |
50–100 |
4 |
28.1.3.2 Administration of Exogenous Surfactant: Practical Issues
The dynamics of how exogenously administered surfactant might spread through the airways into the alveoli have been well studied in the laboratory and theoretical models created (Halpern et al. 1998, 2008). When a bolus of surfactant is instilled and propagates down the airways, it deposits a liquid layer that coats the airways. The bolus may rupture because it may not pick up as much fluid as it is depositing and therefore may not reach the terminal bronchioles and alveoli where it is needed. However, the deposited liquid layer that is left behind may still advance due to the effects of gravity and surface tension, especially as the liquid layer thins.
The potential magnitude of uneven distribution of exogenously administered surfactant has been emphasized by Jobe (2006), who points out that there are 20 generations of airway branching from the trachea to the respiratory bronchioles and saccules, with 250,000 binary branch points and 500,000 distal airways leading to saccules. If the distribution is not proportionate to the number of saccules beyond each branch point, surfactant distribution will not be uniform. Any nonuniformity at a proximal branch point will be amplified at subsequent branch points. When exogenous surfactant is administered in clinical practice, its distribution is not ideal, but is often good enough because of the biophysical properties of surfactant and the small amount that is needed regionally in the lung for a treatment response (Jobe 2006).
There are several variables that contribute to the distribution of surfactant in the lungs (Jobe 2006): surface activity causes rapid adsorption and spreading, gravity contributes to the distribution of surfactant in large airways, a higher volume of surfactant and faster administration cause better distribution, positive pressure ventilation and positive end-expiratory pressure help clear the airways of fluid, and higher volumes of fetal lung fluid or edema fluid improve distribution. Therefore techniques to improve surfactant distribution include positioning the infant to minimize gravity, giving surfactant quickly in a reasonable volume, and giving the infant enough ventilator support to quickly clear the airways of fluid.
According to the manufacturers’ recommendations, beractant and poractant should be administered through a catheter inserted into the endotracheal tube, colfosceril should be administered through a side-port adapter attached to the endotracheal tube, and calf lung surfactant extract can be administered either through a feeding catheter or through a side-port adapter. Other methods of administration of surfactant have been tested in randomized trials as well and are described below.
Administration Through Catheter, Side Port, or Suction Valve: In a randomized trial, the administration of beractant through a catheter inserted through a neonatal suction valve without detachment of the neonate from the ventilator was compared to the administration of the dose (with detachment from the ventilator) in two aliquots through a catheter and to the standard technique of administration of the dose in four aliquots through a catheter (Zola et al. 1993a). Administration through the suction valve led to less dosing-related oxygen desaturation but more reflux of beractant than the two aliquot catheter technique. In another study (Soler et al. 1997), the administration of poractant as a bolus was compared in a randomized trial to administration via a catheter introduced through a side hole in the tracheal tube adaptor without changing the infants’ position or interrupting ventilation. The numbers of episodes of hypoxia and/or bradycardia as well as other outcomes were similar in both groups. A slight and transient increase in PaCO2 was observed in the side-hole group.
Administration Through Dual-Lumen Endotracheal Tube: The administration of poractant through a dual-lumen endotracheal tube without a change in position or interruption of mechanical ventilation was compared to bolus instillation in a randomized trial (Soler et al. 1998). The dual-lumen group had fewer episodes of dosing-related hypoxia, a smaller decrease in heart rate and SaO2, and a shorter total time in supplemental oxygen than the bolus group. The dual-lumen method has also been compared to the side-port method of administration of colfosceril in a randomized trial (Nelson et al. 1997). No difference was found between the two methods in dosing-related hypoxemia.
Slow Infusion Versus Bolus Administration: In one randomized clinical trial (Sitler et al. 1993), the slow infusion of colfosceril using a microinfusion syringe pump over 10–20 min was compared to manual instillation over 2 min. Pump administration resulted in fewer infants with loss of chest wall movement during dosing as well as a lesser increase in peak inspiratory pressure than with hand administration. In another small clinical trial of preterm infants (Zola et al. 1993b), there were no differences in clinical outcomes with administration by bolus versus slow infusion. However in animals, slow infusion of surfactant into the endotracheal tube results in nonhomogeneous distribution of surfactant in the lung (Ueda et al. 1994; Segerer et al. 1993). Because the evidence about the best method of administration is scant, and because bolus administration is likely to lead to better distribution, bolus administration of surfactant is preferred.
Other Methods: Other methods of administration such as nebulization or aerosolization (Berggren et al. 2000; Dijk et al. 1998; Ellyett et al. 1996; Fok et al. 1998; Jorch et al. 1997) and in utero administration to the human fetus (Cosmi et al. 1996; Petrikovsky et al. 1995) have also been reported. These methods require further clinical testing and are not currently recommended.
Chest Position During Administration of Surfactant: In a study in rabbits, pulmonary distribution of intratracheally instilled surfactant was largely determined by gravity, and changing the chest position after instillation did not result in any redistribution of the surfactant. Therefore, for neonates receiving surfactant, keeping the chest in the horizontal position may result in the most even distribution of the surfactant in the two lungs (Broadbent et al. 1995).
In summary, based on available evidence, surfactant should be administered in the standard method of aliquots instilled into an endotracheal tube. There is evidence to suggest that the administration of surfactant using a dual-lumen endotracheal tube or through a catheter passed through a suction valve is effective and may cause less dosing-related adverse events than standard methods. The side-port method of administration and the catheter method of administration appear to be equivalent. More studies are required before firm conclusions can be drawn about the optimal method of administration of surfactant and whether the optimal method is different for different types of surfactant.
28.1.3.3 Clinical Use of Surfactant Therapy in Preterm Infants with RDS
Exogenous surfactant therapy is one of the best-studied therapies in neonatology and numerous randomized controlled trials have been performed comparing various treatment regimens and strategies. The findings from these trials, many of which are summarized in multiple systematic reviews in the Cochrane Database of Systematic Reviews (Sinclair et al. 2003), are described in the following sections. The results of the meta-analysis in these reviews are expressed as typical relative risk (RR) and typical absolute risk difference (ARD), with 95 % confidence intervals (CI) for each of these.
It is useful to clarify the terminology used for various treatment strategies with surfactant.
Prophylactic surfactant therapy: Administration of exogenous surfactant immediately after birth to an infant who is at risk of developing RDS, but may or may not already have clinical features of RDS.
Rescue (or selective) surfactant therapy: Administration of exogenous surfactant to an infant who has already developed clinical features of RDS.
Many clinical trials in the late 1980s and early 1990s studied the effects of rescue and prophylactic surfactant therapy compared to placebo or no therapy. Systematic reviews of these trials show that, compared to placebo or no therapy, surfactant treatment or prophylaxis (with either animal-derived or synthetic surfactant) decreases the risk of pneumothorax and of mortality. Estimates from the meta-analyses indicate that there is a 30–65 % relative reduction in the risk of pneumothorax and up to a 40 % relative reduction in the risk of mortality. There were no consistent effects on other clinical outcomes such as chronic lung disease, patent ductus arteriosus, and intraventricular hemorrhage.
Further evidence of the benefits of surfactant therapy is derived from studies demonstrating decreased mortality and morbidity in very low birth weight infants following the introduction of surfactant therapy into practice (Schwartz et al. 1994; Lee et al. 1999; Philip 1995; Doyle et al. 1999; Hamvas et al. 1996; Horbar et al. 1993a; Hoekstra et al. 1994).
28.1.3.3.1 Efficacy of Surfactant Therapy in Established RDS (Rescue Surfactant Therapy)
Many of the early surfactant trials studied the effects of surfactant treatment in preterm infants with clinical and/or radiologic features of RDS (rescue or treatment trials). Some of these studies used animal-derived surfactant and others used protein-free synthetic surfactant.
Rescue Therapy with Animal-Derived Surfactant: In a systematic review and meta-analysis of 13 randomized trials of animal-derived surfactant (Seger and Soll 2009), infants treated with surfactant had a rapid improvement in respiratory status (improved oxygenation and decreased need for ventilator support), as well as a significant decrease in the risk of (a) any air leak (typical RR 0.47, 95 % CI 0.39–0.58; typical ARD −0.16, 95 % CI −0.21 to −0.12), (b) pneumothorax (typical RR 0.42, 95 % CI 0.34–0.52; typical ARD −0.17, 95 % CI −0.21 to −0.13), and (c) pulmonary interstitial emphysema (typical RR 0.45, 95 % CI 0.37–0.55; typical ARD −0.20, 95 % CI −0.25 to −0.15). There was also a significant decrease in the risk of (a) neonatal mortality (typical RR 0.68, 95 % CI 0.57–0.82; typical ARD −0.09, 95 % CI −0.13 to −0.05), (b) mortality prior to hospital discharge (typical RR 0.63, 95 % CI 0.44–0.90; typical ARD −0.10, 95 % CI −0.18 to −0.03), and (c) bronchopulmonary dysplasia (BPD) or death at 28 days of age (typical RR 0.83, 95 % CI 0.77–0.90; typical ARD −0.11, 95 CI −0.16 to −0.06).
Rescue Therapy with Protein-Free Synthetic Surfactant: In a similar systematic review and meta-analysis of six randomized trials of protein-free synthetic surfactant treatment of established RDS (Soll 1998), surfactant therapy improved pulmonary gas exchange and decreased the requirement for ventilatory support. It also decreased the risk of (a) pneumothorax (typical RR 0.64, 95 % CI 0.55–0.76; typical ARD −0.09, 95 % CI −0.12 to −0.06), (b) pulmonary interstitial emphysema (typical RR 0.62, 95 % CI 0.54–0.71; typical ARD −0.12, 95 % CI −0.16 to −0.09), (c) patent ductus arteriosus (typical RR 0.90, 95 % CI 0.84–0.97; typical ARD −0.06, 95 % CI −0.10 to −0.02), (d) intraventricular hemorrhage (typical RR 0.88, 95 % CI 0.77–0.99; typical ARD −0.04, 95 % CI −0.08 to −0.00), (e) bronchopulmonary dysplasia (typical RR 0.75, 95 % CI 0.61–0.92; typical ARD −0.04, 95 % CI −0.06 to −0.01), (f) neonatal mortality (typical RR 0.73, 95 % CI 0.61–0.88; typical ARD −0.05, 95 % CI −0.07 to −0.02), (g) bronchopulmonary dysplasia or death at 28 days (typical RR 0.73, 95 % CI 0.65–0.83; typical ARD −0.06, 95 % CI −0.11 to −0.05), (h) mortality prior to hospital discharge (typical RR 0.79, 95 % CI 0.68–0.92; typical ARD −0.05, 95 % CI −0.07 to −0.02), and (i) mortality during the first year of life (typical RR 0.80, 95 % CI 0.69–0.94; typical ARD −0.04, 95 % CI −0.07 to −0.01). Treatment with synthetic surfactant increased the risk of apnea of prematurity (typical RR 1.20, 95 % CI 1.09–1.31; typical ARD 0.08, 95 % CI 0.04–0.12).
28.1.3.3.2 Efficacy of Surfactant Therapy in Infants at Risk for RDS (Prophylactic Surfactant)
Several of the early trials of surfactant therapy also studied the effects of prophylactic surfactant in preterm infants at risk for developing RDS (i.e., before they had overt clinical features of RDS). Some of these studies used animal-derived surfactant and others used protein-free synthetic surfactant.
Prophylaxis with Animal-Derived Surfactant: A systematic review and meta-analysis of eight randomized trials (Soll and Özek 1997) found that prophylaxis with animal-derived surfactant lead to an initial improvement in respiratory status and a decrease in the risk of respiratory distress syndrome in infants. It also lead to a decrease in the risk of (a) pneumothorax (typical RR 0.35, 95 % CI 0.26–0.49; typical ARD −0.15, 95 % CI −0.20 to −0.11), (b) pulmonary interstitial emphysema (typical RR 0.46, 95 % CI 0.35–0.60; typical ARD −0.19, 95 % CI −0.25 to −0.13), (c) neonatal mortality (typical RR 0.60, 95 % CI 0.44–0.83; typical ARD −0.07, 95 % CI −0.12 to −0.03), and (d) bronchopulmonary dysplasia or death (typical RR 0.84, 95 % CI 0.75–0.93; typical ARD −0.10, 95 % CI −0.16 to −0.04).
Prophylaxis with Protein-Free Synthetic Surfactant: In a similar systematic review and meta-analysis of seven randomized trials (Soll and Özek 2010), protein-free synthetic surfactant prophylaxis leads to a variable improvement in the respiratory status and a decrease in respiratory distress syndrome in infants who receive prophylactic protein-free synthetic surfactant. It also leads to a decrease in the risk of (a) pneumothorax (typical RR 0.67, 95 % CI 0.50–0.90), (b) pulmonary interstitial emphysema (typical RR 0.68, 95 % CI 0.50–0.93), and (c) neonatal mortality (typical RR 0.70, 95 % CI 0.58–0.85). However, prophylactic protein-free synthetic surfactant administration was associated with an increase in the risk of patent ductus arteriosus (typical RR 1.11, 95 % CI 1.00–1.22) and an increase in the risk of pulmonary hemorrhage (typical RR 3.28, 95 % CI 1.50–7.16).
28.1.3.3.3 Prophylactic Versus Rescue Surfactant Therapy
Many investigators believed that prophylactic administration of surfactant would be the most effective way to deliver surfactant based on the observation in animal studies that surfactant is distributed more uniformly and homogenously when it is administered into a fluid-filled lung (Jobe et al. 1984; Seidner et al. 1995) and the belief that administering surfactant into a previously unventilated or minimally ventilated lung will diminish acute lung injury. In animal models, even brief (15–30 min) periods of mechanical ventilation prior to surfactant administration have been shown to cause acute lung injury resulting in alveolar–capillary damage, leakage of proteinaceous fluid into the alveolar space, and release of inflammatory mediators (Ikegami et al. 1998; Jobe and Ikegami 1998a, b) and to decrease the subsequent response to surfactant replacement (Bjorklund et al. 1997; Rider et al. 1992). Surfactant-deficient animals who receive assisted ventilation develop necrosis and desquamation of the bronchiolar epithelium as early as 5 min after onset of ventilation (Nilsson et al. 1980).
Shortly after surfactant was approved for clinical use, eight randomized controlled trials compared the effects of prophylactic surfactant administration to surfactant treatment of established RDS (Bevilacqua et al. 1996, 1997; Dunn et al. 1991; Egberts et al. 1993; Kattwinkel et al. 1993; Kendig et al. 1991; Merritt et al. 1991; Walti et al. 1995). All these trials used animal-derived surfactant preparations. Trials varied whether surfactant was given before or after the onset of air breathing (pre- or post-ventilatory administration), but all administered surfactant before 15 min of age. The average time of administration of surfactant in the selective treatment groups ranged from 1.5 to 7.4 h. The results of the meta-analysis of the eight trials from a systematic review (Soll and Morley 2012) are summarized in Fig. 28.2.
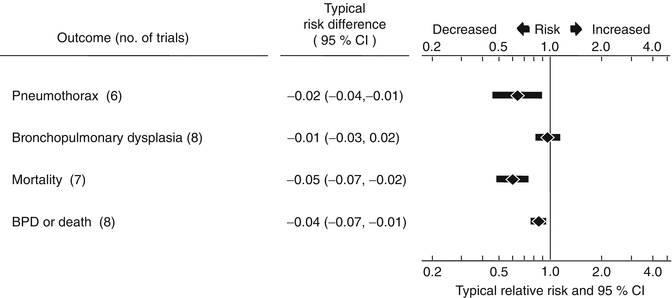
Fig. 28.2
Meta-analysis of eight randomized controlled trials comparing surfactant prophylaxis and treatment (Soll 2001)
Compared to surfactant treatment of established RDS, prophylactic administration of surfactant resulted in a decrease in the risk of pneumothorax (typical RR 0.62, 95 % CI 0.42–0.89; typical ARD −0.02, 95 % CI −0.04 to −0.01), a decrease in the risk of pulmonary interstitial emphysema (typical RR 0.54, 95 % CI 0.36–0.82; typical ARD −0.03, 95 % CI −0.04 to −0.01), a reduction in the risk of neonatal mortality (typical RR 0.61, 95 % CI 0.48–0.77; typical ARD −0.05, 95 % CI −0.07 to −0.02), and a trend towards a decrease in the risk of intraventricular hemorrhage (typical RR 0.92, 95 % CI 0.82–1.03; typical ARD −0.03, 95 % CI −0.06 to 0.01). Because of the greater risk of respiratory distress syndrome and mortality with decreasing gestational age, the benefits of prophylactic administration compared to selective administration were of greater magnitude. The meta-analysis demonstrates that compared to selective administration, prophylactic administration of animal-derived surfactant to infants less than 30 weeks gestation resulted in a greater reduction in neonatal mortality (typical RR 0.62, 95 % CI 0.49–0.78; typical ARD −0.06, 95 % CI −0.09 to −0.03) and a reduction in the combined outcome of bronchopulmonary dysplasia or death (typical RR 0.87, 95 % CI 0.77–0.97; typical ARD −0.05, 95 % CI −0.09 to −0.01).
Preventilatory Versus Post-ventilatory Prophylactic Surfactant Administration: The initial studies using prophylactic surfactant administered the drug as an immediate bolus after intubating the infants rapidly after birth (i.e., “before the first breath”). This approach delays the initiation of neonatal resuscitation, including positive pressure ventilation, and is associated with a risk for surfactant delivery into the right main stem bronchus or esophagus. A randomized trial demonstrated that prophylaxis may be administered in small aliquots soon after resuscitation and confirmation of endotracheal tube position, with equivalent or greater efficacy (Kendig et al. 1998). Based on this trial, prophylactic surfactant should be administered after initial resuscitation of the infant at birth and administration prior to the “first breath” is unnecessary.
The trials mentioned above comparing the use of prophylactic surfactant to surfactant treatment of established RDS were all performed in an era when the use of maternal antenatal glucocorticoids was not as high as in the current era, where 90 % or more of mothers who deliver prematurely receive antenatal glucocorticoids. Also, in these trials, surfactant in the “rescue” group was given relatively late, between 1.5 and 7.4 h, after birth. There are no trials comparing the effects of prophylactic intubation and surfactant administration shortly after birth to infants at high risk of RDS (with intubation primarily performed to administer surfactant) to very early selective administration (e.g., at 30–60 min of life) in intubated infants with early RDS or respiratory insufficiency. Therefore, in current practice, the benefits of prophylactic surfactant demonstrated in these trials might not be as large. A strategy of surfactant prophylaxis subjects infants without RDS to unnecessary intubation and surfactant administration and also to the risk of ventilator-induced lung injury (Clark et al. 2001) (although admittedly some of these infants without RDS may still require intubation and ventilation for respiratory failure not due to RDS). In recent years, prophylactic surfactant administration to preterm infants at risk of RDS has been compared with the use of nasal CPAP (and attempting to avoid mechanical ventilation) as the primary method of respiratory management at birth. These studies are discussed in the next section.
28.1.3.3.4 Initial Respiratory Management of Preterm Infants: CPAP Compared to Intubation Followed by Surfactant Administration
Four large multicenter trials have evaluated the use of prophylactic or early surfactant administration to immediate stabilization on continuous distending pressure (SUPPORT Study Group of the Eunice Kennedy Shriver NICHD Neonatal Research Network 2010; Vermont Oxford Network DRM Study Group et al. 2010; Sandri et al. 2010; Morley et al. 2008).
In the SUPPORT trial (SUPPORT Study Group of the Eunice Kennedy Shriver NICHD Neonatal Research Network 2010), infants 24–27 weeks gestation were randomly assigned to intubation and surfactant treatment (within 1 h after birth) or to CPAP treatment initiated in the delivery room, with subsequent use of a “protocol-driven limited ventilation strategy.” The primary outcome, death, or bronchopulmonary dysplasia (defined as supplemental oxygen requirement at 36 weeks post-menstrual age) did not differ significantly between the CPAP group and the surfactant group (47.8 and 51.0 %, respectively; RR with CPAP, 0.95; 95 % CI, 0.85–1.05) after adjustment for gestational age, center, and familial clustering. The results were similar when bronchopulmonary dysplasia was defined according to the need for any supplemental oxygen at 36 weeks (rates of primary outcome, 48.7 and 54.1 %, respectively; RR with CPAP, 0.91; 95 % CI, 0.83–1.01). Infants who received CPAP treatment, as compared with infants who received surfactant treatment, less frequently required intubation or postnatal corticosteroids for bronchopulmonary dysplasia (P < 0.001), required fewer days of mechanical ventilation (P = 0.03), and were more likely to be alive and free from the need for mechanical ventilation by day 7 (P = 0.01). In secondary analyses, among infants 24 and 25 weeks gestation, there was a significant reduction in the risk of death in the CPAP group, as compared with the early-intubation group. The rate of death during hospitalization was 24 % versus 32 %, RR with CPAP, 0.74; 95 % CI 0.57–0.98. The rate of death at 36 weeks was 20 % versus 29 %, RR, 0.68; 95 % CI 0.5–0.92.
In the Delivery Room Management randomized trial conducted by the Vermont Oxford Network (Vermont Oxford Network DRM Study Group et al. 2010), three strategies were compared in infants at risk of RDS (preterm infants 26–29 weeks): prophylactic surfactant followed by a period of assisted ventilation, intubation with immediate surfactant treatment and rapid extubation to nasal CPAP (ISX), and early stabilization on nasal CPAP (NCPAP). The study was terminated prior to reaching the desired sample size. There were over 200 infants in each of the three study arms. No statistically significant differences were found between the three arms of the trial in the primary outcome of death or chronic lung disease (defined as supplemental oxygen requirement at 36 weeks post-menstrual age). The incidence of the primary outcome was 37 % in the prophylactic surfactant group, 29 % in the ISX group (RR with ISX compared to prophylactic surfactant 0.78, 95 % CI 0.59–1.03), and 31 % in the CPAP group (RR with CPAP compared to prophylactic surfactant 0.83, 95 % CI 0.64–1.09).
In the CURPAP trial (Sandri et al. 2010), infants 25–28 weeks gestation who were not intubated at birth were randomly assigned to prophylactic surfactant or nasal CPAP within 30 min of birth. There were no statistically significant differences between the two groups in the outcomes studied – need for mechanical ventilation in the first 5 days of life, death at 28 days of life, death at 36 weeks post-menstrual age, and the main morbidities of prematurity.
In the COIN trial (Morley et al. 2008), infants 25–28 weeks gestation who were breathing spontaneously but had developed respiratory distress were randomly assigned to CPAP or intubation and ventilation at 5 min after birth. Infants intubated before randomization and those not requiring respiratory support or oxygen were excluded. The primary outcome of death or chronic lung disease (defined as the need for oxygen treatment at 36 weeks gestational age) was not statistically different in infants assigned to receive intubation (39 %) compared with infants assigned to receive CPAP (34 %, odds ratio favoring CPAP, 0.80; 95 % CI 0.58–1.12). At 28 days, there was a lower risk of death or need for oxygen therapy in the CPAP group than in the intubation group (odds ratio, 0.63; 95 % CI 0.46–0.88; P = 0.006). There was little difference in overall mortality. In the CPAP group, 46 % of infants were intubated during the first 5 days, and the use of surfactant was halved. However, the incidence of pneumothorax was 9 % in the CPAP group, as compared with 3 % in the intubation group (P < 0.001). There were no other serious adverse events. The CPAP group had fewer days of ventilation.
The results of these trials suggest that in preterm infants at high risk of RDS, instead of routinely intubating and administering prophylactic surfactant immediately after birth, it is reasonable to try and initially stabilize such infants on nasal CPAP. This approach can avoid unnecessary intubation, unnecessary surfactant administration, and minimize ventilator-induced lung injury, while reducing healthcare costs and resource utilization. However, clinicians should closely monitor infants initially stabilized on CPAP to identify infants who have progressive respiratory failure, so that infants who require surfactant can be intubated and given surfactant as early as possible. Clinicians should also use information available prior to the birth of the infant to identify infants who are not likely to do well with initial stabilization on nasal CPAP, such as infants born to mothers who have not received antenatal glucocorticoids.
28.1.3.3.5 The Preterm Infant with Respiratory Distress Syndrome Who Is Not on Mechanical Ventilation
When a preterm infant has respiratory distress syndrome that is initially managed with CPAP or with supplemental oxygen through a hood, is it better to intubate him early, administer surfactant and extubate within an hour after brief mechanical ventilation, or to wait and see if the infant develops significant respiratory insufficiency, and if he does, only then intubate, give surfactant, and wean him off the ventilator gradually? Six randomized controlled clinical trials addressed this question and are summarized in a systematic review (Stevens Timothy et al. 2007). The rapid (within one hour) extubation attempted in these trials contrasts with the traditional approach – developed when surfactant therapy was first used – of keeping an infant on mechanical ventilation after surfactant administration, weaning ventilator support gradually as the pulmonary status improved, and extubating the infant from low ventilator settings. This approach of rapid extubation followed immediately by the use of nasal CPAP has been called “INSURE” (INtubate, SURfactant, Extubate to CPAP) and is intended to prevent ventilator-induced lung injury than can result from even brief periods of mechanical ventilation (Donn and Sinha 2006; Schmolzer et al. 2008). The six randomized trials, all of which are trials of “rescue” surfactant administration, are summarized in a systematic review (Stevens Timothy et al. 2007). Most of these studies included infants with a gestation of 35 weeks and below and a birth weight of 2,500 g and below. Many of the infants in these studies were between 32 and 35 weeks (few were extremely premature). In these studies of infants with signs and symptoms of RDS, intubation and early surfactant therapy followed by extubation to nasal CPAP (NCPAP) compared with later selective surfactant administration was associated with a lower incidence of mechanical ventilation (typical RR 0.67, 95 % CI 0.57–0.79; typical ARD −0.19, 95 % CI −0.26 to −0.11), air leak syndromes (typical RR 0.52, 95 % CI 0.28–0.96; typical ARD −0.04, 95 % CI −0.08 to 0.00), and BPD (typical RR 0.51, 95 % CI 0.26–0.99; typical ARD −0.08, 95 % CI −0.15 to −0.01). A larger proportion of infants in the early surfactant group received surfactant than in the selective surfactant group (typical RR 1.62, 95 % CI 1.41–1.86; typical ARD 0.38, 95 % CI 0.30–0.47). The number of surfactant doses per patient was significantly greater among patients randomized to the early surfactant group (WMD 0.57 doses per patient, 95 % CI 0.44–0.69). In stratified analysis by FiO2 at study entry, a lower threshold for treatment (FiO2 ≤ 0.45) resulted in lower incidence of air leak (typical RR 0.46 and 95 % CI 0.23–0.93; typical ARD −0.05, 95 % CI −0.10 to −0.01) and BPD (typical RR 0.43, 95 % CI 0.20–0.92; typical ARD −0.10, 95 % CI −0.19 to −0.02). A higher treatment threshold (FiO2 > 0.45) at study entry was associated with a higher incidence of patent ductus arteriosus requiring treatment (typical RR 2.15, 95 % CI 1.09–4.13; typical ARD 0.12, 95 % CI 0.02–0.21). In another recent randomized trial (Rojas et al. 2009), infants 27–31 weeks gestation with RDS who were randomly assigned within the first hour of life either to intubation, very early surfactant, extubation, and nasal continuous positive airway pressure required less ventilation and had a lower incidence of mortality and air leaks (pneumothorax and pulmonary interstitial emphysema) than infants assigned to nasal continuous airway pressure alone. These data suggest that for a preterm infant with RDS who is not on mechanical ventilation (and is being managed on CPAP or an oxygen hood), early intubation at a low FiO2 threshold (FiO2 < 0.45), surfactant administration, and rapid extubation to CPAP decreases the risk of needing mechanical ventilation, BPD, and air leak syndrome, although it results in more surfactant use. Whether the same INSURE approach should be followed when prophylactic surfactant therapy is used is not clear due to lack of evidence.
28.1.3.3.6 Early Versus Late Treatment of Established RDS
Preterm infants who do not receive prophylaxis and subsequently develop RDS should be treated with surfactant as soon as possible. This strategy is supported by many of the same arguments that support prophylactic surfactant administration as well as by clinical trials. Four randomized controlled trials (European Exosurf Study Group 1992; Gortner et al. 1998; Konishi et al. 1992; The OSIRIS Collaborative Group 1992), including the largest randomized trial conducted in neonatology (the OSIRIS trial), have evaluated early versus delayed selective surfactant administration. The results of these trials are summarized in a systematic review (Yost and Soll 2000). In these trials, early administration of surfactant consisted of administration of the first dose within the first 30 min to the first 2 h of life. Two of these studies used animal-derived surfactants and two used protein-free synthetic surfactant. The results of the meta-analysis of these studies are summarized in Fig. 28.3.
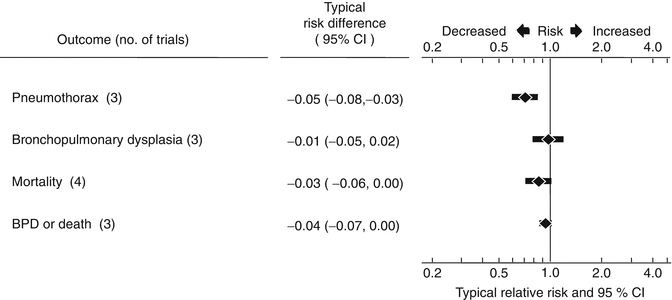
Fig. 28.3
Meta-analysis of four randomized controlled trials comparing early and delayed surfactant treatment (Yost and Soll 2000)
Early selective treatment resulted in a decrease in the risk of pneumothorax (typical RR 0.70, 95 % CI 0.59–0.82; typical ARD −0.05, 95 % CI −0.08 to −0.03), a decrease in the risk of pulmonary interstitial emphysema (typical RR 0.63, 95 % CI 0.43–0.93; typical ARD −0.06, 95 % CI −0.10 to −0.01), a decrease in the risk of chronic lung disease (requirement for supplemental oxygen at 36 weeks gestation, typical RR 0.70, 95 % CI 0.55–0.88; typical ARD −0.03, 95 % CI −0.05 to −0.01) and a decrease in the risk of neonatal mortality (typical RR 0.87, 95 % CI 0.77–0.99; typical ARD −0.03, 95 % CI −0.06 to 0.00). Therefore preterm infants who do not receive prophylactic surfactant and subsequently develop clinical features of RDS should receive the first dose of surfactant as early as possible. Outborn infants are at highest risk of delayed administration. Tertiary referral units accepting outborn infants should attempt to develop systems to ensure that surfactant is administered as early as possible to these infants, either by the transporting team or, if appropriate, by the referring hospital. In inborn infants, delays in administration of surfactant occur if other admission procedures such as line placement, radiographs, and nursing procedures are allowed to take precedence over surfactant dosing soon after birth. Surfactant administration should be given priority over such admission procedures.
28.1.3.3.7 Single Versus Multiple Surfactant Doses
Many of the initial trials of surfactant therapy tested a single dose of surfactant. However, surfactant may become rapidly metabolized and functional inactivation of surfactant can result from the action of soluble proteins and other factors in the small airways and alveoli (Jobe and Ikegami 1993). Administering repeat doses of surfactant can overcome such inactivation. The results of two randomized controlled trials that compared multiple dosing regimens to single-dose regimens of animal-derived surfactant extract for treatment of established respiratory distress syndrome (Dunn et al. 1990; Speer et al. 1992) have been evaluated in a systematic review (Soll and Özek 2009). In one study (Dunn et al. 1990), after the initial dose of bovine lipid extract surfactant, infants assigned to the multiple-dose group could receive up to three additional doses during the first 72 h of life if they had a respiratory deterioration, provided they had shown a positive response to the first dose and a pneumothorax had been eliminated as the cause of the respiratory deterioration. In the other study (Speer et al. 1992), infants in the multiple-dose group received additional doses of poractant at 12 and 24 h after the initial dose if they still needed supplemental oxygen and mechanical ventilation. Approximately 70 % of the infants randomized to the multiple-dose regimen received multiple doses.
The meta-analysis supports a decreased risk of pneumothorax associated with multiple dose surfactant therapy (typical RR 0.51, 95 % CI 0.30–0.88; typical ARD −0.09, 95 % CI −0.15 to −0.02). There was also a trend towards decreased mortality (typical RR 0.63, 95 % CI 0.39–1.02; typical ARD −0.07, 95 % CI −0.14 to 0.00). No differences were detected in other clinical outcomes. No complications associated with multiple-dose treatment were reported in these trials. In a third study, in which protein-free synthetic surfactant was used in a prophylactic manner, the use of two doses of surfactant in addition to a prophylactic dose lead to a decrease in mortality, respiratory support, necrotizing enterocolitis, and other outcomes when compared to a single prophylactic dose (Corbet et al. 1995). In the OSIRIS trial, which used protein-free synthetic surfactant, a two-dose treatment schedule was found to be equivalent to a treatment schedule permitting up to four doses of surfactant.
28.1.3.3.8 Threshold for Administration of Repeat Doses of Surfactant
The use of a higher threshold for retreatment with surfactant appears to be as effective as a low threshold and can lead to significant savings in costs of the drug. The criteria for administration of repeat doses of surfactant were investigated in two studies that both used animal-derived surfactant. In one study (Dunn et al. 1991), the retreatment criteria compared were an increase in the fraction of inspired oxygen by 0.1 over the lowest baseline value (standard retreatment) versus a sustained increase of just 0.01 (liberal retreatment). There were no differences in complications of prematurity or duration of respiratory support. However, short-term benefits in oxygen requirement and degree of ventilator support were noted in the liberal retreatment group.
In another study (Kattwinkel et al. 2000), retreatment at a low threshold (FiO2 > 30 %, still requiring endotracheal intubation) was compared to retreatment at a high threshold (FiO2 > 40 %, mean airway pressure >7 cm H2O). Again, there were minor short-term benefits to using a low threshold with no differences in major clinical outcomes. However, in a subgroup of infants with respiratory distress syndrome complicated by perinatal compromise or infection, infants in the high-threshold group had a trend towards higher mortality than the low-threshold group. Based on current evidence, it appears appropriate to use persistent or worsening signs of respiratory distress syndrome as criteria for retreatment with surfactant. A low threshold for repeat dosing should be used for infants with RDS who have perinatal depression or infection.
28.1.3.3.9 Comparisons Between Surfactant Products
Comparison of Animal-Derived and Protein-Free Synthetic Surfactants: Although both protein-free synthetic and animal-derived surfactants are effective, their composition differs. Animal-derived surfactant extracts contain surfactant-specific proteins that aid in surfactant adsorption and resist surfactant inactivation (Kuroki and Voelker 1994; Possmayer 1990). Eleven randomized trials have compared the effects of animal-derived and protein-free synthetic surfactants in the treatment or prevention of RDS (Ainsworth et al. 2000; Alvarado et al. 1993; da Costa et al. 1999; Horbar et al. 1993b; Hudak et al. 1996, 1997; Kukkonen et al. 2000; Modanlou et al. 1997; Pearlman et al. 1993; Sehgal et al. 1994; Neonatal 1996). A total of over 4,500 infants were studied in these trials. A systematic review of these trials is available (Soll and Blanco 2001). The results of the meta-analysis are summarized in Fig. 28.4.
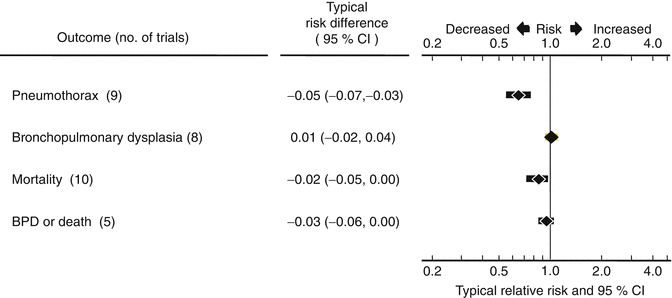
Fig. 28.4
Meta-analysis of 11 randomized controlled trials comparing animal-derived and synthetic surfactants (Soll 2001)
Compared to protein-free synthetic surfactant, treatment with animal-derived surfactant extracts resulted in a significant reduction in the risk of pneumothorax (typical RR 0.63, 95 % CI 0.53–0.75; typical ARD −0.04, 95 % CI −0.06 to −0.03) and the risk of mortality (typical RR 0.87, 95 % CI 0.76–0.98; typical ARD −0.02, 95 % CI −0.05 to 0.00). Natural surfactant extract is associated with a marginal increase in the risk of intraventricular hemorrhage (typical RR 1.09, 95 % CI 1.00–1.19; typical ARD 0.03, 95 % CI 0.00–0.06), but no increase in grade 3 to 4 intraventricular hemorrhage (typical RR 1.08, 95 % CI 0.92–1.28; typical ARD 0.01, 95 % CI −0.01 to 0.03). The meta-analysis also supports a marginal decrease in the risk of bronchopulmonary dysplasia or mortality associated with the use of natural surfactant preparations (typical RR 0.95, 95 % CI 0.90–1.01; typical ARD −0.03, 95 % CI −0.06 to 0.00).
In addition to these benefits, animal-derived surfactants have a more rapid onset of action, allowing ventilator settings and inspired oxygen concentrations to be lowered more quickly than with protein-free synthetic surfactant (Horbar et al. 1993b; Hudak et al. 1996; Modanlou et al. 1997; Rollins et al. 1993; Choukroun et al. 1994). A comparison of physical properties and the results of animal studies also suggest that animal-derived surfactants have advantages over protein-free synthetic surfactants (Halliday 1996). These properties are attributed to the presence of the surfactant proteins SP-B and SP-C in animal-derived surfactants (Hall et al. 1992a).
The use of animal-derived surfactant preparations should be favored in most clinical situations, as their use results in greater clinical benefits than protein-free synthetic surfactants. However, all animal-derived surfactants have to be refrigerated for storage. The protein-free synthetic surfactant colfosceril is available as a lyophilized powder that is to be stored at below 30 °C in a dry place (not to be frozen) and reconstituted with sterile water before use. Therefore in situations where refrigeration is a problem (as in developing countries), it may be more practical to use colfosceril than animal-derived surfactants.
Comparison of Animal-Derived and Protein-Containing Synthetic Surfactants: Clinical trials have compared the effects of synthetic surfactants containing peptides to animal-derived surfactant preparations. These synthetic surfactants do not have the theoretical concerns associated with animal-derived surfactants, namely, transmission of microorganisms, exposure to animal proteins and inflammatory mediators, susceptibility to inactivation, and inconsistent content (Engle and Committee on Fetus and Newborn 2008). Lucinactant, the synthetic surfactant containing an analog of SP-B, sinapultide, was compared with beractant in SELECT, a multicenter masked randomized trial of surfactant prophylaxis in infants 24–32 weeks gestation (Moya et al. 2005). Lucinactant was also compared with poractant in STAR, a multicenter randomized trial of surfactant prophylaxis in infants 24–28 weeks gestation that was structured as a non-inferiority trial (Sinha et al. 2005). A meta-analysis of these two studies (Pfister et al. 2007) found no significant differences in outcomes between lucinactant and the comparison animal-derived surfactant in mortality at 36 weeks post-menstrual age (typical RR 0.81, 95 % CI 0.64–1.03), chronic lung disease at 36 weeks post-menstrual age (typical RR 0.99, 95 % CI 0.84–1.18), the composite outcome of mortality or chronic lung disease at 36 weeks post-menstrual age (typical RR 0.96, 95 % CI 0.82–1.12), or in other respiratory outcomes. A decreased risk of necrotizing enterocolitis, a secondary outcome, was noted in infants receiving lucinactant (typical RR 0.60, 95 % CI 0.42–0.86; typical RD −0.06, 95 % CI −0.10 to −0.01).
However, both trials of lucinactant described above had multiple methodologic problems (Kattwinkel 2005) that undermine their validity, and at present there is no clear evidence of the equivalence or superiority of lucinactant over any animal-derived surfactant product (Halliday 2006). While these newer surfactants show promise, further research is required to elucidate their role in the prevention or treatment of RDS.
Comparison of Protein-Containing Versus Protein-Free Synthetic Surfactants: In the SELECT trial (Moya et al. 2005; Pfister Robert et al. 2009), the randomized trial of lucinactant mentioned above where it was compared with beractant, it was also compared to colfosceril. Compared to infants receiving colfosceril, infants receiving lucinactant had less RDS (39 % vs 47 %) and less RDS-related mortality (4.7 % vs 9.4 %, RR 0.50, 95 % CI 0.32–0.80). All-cause mortality at 36 weeks post-menstrual age was not significantly different (21 % for lucinactant vs 24 % for colfosceril). A trend towards a reduction in BPD or death at 36weeks post-menstrual age was associated with lucinactant treatment when compared to colfosceril (RR 0.88, 95 % CI 0.77–1.01).
Comparison of Different Types of Bovine Surfactants: Two randomized trials, both from the same group of investigators, have compared the efficacy and adverse effects of different bovine surfactant products. In a comparison of beractant (Survanta) and calf lung surfactant extract (Infasurf) (Bloom et al. 1997), there were no differences detected between the two groups in the frequency of air leaks, complications associated with dosing, complications of prematurity, mortality, or survival without chronic lung disease. However, some differences were noted among subgroups of infants. Among infants treated for established respiratory distress syndrome, those who received calf lung surfactant extract had a significantly longer interval between doses, a lower inspired oxygen concentration, and a lower mean airway pressure in the first 48 h of life than infants treated with beractant. Among infants in whom these surfactants were administered in a preventive manner, mortality in infants with a birth weight <600 g was significantly higher with calf lung surfactant extract than with beractant. In a second report (Bloom et al. 2005) that included two separate trials – a prophylaxis trial and a treatment trial – the trials were halted prematurely due to recruitment problems and hence had inconclusive results with no demonstrated differences in outcomes between the two products. Thus, there is no evidence of the superiority of one bovine preparation over the other.
Comparison of Porcine and Bovine Surfactants: Five studies comparing surfactant treatment of established moderate to severe RDS with poractant versus beractant have been published (Baroutis et al. 2003; Speer et al. 1995; Malloy et al. 2005; Halahakoon 1999; Ramanathan et al. 2004).
A meta-analysis of these studies (Halliday 2005) found that compared to beractant, poractant treatment led to a significant reduction in neonatal mortality (typical RR 0.57, 95 % CI 0.34–0.96). The dose of beractant was uniformly 100 mg/kg across all five studies. When only studies that used a 100 mg/kg dose of poractant were considered, the reduction in mortality was not statistically significant (typical RR 0.82, 95 % CI 0.44–1.58), emphasizing the fact that the most significant effect on mortality was seen with a 200 mg/kg dose of poractant (typical RR 0.29, 95 % CI 0.10–0.79). Two of these five studies (Speer et al. 1995; Ramanathan et al. 2004) also reported more rapid improvement in oxygenation with poractant compared to beractant. The difference in outcomes described above between poractant and beractant may well be related to the dose of phospholipids and not to other characteristics of the products. There are no studies to determine whether poractant, especially in a 100 mg/kg dose, is superior to beractant when surfactant is dosed for prophylaxis.
28.1.3.3.10 Factors Affecting the Response to Surfactant Therapy
Several factors have been reported by various authors to be associated with a poor response to surfactant therapy, either in terms of immediate pulmonary response or in terms of later morbidity and mortality. These factors include high total fluid and colloid intake in the first days of life (Hallman et al. 1993), a low mean airway pressure relative to the FiO2 (Hallman et al. 1993), the presence of an additional pulmonary disorder such as infection (Segerer et al. 1991) and perinatal asphyxia, other complications of prematurity (Konishi et al. 1992), high fraction of inspired oxygen requirement at entry (had a negative impact on a/APO2 6 and 24 h after treatment), lower birth weight, male sex, outborn status, and high airway pressure requirement at entry (Collaborative European Multicentre Study Group 1991). Low birth weight, low Apgar score, and initial disease severity were associated with an increased mortality (Herting et al. 1992).
A high pulmonary resistance prior to therapy was associated with a poor response to therapy at 24 and 48 h (Wallenbrock et al. 1992). In addition, the immediate response to surfactant therapy itself has been reported to be a significant prognostic indicator for mortality and morbidity (Kuint et al. 1994). In animal studies, poor response to surfactant has been associated with delayed administration (Seidner et al. 1995) and the leakage of proteinaceous fluid into the alveolar spaces. Within some multicenter trials, significant differences in outcomes of surfactant-treated infants have been noted between participating hospitals (Collaborative European Multicentre Study Group 1991; Herting et al. 1992), suggesting that variations in patient care practices have an important influence on the outcomes of surfactant-treated infants.
As noted earlier, observational studies have demonstrated a decrease in mortality and morbidity for such infants after the introduction of surfactant therapy. However, racial differences in this decline in mortality have been reported. In one study, the overall neonatal mortality for black very low birth weight infants did not change after the introduction of surfactant therapy (Hamvas et al. 1996), and in another study, declines in neonatal mortality risks caused by respiratory distress syndrome and all respiratory causes were greater for non-Hispanic white VLBW infants than for black VLBW infants (Ranganathan et al. 2000). While such racial differences have been noted at a population level, the role of racial factors in the response pattern of individual infants with respiratory distress syndrome to exogenous surfactant therapy is unknown.
28.1.3.4 Adverse Effects of Surfactant Therapy
Transient hypoxia and bradycardia can occur due to acute airway obstruction immediately following surfactant instillation (Zola et al. 1993a; Liechty et al. 1991). Other acute adverse effects of surfactant administration include reflux of surfactant into the pharynx from the endotracheal tube, increase in transcutaneous carbon dioxide tension, tachycardia, gagging, and mucous plugging of the endotracheal tube. These complications of surfactant administration generally respond to a slower rate of surfactant administration or to an increased airway pressure or FiO2 during administration. Rapid improvement in oxygenation after surfactant administration necessitates close monitoring and appropriate reduction of ventilatory parameters.
Several authors have reported a transient decrease in blood pressure (Hellstrom-Westas et al. 1992; Skov et al. 1992a, b), a transient decrease in cerebral blood flow velocity (Cowan et al. 1991; Murdoch and Kempley 1998; Edwards et al. 1992), a transient decrease in cerebral oxyhemoglobin concentration (Edwards et al. 1992), and a transient decrease in cerebral activity on amplitude-integrated electroencephalography (Hellstrom-Westas et al. 1992) immediately after surfactant administration. The EEG depression observed after surfactant instillation is not caused by cerebral ischemia (Bell et al. 1994), and the EEG suppression is not directly related to alterations in blood gases or systemic circulation (Lundstrom and Greisen 1996). The clinical significance of these findings is uncertain. One study (Horbar et al. 1990) reported an increase in the incidence of intraventricular hemorrhage and a case report documents a temporal association between the development of intraventricular hemorrhage and the administration of surfactant-TA to improve respiratory failure caused by pulmonary hemorrhage (Funato et al. 1992). However, the meta-analyses of multiple trials do not show an increase in the risk of intraventricular hemorrhage with surfactant therapy compared to placebo (Seger and Soll 2009; Soll 1998; Soll and Özek 1997, 2010).
There is well-described increase in the risk of pulmonary hemorrhage with surfactant therapy (Raju and Langenberg 1993; Tomaszewska et al. 1999). Although trials in which animal-derived surfactants were used reported a higher incidence (5–6 %) of pulmonary hemorrhage than trials of protein-free synthetic surfactant (1–3 %), direct comparison demonstrates no difference in the risk of pulmonary hemorrhage. The overall incidence of pulmonary hemorrhage was low and the absolute magnitude of the increased risk is small (Raju and Langenberg 1993). However, moderate and severe pulmonary hemorrhage is associated with an increased risk of death and short-term morbidity. It is not associated with increased long-term morbidity (Pandit et al. 1999).The occurrence of pulmonary hemorrhage may be related to the presence of a hemodynamically significant patent ductus arteriosus (Garland et al. 1994). Seppanen et al. studied the association of neonatal complications with the Doppler-derived aortopulmonary pressure gradient (APPG) across the ductus arteriosus, which reflects pulmonary artery pressure during the first day of life. Infants in whom the APPG decreased after birth had a lower frequency of patent ductus arteriosus and pulmonary hemorrhage than those whose APPG remained low (Seppanen et al. 1995). Another mechanism for the pulmonary hemorrhage may be a direct cytotoxicity, which has been demonstrated in in vitro studies and appears to be different for different surfactants and different dosages (Findlay et al. 1995).
When surfactant initially became available for clinical testing, there was concern that the introduction of foreign proteins from animal-based lung surfactants into the lungs of preterm infants could lead to immunological responses. Two studies did not find antibodies specific to surfactant protein in the sera of preterm infants treated with bovine surfactant (Bartmann et al. 1992; Whitsett et al. 1991). In other studies, immune complexes or antibodies to the protein in exogenous porcine, bovine, or human surfactant have been identified in the sera of neonates with respiratory distress syndrome. However similar immune complexes or antibodies were also noted in control infants who did not receive surfactant, and no significant differences were noted between surfactant-treated and control infants (Chida et al. 1991; Strayer et al. 1989; Robertson et al. 1992). The presence of antibodies in control infants may be the result of leakage of surfactant proteins into the circulation (Chida et al. 1991).
With animal-derived surfactants, there is a theoretical risk of the transmission of infectious agents, including bovine spongiform encephalitis with surfactants derived from bovine sources and other viral infections in swine. Organic solvent processing of phospholipids, terminal sterilization techniques, and screening of animal sources have been used to minimize this risk.
28.1.3.5 Long-Term Outcomes After Surfactant Therapy
Long-term outcomes after surfactant therapy have been well studied for protein-free synthetic surfactant. Follow-up studies of long-term outcomes after animal-derived surfactant therapy have consisted of small numbers of patients, with a variable proportion of survivors being tested. For both protein-free synthetic and animal-derived surfactant, the “long-term” outcomes reported consist of outcomes predominantly in the first 3 years of life, with very few reports of outcomes at school age or higher. Given these limitations, the evidence suggests that not only do more infants survive from surfactant therapy but they are also at no selective disadvantage for neurodevelopmental sequelae due to the surfactant therapy. Most comparisons of long-term outcomes have been between infants treated with surfactant and placebo. There are few or no comparisons of long-term outcomes between infants treated with different types of surfactant or different regimens of the same surfactant. The following sections mainly address comparisons between infants treated with surfactant and placebo.
28.1.3.5.1 Neurodevelopmental Outcomes
No significant differences have been reported in the long-term neurodevelopmental outcomes of infants treated with surfactant compared to those treated with placebo, either with protein-free synthetic surfactant (Corbet et al. 1995; Morley and Morley 1990; Courtney et al. 1995) or animal-derived surfactant (Hoekstra et al. 1994; Robertson et al. 1992; Dunn et al. 1988; Ferrara et al. 1991; Vaucher et al. 1988; Wagner et al. 1995; Ware et al. 1990).
28.1.3.5.2 Long-Term Respiratory Outcomes
Compared to infants treated with placebo, infants treated with surfactant in the neonatal period have been reported to have either improved (Abbasi et al. 1993; Pelkonen et al. 1998; Yuksel et al. 1993) or equivalent (Couser et al. 1993; Gappa et al. 1999; Walti et al. 1992) results on pulmonary function testing. Some studies have reported a lower frequency of subsequent clinical respiratory disorders in surfactant-treated infants compared to placebo (Vaucher et al. 1988; Sell et al. 1995), while others have reported no difference (Robertson et al. 1992; Morley and Morley 1990; Dunn et al. 1988; Abbasi et al. 1993) or a trend towards an increase in allergic manifestations (Ware et al. 1990).
28.1.3.5.3 Physical Growth
28.1.3.5.4 Outcomes of Prophylactic Versus Rescue Treatment Strategies
Two studies compared the long-term outcomes of infants treated with prophylactic surfactant to those treated with a “rescue” strategy. In one, there were no differences at school age in neurodevelopmental outcome or in the results of pulmonary function testing between the two groups, though infants who had received prophylactic surfactant showed fewer clinical pulmonary problems than those that received rescue treatment (Sinkin et al. 1998). In another study, in which there was significant loss of infants to follow-up (and therefore a high likelihood of attrition bias), the mean scores on the Bayley scales of infant development at 12 months adjusted age were higher in the rescue group than in the prophylactic group (Vaucher et al. 1993).
28.1.3.6 Exogenous Surfactant Therapy for Conditions Other than RDS
28.1.3.6.1 Meconium Aspiration Syndrome
In non-controlled studies of human infants with meconium aspiration syndrome, improved oxygenation has been reported with exogenous surfactant therapy (Auten et al. 1991; Halliday et al. 1996; Khammash et al. 1993). A randomized trial in infants greater than 34 weeks gestation (including infants with MAS) with severe respiratory failure on extracorporeal membrane oxygenation (ECMO) showed that infants treated with beractant had improved lung function, a shorter duration of ECMO, and fewer complications after ECMO (Lotze et al. 1993).
Four randomized trials (Lotze et al. 1998; Findlay et al. 1996; Chinese Collaborative Study Group for Neonatal Respiratory Diseases 2005; Maturana et al. 2005) have studied the effect of animal-derived surfactant in term infants with meconium aspiration syndrome and are included in a systematic review. In these trials, surfactant therapy was administered as a continuous infusion over 20 min (Findlay et al. 1996) or as a bolus. The meta-analysis of these four trials (El Shahed et al. 2007) showed a decreased need for extracorporeal membrane oxygenation with surfactant therapy (typical RR 0.64, 95 % CI 0.46–0.91; typical ARD −0.17, 95 % CI −0.30 to −0.04). One trial reported a reduction in the length of hospital stay (mean difference −8 days (95 % CI −14 to −3 days)). There were no statistically significant effects on mortality (typical RR 0.98 (95 % CI 0.41–2.39), typical ARD 0.00 (95 % CI −0.05 to 0.05)) or other outcomes (duration of assisted ventilation, duration of supplemental oxygen, pneumothorax, pulmonary interstitial emphysema, air leaks, chronic lung disease, need for oxygen at discharge, or intraventricular hemorrhage).
In summary, infants with severe meconium aspiration syndrome are likely to benefit from treatment with animal-derived surfactants. Multiple doses are usually required in such infants. Only animal-derived surfactants have been tested in human clinical trials in this setting. Each dose should be administered cautiously, with close cardiac, respiratory, and oxygen saturation monitoring, because surfactant can aggravate preexisting airway obstruction from meconium and transient oxygen desaturation and endotracheal tube obstruction have been reported with bolus administration in nearly one-third of infants (Lotze et al. 1998).
Investigators have also attempted to treat MAS by lavaging the airways with diluted surfactant solutions in order to wash out residual meconium (Dargaville et al. 2007; Wiswell et al. 2002; Hung et al. 2006; Lista et al. 2006; Gadzinowski et al. 2008). When this approach was tested in a randomized trial with a small number of babies, there were no statistically significant differences in clinical outcomes, although there was a trend towards reduction in the combined outcome of death or need for ECMO (Dargaville et al. 2010).
28.1.3.6.2 Acute Respiratory Distress Syndrome
Surfactant dysfunction is well described in acute lung injury (Willson et al. 2008). Therefore, surfactant replacement has been proposed as a treatment for patients with acute lung injury and the acute respiratory distress syndrome (ARDS), which, although more common in adults and older children, can occur in term neonates (Faix et al. 1989; Pfenninger et al. 1991). Exogenous surfactant therapy has been attempted in ARDS in adults but the results of clinical trials have not been promising (Anzueto et al. 1996; Gregory et al. 1997a). There are no randomized trials of exogenous surfactant therapy specifically for ARDS in neonates, but in older children with acute respiratory failure, surfactant use decreased mortality and duration of ventilation (Willson et al. 1999; Duffett et al. 2007). Because of this, and based on the pathophysiologic, clinical, and radiologic similarities between RDS and ARDS, it is reasonable to provide exogenous surfactant therapy to term infants with clinical and radiologic features of ARDS (severe respiratory failure with pulmonary opacification and air bronchograms on chest radiographs). The use of surfactant in ARDS is discussed in detail in Sect. 28.2.
28.1.3.6.3 Other Conditions
There are reports (single-case reports or case series) of the use of exogenous surfactant therapy in human infants for the management of pulmonary hemorrhage (Pandit et al. 1995; Amizuka et al. 2003) and neonatal pneumonia (Auten et al. 1991; Robertson 1996; Herting et al. 2000; Fetter et al. 1995). However, the efficacy of surfactant in these conditions is uncertain and its routine use in these conditions cannot be recommended. Surfactant therapy for infants with congenital diaphragmatic hernia has also been attempted (Bos et al. 1991; Lotze et al. 1994; Glick et al. 1992; Lally et al. 2004; Van Meurs and Congenital Diaphragmatic Hernia Study Group 2004) but actually resulted in worse outcomes and therefore is not recommended.
28.1.4 Future Developments
Future research in neonatal surfactant therapy will likely attempt to understand the functioning and clinical effects of protein-containing synthetic surfactants. Emerging research is also addressing different methods of surfactant delivery to the lungs.
Surfactant administration through a laryngeal mask airway is noninvasive, avoids endotracheal intubation, and has been reported in a series of eight preterm infants with RDS managed with nasal CPAP (Trevisanuto et al. 2005). The mean arterial-to-alveolar oxygen tension ratio improved significantly after the treatment and no complications were reported. This method of administration is promising, as it potentially avoids the complications associated with intubation, but requires testing in a large randomized trial before it can be recommended.
Another noninvasive method of surfactant administration is instillation of surfactant into the nasopharynx during or immediately after delivery and before the first breath. Such instillation is thought to cause the surfactant to be aspirated into the fluid-filled airway as an air–fluid interface is established. A case series (Kattwinkel et al. 2004) of 23 preterm infants 27–30 weeks receiving such intrapartum nasopharyngeal instillation of surfactant followed by placement on CPAP immediately after birth (mask CPAP initially followed by nasal CPAP) demonstrated the feasibility of such administration. However, more evidence is required to prove the efficacy of this approach before it can be used or recommended.
The administration of surfactant to spontaneously breathing infants on nasal CPAP by passing a thin catheter into the trachea has been reported, initially from a single center (Kribs et al. 2008) and subsequently from a nonrandomized multicenter study (Kribs et al. 2010). While this method is promising, it requires rigorous evaluation in randomized trials. It is now being evaluated in a multicenter study called the AMV (Avoid Mechanical Ventilation) trial.
Finally, future research is likely to (or should) address methods of better identifying infants with respiratory disorders who would benefit from surfactant therapy. Current methods of selecting infants for surfactant therapy are primarily demographic (e.g., infants below a certain gestation), clinical (signs of respiratory distress with oxygen requirement), or radiographic (radiographic features of RDS). The development of more sophisticated and highly accurate tests to identify infants who will benefit from surfactant and those in whom surfactant therapy is not required will be very useful to clinicians.
Essentials to Remember
-
Exogenous surfactant therapy is now a standard therapy for respiratory distress syndrome. Numerous randomized controlled trials have proved its efficacy and compared different products and treatment strategies.
-
In infants with RDS, surfactant should be administered as early as possible after its need is identified.
-
Animal-derived surfactant products are superior to protein-free synthetic surfactants. A new class of protein-containing synthetic surfactants is being tested for efficacy and might prove to have an important role in exogenous surfactant therapy in the future.
-
While prophylactic surfactant administration to preterm infants at risk of RDS was previously the preferred practice compared to “rescue” surfactant administration, emerging new evidence suggests that initial stabilization of preterm infants on nasal continuous positive airway pressure might be equally effective, requires fewer resources, and avoids ventilator-induced lung injury.
-
Exogenous surfactant therapy has potential benefits in term infants with meconium aspiration syndrome.
28.2 Exogenous Surfactant in the Pediatric Patient
Douglas Willson10, 11
(10)
Department of Pediatrics, University of Vermont College of Medicine, Burlington, VT 05401, USA
(11)
Fletcher Allen Health Care, University of Vermont College of Medicine, Burlington, VT 05401, USA
Educational Aims
-
To briefly review the history of surfactant therapy in neonates and in older children and adults
-
To review the physiology underlying the potential value of exogenous surfactant therapy in ALI/ARDS
-
To describe the different types of pharmaceutical surfactants
-
To detail the animal and human studies examining the effects of exogenous surfactant in ALI/ARDS
-
To speculate on the future role of exogenous surfactant as a component of therapy for ALI/ARDS
28.2.1 Introduction
With the identification of surfactant deficiency as the putative cause of infantile respiratory distress syndrome (IRDS) by Avery and Mead in 1959 (Avery and Mead 1959) and the closely following recognition of dipalmitoyl phosphatidylcholine (DPPC) as a major surfactant lipid component (Brown 1962, 1964), it was not long before treatment with this compound as a substitute “lung surfactant” was attempted. The studies of both Robillard et al. in 1964 (1964) and Chu et al. in 1967 (1967) tried unsuccessfully to treat infants with established IRDS (called hyaline membrane disease at the time) using aerosolized DPPC. The failure of this initial “surfactant replacement therapy,” together with a small but apparently positive physiological response to pulmonary vasodilatation, led to the erroneous conclusion that IRDS was due to ischemia and that surfactant deficiency was the result and not the cause of this disease (Chu et al. 1965, 1967). This misinterpretation was widely accepted until Enhorning and colleagues (Enhorning et al. 1973a, b) in 1973 demonstrated that whole surfactant isolated from adult rabbits could induce near-normal gas exchange when instilled into the trachea of premature rabbit pups, documenting that their respiratory failure was caused by surfactant deficiency. Moreover, it was not until 1981 that Fujiwara et al. (Fujiwara 1981) reported positive responses in respiratory function in human infants treated with a bovine-derived exogenous lung surfactant. We now understand that pulmonary surfactant is more than just DPPC, and that multiple lipid and peptide components are required for full activity. It is also now appreciated that the attempts of Robillard et al. (1964) and Chu et al. (1967) to aerosolize DPPC were not effective in delivering adequate amounts of material to the alveoli. Intratracheal instillation of exogenous surfactants in aqueous suspension is the current standard of care for the treatment and prevention of IRDS.
The ongoing development of surfactant therapy for clinical acute lung injury (ALI) and the acute (formerly “adult”) respiratory distress syndrome (ARDS) has been subject to some of the same issues that occurred during early attempts at this intervention in premature infants with IRDS. It has been known for some time that endogenous surfactant becomes dysfunctional in many forms of acute inflammatory lung injury, providing a direct conceptual rationale for exogenous surfactant supplementation. Despite this rationale, initial controlled studies attempting to treat adults with ARDS with exogenous surfactants were unsuccessful (Anzueto et al. 1996; Gregory et al. 1997b) and prompted calls for abandoning further clinical trials (Matthay 1996). However, the exogenous surfactants used in these studies (Anzueto et al. 1996; Gregory et al. 1997b) lacked one or more highly active components found in native surfactant, and the patients had sepsis-induced ARDS that involved substantial extrapulmonary pathology. Other studies of exogenous surfactant therapy in patients with ALI/ARDS give reason for cautious optimism about the use of this intervention, particularly in the case of direct pulmonary forms of these syndromes. This chapter briefly reviews the history and rationale for surfactant therapy in ALI/ARDS.
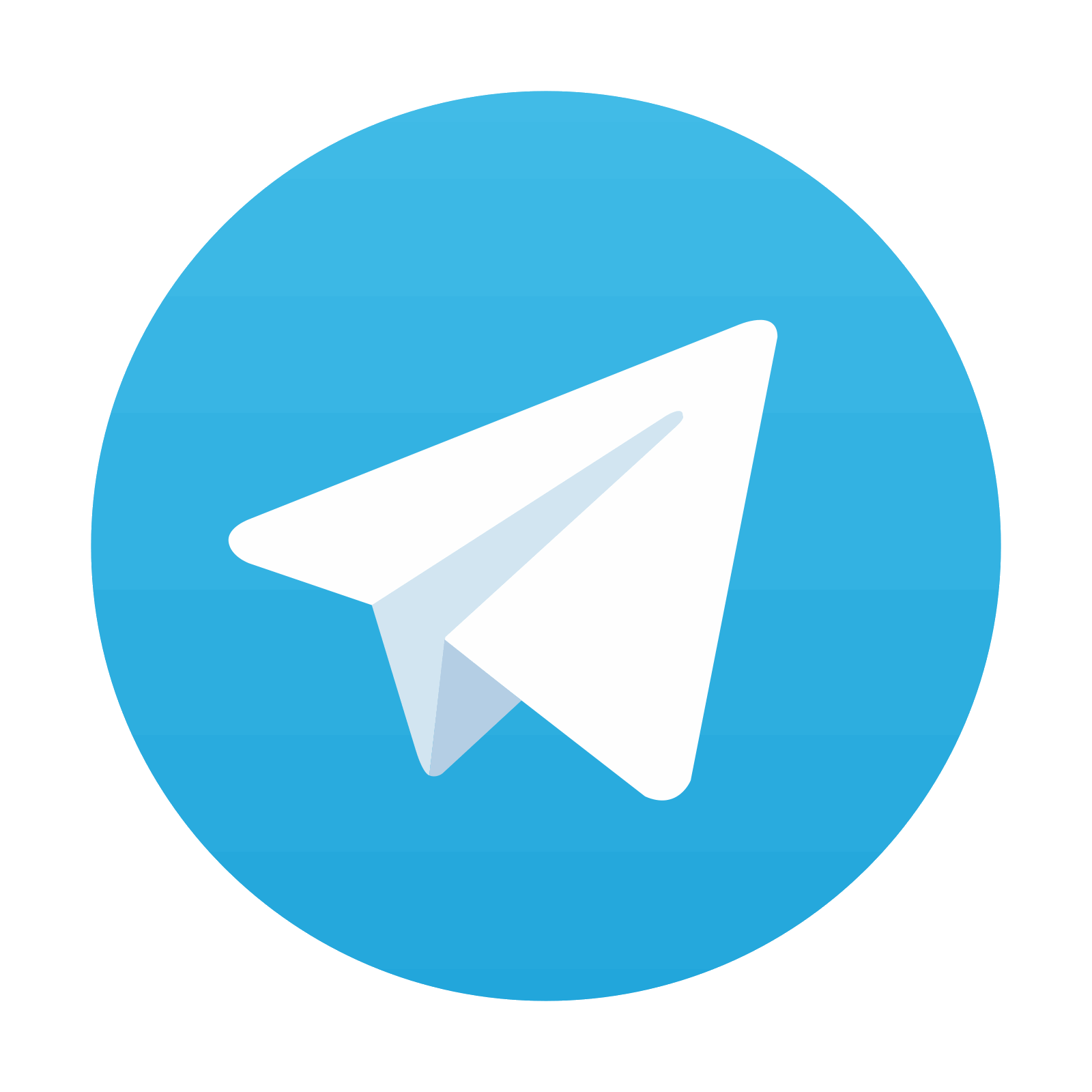
Stay updated, free articles. Join our Telegram channel
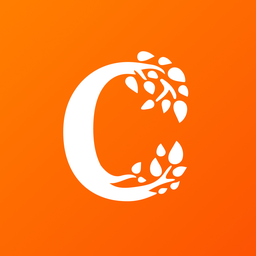
Full access? Get Clinical Tree
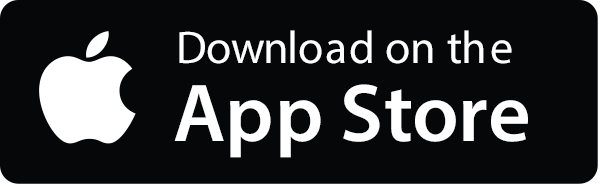
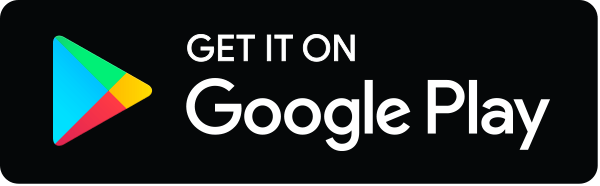