INTRODUCTION
The purpose of this chapter will be to review selected neuropathologic substrates of intractable epilepsy, including lesions that cause seizures in infants and children, as well as adults. We will not consider the pathologic findings in (usually low-grade) neoplasms that are especially common as a cause of intractable epilepsy.
PATHOLOGY OF TEMPORAL LOBE EPILEPSY
Temporal lobe epilepsy (TLE) is the most common form of epilepsy across all age groups, and hippocampal sclerosis is the most consistent pathology in this disorder. Although TLE is often diagnosed in adolescence or adulthood, the processes leading to epilepsy may have begun earlier and, in many cases, during childhood. In this context, we will consider the patterns of neuronal loss, as well as preservation, in hippocampal sclerosis; evidence for plasticity of remaining neurons, including the reorganization of their axons and synaptic connections; and a disorganization of the granule cells of the dentate gyrus that could be related to alterations in basic developmental programs for neuronal migration.
Neuronal Loss and Resultant Circuitry
Hippocampal sclerosis is characterized by several well-recognized and intriguing patterns of neuronal loss (1–3). The most common pattern includes extensive loss of neurons in three major regions of the hippocampal formation—CA1, CA3, and the hilus or polymorph layer of the dentate gyrus (Figure 5.1B; compare with a normal autopsy specimen in Figure 5.1A). In contrast, granule cells of the dentate gyrus and neurons of CA2 and the subiculum are comparatively well preserved (Figure 5.1B), even though some cell loss often occurs in these regions also. The cell loss is accompanied by astrogliosis, and the combined changes lead to a smaller than normal hippocampus (Figure 5.1A and 5.1B). This pattern of cell loss or “classical hippocampal sclerosis” is found in approximately 60% to 80% of all TLE patients with hippocampal sclerosis. However, other patterns of cell loss have been observed by numerous investigators (4–6), and recognition of the different patterns has recently led to the development of a classification system for hippocampal sclerosis in temporal lobe epilepsy by the International League Against Epilepsy (ILAE) (7). Three patterns of hippocampal sclerosis are included in this system. Hippocampal sclerosis (HCS) Type 1 is characterized by severe neuronal loss and gliosis, predominantly in CA1 and CA4, and corresponds to the pattern previously described (Figure 5.1B). A second pattern, HCS Type 2, has predominant neuronal loss and gliosis in CA1 and is found in approximately 5% to 10% of all TLE surgical cases (3). HCS Type 3 exhibits predominant cell loss in CA4, with relative preservation of CA1, and is found in approximately 4% to 7.4% of TLE surgical cases (7). This pattern of cell loss most closely resembles that of end-folium sclerosis (8), as illustrated in Figure 5.1C. An additional pattern is characterized by astrogliosis without evident hippocampal cell loss (7). This system provides a broad framework for standardizing the descriptions of cell loss in patients with TLE and could allow more consistency when correlating neuropathologic findings with patient histories, neuroimaging, and surgical outcomes.
While the new ILAE classification system is focused on neuronal cell loss in the hippocampus (CA1–CA4), identifying neuronal loss in the dentate gyrus, particularly in the polymorph or hilar region of the dentate gyrus, is also important. The polymorph region extends for varying distances (500–1000 μm) deep to the inner border of the dentate granule cell layer (9,10). In the rodent, neurons of this pyramidal cell layer (CA3) form a distinct layer, making it relatively easy to distinguish from the adjacent hilar neurons that are distributed more diffusely. However, in the human hippocampal formation, the boundary between the pyramidal cells that are located between the blades of the dentate gyrus and neurons within the polymorph layer is often more difficult to distinguish, particularly with standard histologic methods. However, the polymorph region can be delineated more clearly with histochemical methods such as Timm staining or dynorphin labeling of mossy fibers, acetylcholinesterase (AChE) staining, and immunohistochemical labeling for several groups of interneurons, including somatostatin neurons (9–11).
FIGURE 5.1 Hippocampal formation of normal human autopsy (A) and temporal lobe epilepsy (B, C) specimens that are stained with cresyl violet to demonstrate neuronal cell bodies. (A) In a control specimen, neurons are abundant throughout the hilus (H) of the dentate gyrus and in the pyramidal cell layer of all hippocampal fields (CA3, CA2, CA1) and the subiculum (Sub). (B) This TLE specimen exhibits classical hippocampal sclerosis (HCS Type 1) that is characterized by severe depletion of neurons in the dentate hilus (H), CA3, and CA1, and a smaller hippocampus (compared to the normal autopsy specimen in Panel A). Granule cells (G) of the dentate gyrus and neurons in CA2 and the subiculum (Sub) are comparatively well preserved. (C) This TLE specimen shows extensive neuronal loss in the hilus (H) and CA3 field with relative preservation of neurons in CA1, CA2, and the subiculum (Sub). This pattern of cell loss corresponds most closely to end-folium sclerosis and HCS Type 3 of the ILAE classification system. Specimens are shown at the same magnification.
While the polymorph or hilar region is not specifically considered in the current classification system, loss of neurons in the region could be very important because these include major groups of GABAergic interneurons, as well as mossy cells, which provide critical control over the activity of the dentate gyrus. In this review, the polymorph later will be referred to as the hilus, and the adjacent hippocampal pyramidal cells will be identified as CA3. This terminology will avoid the confusion associated with defining the CA4 field (7,9), and will also facilitate comparisons with morphologically and functionally comparable regions in rodents and other species. Cell loss is prominent in the hilus in both HCS Type 1 (classical hippocampal sclerosis) and HCS Type 3 (end-folium sclerosis) (Figure 5.1A–5.1C).
Despite extensive neuronal loss in human TLE, a functional circuit through the hippocampal formation is likely to remain. Even in the neuron-depleted CA1 and CA3 fields, some neurons are frequently present, either locally, within a hippocampal slice, or at different anterior–posterior levels of the hippocampus. It is presumed that such neurons continue to form a functional hippocampal circuit. Importantly, major input and output regions of the hippocampal formation, the dentate granule cells and subicular neurons, respectively, remain relatively intact. However, the normal inputs to both regions are often severely reduced as a result of cell loss in other hippocampal regions. Loss of both inhibitory gamma-aminobutyric acid (GABA) neurons and excitatory mossy cells in the hilus reduces their control of the dentate granule cells, and such loss of neurons in the hilus (sometimes included as part of CA4) is one of the most consistent findings in TLE (8,12,13). Likewise, the severe depletion of CA1 neurons considerably reduces the normal input to the subiculum. Yet the subiculum remains active and constitutes a major output of the hippocampal formation. Activity from the subiculum can be propagated to the entorhinal cortex and from there to widespread regions of the brain as well as back to the dentate gyrus in a reentrant excitatory path. Thus, despite neuronal loss, a hippocampal circuit persists, and alterations of this remaining basic circuit are likely to form the morphologic substrates for the excessive, hypersynchronous activity of epilepsy.
Reorganization of Remaining Neurons
Axonal Reorganization as a Basic Feature of TLE Pathology. Although, for many years, the focus of pathologic studies of TLE has been on neuronal loss, it has become increasingly clear that the remaining neurons and their connections are a critical part of the “pathology of epilepsy.” When the initial cell loss occurs in childhood, the remaining neurons are present during a period when the nervous system has the capacity for the greatest developmental plasticity. In particular, processes such as axonal growth and synaptogenesis could be stimulated. Indeed, numerous studies now suggest that reorganization of the synaptic connections of the remaining neurons is a central feature of the morphologic changes in TLE. This axonal reorganization appears to occur in both the principal cells (granule cells of the dentate gyrus and pyramidal cells of the hippocampus) and the GABAergic interneurons.
Reorganization of Principal Cells. The reorganization or sprouting of mossy fibers that originate from dentate granule cells is one of the most striking alterations in the hippocampus of humans with TLE. Since the early descriptions of mossy fiber sprouting in human epilepsy (10,14,15), numerous studies have confirmed the findings in a very high percentage of cases [eg, (16,17)].
The mossy fibers and their axon collaterals normally innervate neurons in the hilus and CA3; however, in many patients with TLE, the mossy fibers are distributed aberrantly in the inner molecular layer, where they form a distinct band above the granule cell layer (Figure 5.2A and 5.2C). This contrasts sharply with the distribution of mossy fiber terminals in normal tissue where labeled mossy fibers are highly concentrated around neurons in the hilus but are not evident in the inner molecular layer (Figure 5.2B).
The inner molecular layer is normally innervated by excitatory mossy cells of the hilus, and many of these neurons are frequently lost as part of the broad loss of neurons in the hilus. Loss of mossy cells could serve as a major stimulus for reorganization of mossy fibers into these regions because, through their loss, many of the normal targets of the granule cells in the hilus are missing (10). Also, synaptic sites on granule cells in the inner molecular layer are vacated as a result of degeneration of mossy cell terminals. However, despite the frequent occurrence of both mossy fiber sprouting and mossy cell loss, a direct link between these changes has not been established.
In addition to the frequency with which mossy fiber sprouting is observed in human TLE, as well as in many epilepsy models, ultrastructural findings provide strong support for the creation of an aberrant excitatory circuit in the dentate gyrus in TLE. The reorganized mossy fibers in human TLE form distinct synaptic contacts in the molecular layer (15,18,19). Moreover, the morphologic features of the reorganized mossy fiber synapses suggest that they could exert strong excitatory influences. In human TLE, the reorganized mossy fiber terminals are packed with clear synaptic vesicles (Figure 5.2D), presumably containing glutamate, and contain numerous mitochondrial profiles, suggesting that the terminals are functional and capable of strong, excitatory influences. Many of the terminal profiles in humans are quite large (Figure 5.2D), with a mean major diameter of 2.3 µm, and this exceeds the size of most other axon terminals in the region (19).
The reorganized mossy fiber terminals in humans establish distinct, asymmetric synapses that are characteristic of excitatory synapses, and a single terminal often establishes multiple synaptic contacts (Figure 5.2D). The large aberrant mossy fibers are indented and invaginated by multiple spines (19), and such patterns are characteristic of the final stages of normal mossy fiber development in CA3, when complex spines are formed (20). Thus, reorganized mossy fibers in the human dentate gyrus appear to have progressed through their full development and show synaptic organization that is consistent with a powerful, aberrant, re-excitatory circuit among granule cells.
Whether the reorganized mossy fibers form synaptic contacts with inhibitory neurons or other granule cells in the dentate gyrus is important for determining their function, and the predominant target of sprouted mossy fibers in animal models continues to be debated (21–23). However, in human TLE, granule cell dendrites appear to be the most common target, with the vast majority of synaptic contacts being formed with dendritic spines and the remainder with dendritic shafts of dentate granule cells (15,18,19).
FIGURE 5.2 Dynorphin labeling of mossy fibers in the human hippocampus. (A) In a human temporal lobe epilepsy (TLE) specimen, a dense band of labeled fibers (arrows) is present in the inner molecular layer of the dentate gyrus and indicates an aberrant distribution of mossy fibers from dentate granule cells (G). Normally, such mossy fibers are restricted to the dentate hilus (H) and CA3. (B) In a control autopsy specimen, dynorphin-labeled mossy fibers are concentrated around neurons in the hilus (H) and are not present in the granule cell (G) and molecular (M) layers. (C) In a TLE specimen, dynorphin-labeled mossy fibers are concentrated in the inner molecular layer (M), and the normal labeling around cell bodies in the hilus (H) is reduced due to loss of neurons in this region. (D) In an electron micrograph of a TLE specimen, a dynorphin-containing mossy fiber terminal (MF) in the inner molecular layer contains numerous clear synaptic vesicles as well as several dense core vesicles. The terminal forms distinct, asymmetric synaptic contacts (arrows) with several dendritic profiles (D). The size of the mossy fiber terminal is considerably larger than that of another terminal (T) in the region.
The sprouting of mossy fibers may not be limited to the dentate gyrus but may extend to pyramidal cells of CA2 (10,24). Interestingly, in CA2, axon terminals with the characteristics of mossy fiber terminals formed asymmetric synapses on the cell bodies of CA2 pyramidal cells where they could have a particularly strong excitatory influence on persisting CA2 pyramidal cells.
Axonal reorganization of other excitatory neurons is also likely to occur in TLE. In the dentate gyrus, the normal projection from the supramammillary region to the inner molecular layer appears to have increased, as indicated by an expanded band of calretinin labeling (25). Thus, growth and reorganization of extrinsic as well as intrinsic excitatory projections may occur in the dentate gyrus in TLE and contribute to enhanced excitability in the region.
Axonal reorganization may also occur in CA1 and other regions where there is partial loss of principal neurons. Aberrant collateral sprouting of CA1 pyramidal cell axons into the pyramidal cell layer and stratum radiatum has been described in human TLE following injections of fluorescent tracers in hippocampal slices (26). Such network reorganization could contribute to increased excitability and synchrony among the neurons in each region.
However, reorganized mossy fibers in the dentate gyrus provide the clearest example to date of axonal reorganization and new synapse formation in the human central nervous system (CNS), and studies of human epilepsy tissue have been instrumental in demonstrating such plasticity in the adult human brain. Yet, despite the robustness of mossy fiber sprouting and the appeal of recurrent excitatory circuits as a basic mechanism in epilepsy, the functional significance of mossy fiber sprouting and its contribution to epileptogenesis remains unclear (27,28).
Some functional influences have been demonstrated. In in vitro studies of the dentate gyrus in patients with TLE, impaired inhibition was correlated with the extent of mossy fiber sprouting (18). Also, in an animal model of epilepsy with extensive mossy fiber sprouting, effects of the reorganized mossy fibers were observed when inhibition was reduced (29). Thus, the functional impact of mossy fiber sprouting may be closely linked to the state of inhibition within the neuronal network.
Loss and Reorganization of GABA Neurons. The general histologic view of neuronal loss and preservation in TLE emphasizes the principal, excitatory neurons that form the basic circuitry of the hippocampal formation. The fate of GABA neurons that control activity within the circuit has been more difficult to determine. Indeed, early reports appeared to be contradictory, with some studies suggesting that GABA neurons are preserved in the hippocampus of humans with TLE (30) and others demonstrating loss of specific groups of peptide-containing neurons (11,31) that are now known to be subgroups of GABA neurons.
Current findings indicate that the changes in GABA neurons in human TLE are indeed complex but fascinating. First, different classes of GABA neurons appear to have different vulnerabilities, according to whether they innervate dendritic regions or perisomatic sites that include cell bodies and axon initial segments (13,32). Within the dentate gyrus, neurons that terminate in dendritic regions are among the most vulnerable to damage, and many of these neurons are labeled with somatostatin or neuropeptide Y (NPY). Loss of these neurons in human tissue was demonstrated initially by de Lanerolle et al (11,33), and their loss has been confirmed in subsequent studies (34,35). Because many of the cell bodies of somatostatin- and NPY-containing neurons that innervate dentate granule cells are located in the hilus, the loss of hilar neurons in TLE provides additional support for degeneration of these groups of neurons, perhaps as a result of an initial precipitating event (36,37). This would be consistent with the extreme vulnerability of somatostatin neurons in animal models following status epilepticus, ischemia, and traumatic head injury (38–42). These hilar GABA neurons (Figure 5.3A) normally project to the dentate molecular layer, where they help regulate the responses of granule cells to excitatory input from the entorhinal cortex, and loss of these GABA neurons (Figure 5.3B) could increase granule cell excitability in response to such input.
Despite the marked loss of hilar neurons in many patients with TLE, a plexus of somatostatin- and NPY-containing fibers, as well as GABAergic terminals, can often be found in the molecular layer at chronic stages of epilepsy (11,31,35) (Figure 5.3A and 5.3B). Such findings have suggested that remaining GABA neurons within the region may have reorganized their axonal connections. However, another possibility is that the relevant peptides, GABA, or its synthesizing enzyme, glutamate decarboxylase (GAD), could be increased in remaining terminals, thus creating the appearance of abundant axon terminals. Recently, using mice that express green fluorescent protein (GFP) in a subgroup of somatostatin neurons, Buckmaster and colleagues demonstrated convincingly that remaining somatostatin neurons in the hilus undergo axonal sprouting and reinnervate regions that had lost their original somatostatin/GABAergic innervation (44). Such axon terminals have generally been presumed to have compensatory effects. However, in the human dentate gyrus, as in animal models, the labeled fibers no longer exhibit a normal laminar pattern in the molecular layer (Figure 5.3A and 5.3B) and, in some cases, the fibers appear larger and more prominent (11,35). Also, in human TLE tissue, the somatostatin receptor remains decreased in the dentate molecular region (35). Thus, the overall functional effects of such presumed axonal reorganization remains uncertain, but it is unlikely to compensate fully for loss of the normal, highly organized GABAergic innervation of the dendritic region.
FIGURE 5.3 GABAergic innervation of the human dentate gyrus in normal autopsy (A) and temporal lobe epilepsy (TLE) (B) specimens, as indicated by glutamate decarboxylase immunolabeling and schematics of somatostatin/GABAergic reorganization in a mouse model of epilepsy (C,D). (A) In the normal dentate gyrus, the cell bodies of numerous GABA neurons (examples at arrows) are located throughout the dentate hilus (H) and these neurons contribute to the rich GABAergic innervation of the outer molecular layer (Mo) and lower levels of labeling in the inner molecular layer (Mi). (B) In a TLE specimen, labeled GABAergic neurons in the hilus (H) are severely depleted. However, numerous labeled fibers and axon terminals are evident in the outer molecular layer (Mo), possibly due to reorganization of the remaining GABA neurons. The distinction between the inner and outer molecular layer (Mi and Mo) is less evident than in the normal specimen (compare with Panel A). (C,D) Schematics illustrate one pattern of SOM/GABAergic axonal reorganization that has been identified in the hippocampus in a mouse model of epilepsy. In a control mouse (C), selective labeling of (SOM) neurons in stratum oriens (O) of CA1 leads to labeling of their axon terminals that are confined to stratum lacunosum-moleculare (LM) of the hippocampus and do not cross the hippocampal fissure. SOM neurons (red) in the hilus (H) innervate the outer molecular layer (M) of the dentate gyrus where they form synapses with dentate granule cells (G, blue). In a mouse model of epilepsy (D), similar labeling of SOM neurons in s. oriens leads to axonal labeling not only in stratum lacunosum-moleculare of CA1 but also in the molecular layer of the dentate gyrus, a region that was previously innervated by vulnerable SOM neurons (red) in the hilus. Thus, loss of SOM neurons in the dentate hilus leads to sprouting of remaining SOM neurons in the hippocampus, creating a reorganized but aberrant pathway (43).
In most studies of GABAergic axonal reorganization, it has been assumed that the reorganized fibers are originating from interneurons of similar types to those that were lost and “recreating” the basic circuity, and thus will be compensatory. However, recent studies of a mouse model of epilepsy have demonstrated that aberrant GABAergic circuits can be created following severe loss of SOM neurons within the dentate hilus (43). Contrary to expectations, somatostatin neurons in stratum oriens of the hippocampus exhibited robust sprouting, and many of the fibers crossed the hippocampal fissure and innervated the molecular layer of the dentate gyrus, where they formed functional synaptic connections with dentate granule cells (Figure 5.3D). These somatostatin interneurons in CA1 do not normally innervate the dentate gyrus (Figure 5.3C); thus, an aberrant circuit has been created that would no longer be activated by the normal dentate pathways.
These findings add a new dimension to the changes that can occur following loss of subpopulations of GABAergic neurons. The results suggest that, despite the abundance of GABAergic axon terminals that are often observed in regions with prior loss of GABA neurons, some of these axons may not be part of the normal circuit. This could limit their effectiveness in controlling activity within the dentate gyrus, particularly during periods of increased excitability when such inhibitory circuits could be most important (45).
In contrast to a severe loss of hilar GABA neurons that innervate granule cell dendrites, those that innervate perisomatic regions in the dentate gyrus and hippocampus may be relatively well preserved (46,47). Many of these neurons normally express the calcium-binding protein parvalbumin, and several studies of human TLE have reported a decrease in parvalbumin labeling in the dentate gyrus, CA1, and CA2 (24,48–50). Although this could reflect a loss of these GABA neurons, electron microscopic studies have found that many GABAergic axon terminals around the cell bodies and axon initial segments, presumed to originate from parvalbumin-containing neurons, are preserved (46,47,50). Thus, the decreased parvalbumin labeling has been interpreted by some investigators as a decrease in parvalbumin content in persisting interneurons (24,47). In regions such as the dentate gyrus, the number of GABAergic terminals and complexity of the innervation around the cell bodies and along the axon initial segments are even increased (46,51). Such alterations have again suggested sprouting of the remaining GABA neurons.
While such changes could be viewed as compensatory, Maglóczky and Freund have suggested that the maintained or increased GABAergic innervation on the cell bodies and axon initial segments of projection neurons could contribute to enhanced, abnormal synchronization of neuronal firing (32). This is consistent with current views of GABAergic function, in which GABAergic innervation at perisomatic locations is considered to be critical for the normal synchronization of ensembles of principal cells (52).
The complexity of changes in GABAergic innervation in TLE has been emphasized in detailed studies of axo-axonic cells by DeFelipe (50) and others (51). Despite what appeared to be a general preservation of perisomatic GABAergic innervation in human TLE, a loss of GABAergic terminals along the axon initial segments of some granule cells was identified (51). When a loss was observed, it was often extreme and could be sufficient to severely decrease inhibitory influences at the axon initial segment, which strongly influences the final output of the projection neurons (51). These findings suggest that loss of even a small number of GABA neurons could severely deplete the GABAergic innervation at critical locations on remaining principal cells, and such projection neurons could feasibly become sites of seizure initiation.
Calbindin-containing interneurons are an additional group of GABA neurons that may be preserved in several regions of the hippocampal formation (25,48). However, these persisting neurons undergo numerous morphologic changes that include increased numbers of spines and increased GABAergic innervation (53,54). Increased inhibitory control of inhibitory interneurons could potentially limit their output and lead to decreased inhibitory control of the principal cells.
Finally, although no loss of GABA neurons and their terminals has been observed in the subiculum (51), functional alterations in GABAergic signaling have recently been described in this region. In a subgroup of pyramidal cells of the subiculum of human TLE specimens, GABA had unexpected depolarizing actions, and the resultant activity contributed to rhythmic interictal discharges (55). These responses are reminiscent of GABA-mediated excitation that occurs during early development and is related to late expression of the potassium-chloride cotransporter (KCC2) that normally extrudes Cl– from the cell (56). Further studies have demonstrated both downregulation of the KCC2 transporter mRNA and upregulation of the Na+-K+-2Cl– (NKCC1) transporter mRNA, a transporter that facilitates accumulation of Cl– within neurons, in the subiculum in human TLE (57). Interestingly, similar changes were not found in the hippocampus or temporal neocortex in these studies or in CA2 in a subsequent study (24), suggesting that such changes in chloride homeostasis could be region specific. Nevertheless, a functional reversion of some neurons in TLE to earlier developmental stages, when GABA has excitatory actions, could substantially alter GABAergic influences and possibly contribute to seizure activity (58–60).
Current findings thus suggest that GABA neurons and their function can be altered in multiple ways in TLE. Clearly some groups of GABA neurons are vulnerable to damage, and their loss could reduce GABAergic control of principal cells, particularly at dendritic sites, where they receive their major excitatory input. In contrast, many GABA neurons remain viable, may exhibit substantial axonal reorganization, and could either have compensatory effects, create aberrant circuitry that could be less effective than the original circuits, or contribute to enhanced neuronal synchronization. Finally, some remaining GABA neurons could exert excitatory influences in specific regions of the hippocampal formation.
Reorganization of the axons and synaptic connections of both principal cells and GABAergic interneurons now appears to be a central component of hippocampal pathology in TLE. Interestingly, the sprouting of excitatory neurons, as well as inhibitory neurons that innervate perisomatic sites, could contribute to enhanced synchrony of the projection neurons of the circuit, although through different mechanisms (32,61). Determining the “rules” and the cellular and molecular mechanisms that govern such reorganization are critical for developing interventions that could limit the formation and maturation of aberrant synaptic connections that are likely to contribute to seizure activity.
Granule Cell Disorganization
Altered Patterns of Dentate Granule Cells. In many patients with TLE and hippocampal sclerosis, the granule cells of the dentate gyrus are one of the most persistent groups of neurons. However, in a subpopulation of these patients, the granule cells have a disorganized appearance that contrasts sharply with the compact, relatively narrow band of granule cells in the normal hippocampus (Figure 5.4A). The most common altered pattern has been described as granule cell dispersion (62) and is characterized by a wider than normal granule cell layer, increased space between many of the granule cells, and poorly defined laminar borders (Figure 5.4B). The granule cells are often aligned in vertical rows that extend into the molecular layer to varying extents and create an irregular outer border of the granule cell layer (Figure 5.4D). The vertical alignment of dispersed granule cells and their elongated appearance are remarkably similar to those of developing neurons as they migrate along radial glia (62) (Figure 5.4D). Recent studies have demonstrated a close approximation between dispersed granule cells and radial glia fibers and found that the length of the glial fibers increased with the severity of granule cell dispersion (63).
In several series of TLE patients, granule cell dispersion has been found in approximately 40% to 50% of TLE cases (16,17,64–66). Thus, while this alteration is not an essential feature of hippocampal sclerosis, it occurs frequently and is of interest because it suggests alterations in the normal development of the dentate gyrus that could be associated with altered circuitry and contribute to abnormal function of the region.
Another abnormal pattern is a bilaminar distribution of granule cells in which two layers of granule cells are separated by a clear region that is relatively devoid of neuronal cell bodies (62,67) (Figure 5.4C). The bilaminar pattern is observed less frequently than generalized dispersion and has been found in approximately 10% to 14% of TLE cases (17,66). Within dispersed and bilaminar regions, granule cells are numerous, and the laminar alterations do not appear to result primarily from granule cell loss. However, distinct regions of granule cell loss are frequently found in the granule cell layer (Figure 5.4C). In a recently described classification system, granule cell dispersion and bilamination of the granule cell layer, as well as clusters of ectopic neurons in the molecular layer, have been included in a general category of architectural abnormalities of the granule cell layer, thus suggesting that these three patterns reflect variations of a broader process of structural dentate gyrus pathology (66). Nevertheless, each pattern is sufficiently unique to suggest somewhat different mechanisms that could be important to identify.
An additional pattern of granule cell pathology is a thinning of the granule cell layer and substantial loss of granule cells (66). This pattern has been reported in approximately 38% of patients with temporal lobe epilepsy and was most frequently observed in patients with a long history of TLE, raising the possibility that such loss could reflect either ongoing neuronal damage or a reduction in normal adult neurogenesis, as has been observed in some animal models of epilepsy (66).
FIGURE 5.4 Granule cell organization in the human hippocampus of control autopsy (A) and temporal lobe epilepsy (TLE) (B, C, D) specimens as demonstrated by NeuN immunolabeling. (A) In control tissue, the granule cells of the dentate gyrus are closely approximated and form a relatively narrow, compact granule cell layer (G). (B) In a TLE specimen, granule cells (G) are dispersed, creating a widened layer with uneven borders. Granule cell somata are more widely separated than normal, particularly in the outer part of the layer. (C) In another TLE specimen, the granule cells (G) form a bilaminar pattern with a relatively cell-free zone between the two layers of neurons. Short segments of severe cell loss are found periodically within the bilaminar granule cell layer. Extensive loss of neurons is evident in the hilus (H), CA3, and CA1. (D) In a TLE specimen with granule cell dispersion, many of the NeuN-labeled granule cells have elongated cell bodies (examples at arrows) with vertically oriented processes and often appear to be aligned in columns. Numerous granule cells extend beyond the normal granule cell layer (G) into the molecular layer (M). Dispersed neurons are also evident in the hilus (H).
Interestingly, when the two broad types of granule cell pathology (severe granule cell loss and granule cell dispersion) were evaluated in those TLE patients with hippocampal sclerosis, virtually all cases had granule cell pathology, with significant cell loss in 40% and granule cell dispersion (including all subtypes) in 60% of the patients (66).
The functional significance of these alterations in dentate granule cells remains unknown, but the findings emphasize the importance of the dentate gyrus as a critical region for study in human TLE. Of particular interest is the suggestion that alterations in dentate granule cells could be closely associated with impaired memory performance in human TLE (68).
Possible Mechanisms of Granule Cell Dispersion. The basic mechanisms responsible for granule cell dispersion in human TLE have not been determined, but recent studies of human tissue and various animal models have suggested several interesting possibilities. Two general hypotheses are being explored. The first is that the alterations reflect an intrinsic, developmental migration disorder. The second is that the altered positioning of the granule cells is acquired as a result of an initial precipitating event such as severe febrile convulsions, status epilepticus, or, possibly, repeated seizure activity. Support can be found for both hypotheses.
The presence of granule cell dispersion has generally been restricted to patients with TLE and has seldom been reported in autopsies of neurologically normal individuals or those with other neurologic disorders. However, Harding and Thom (69) described bilateral granule cell dispersion in autopsy studies of three pediatric cases. In one case, the child presented with a severe seizure disorder, and bilateral hippocampal sclerosis accompanied the granule cell disorganization. In two other cases, no seizures were documented, and the remainder of the hippocampal formation appeared intact. In all three cases, heterotopias or polymicrogyria were also present. The presence of bilateral granule cell dispersion and other migrational defects suggested that granule cell dispersion could be an independent developmental disorder in at least a small subgroup of cases (69).
Disorganization of dentate granule cells has also been observed in several mouse models with genetic mutations, and these include the Reeler mouse, which lacks the Reelin protein, and the p35-deficient mouse. However, some differences exist between the patterns of neuronal lamination in human TLE and those in the mutant mice. In genetic models with altered neuronal migration, multiple groups of cortical and hippocampal neurons are often affected. Yet, in most TLE cases with granule cell dispersion, other regions of the hippocampal formation do not show noticeable laminar disruption (Figure 5.4C). In addition, granule cell dispersion in human TLE is frequently accompanied by severe cell loss in other regions of the hippocampus, and similar patterns of cell loss are not found in the mutant mice.
The association between granule cell dispersion and neuronal loss suggests that either an initial precipitating event or recurrent seizures could have influenced the positions of the granule cells. In several studies of human TLE, a strong association has been found between neuronal loss in the hilar (polymorph) region and the presence of granule cell dispersion (62,65,70). Similarly, in a recent study of a large number of TLE cases, Thom et al found that granule cell dispersion correlated more closely with the extent of neuronal loss throughout the hippocampal formation than with any other variables examined (17).
This relationship, when viewed in conjunction with findings from mutant mice with altered granule cell lamination, suggests that the initial precipitating event could have produced specific cellular and molecular changes that, in turn, cause the altered patterns of granule cells. Several potential candidate molecules have recently been identified.
Reelin is an extracellular matrix protein that is important for the proper lamination of the cerebral cortex and hippocampus, and loss of Reelin, as occurs in the Reeler mutant mouse, leads to a severely disordered cortex and hippocampus, including nearly complete loss of a compact granule cell layer (71,72). In the hippocampus, Reelin is synthesized and secreted by Cajal–Retzius cells, which are located in the outer molecular layer of the dentate gyrus and are particularly prominent during development (73,74), as well as hilar somatostatin neurons (75,76).
Studies by Haas et al have found that Reelin mRNA expression in Cajal–Retzius neurons of the dentate gyrus is reduced in some patients with TLE (77). Furthermore, the amount of loss of Reelin mRNA-labeled cells was correlated with the extent of granule cell dispersion into the molecular layer. Also, Reelin-neutralizing antibodies in the hippocampus of normal adult mice induced granule cell dispersion (63). These and other findings suggest that Reelin plays an important role in the formation of a compact granule cell layer and that Reelin deficiencies are strongly associated with increased neuronal mobility, with the Reelin deficiency possibly reducing phosphorylation of the actin-depolymerizing protein cofilin (78).
However, it is still not known whether the reduction in Reelin is primarily related to loss of Reelin-synthesizing cells, such as Cajal–Retzius cells and GABAergic somatostatin hilar neurons; a reduction in Reelin expression within remaining cells; altered Reelin processing (79,80); or a compromised Reelin signaling pathway, including increased Reelin promotor methylation (81).
Recently, an interesting link between seizure activity and granule cell mobility has been demonstrated in hippocampal slice cultures (82). The application of kainate, a glutamate receptor agonist, induced epileptiform activity in dentate granule cells and led to increased mobility of differentiated granule cells and spread of the granule cells into the dentate molecular layer. The increased motility of the granule cells was associated with nuclear translocation (82,83), a process that is generally thought to be limited to immature neurons. Interestingly, these changes were associated with decreased Reelin expression due to degeneration of interneurons in the hilar region. This is consistent with previous suggestions of an association between hilar neuron loss and granule cell dispersion in human TLE (62,66).
These findings raise the possibility that granule cell dispersion in human TLE could involve similar processes and that repeated seizure activity might contribute directly to the increased spread of granule cells and the appearance of granule cell dispersion (82). These ideas are provocative and suggest that granule cell dispersion could be an ongoing process. They also raise questions as to what additional factors lead to the development of granule cell dispersion in some, but not all, patients with medically intractable TLE.
Granule cell positioning could also be influenced by brain-derived neurotrophic factor (BDNF). In a mouse model of TLE that is produced by the intrahippocampal injection of kainate, granule cells become severely dispersed and enlarged (84). In this model, levels of BDNF were significantly elevated and could have stimulated migration of the granule cells as well as their increased size (85,86). Injection of BDNF into the hilus in rodents also leads to increased numbers of ectopic granule cells in this region, possibly due to aberrant migration from the subgranular layer (87). Interestingly, increased BDNF mRNA and protein levels also have been found in humans with TLE (88,89).
The p35 signaling pathway, involved in neuron-specific activation of cyclin-dependent kinase 5 (Cdk5), could also be involved in granule cell dispersion and mossy fiber sprouting. In p35-deficient mice, lamination of the dentate gyrus is altered, and the distribution of granule cells closely resembles that of granule cell dispersion in TLE patients (90). Furthermore, these mice demonstrated spontaneous seizures (91).
Altered migration of granule cells along radial glia or failure of the neurons to leave the scaffold could be a common mechanism underlying granule cell dispersion, through either the Reelin or p35 signaling pathway (92,93). Similarly, immature astrocytes and increased expression of several neural cell adhesions and related molecules could contribute to the altered cellular architecture of the dentate gyrus in TLE (94,95).
Finally, evidence for the development of granule cell dispersion following an initial insult, rather than from an intrinsic developmental abnormality, has come from a study of the effects of limbic-like seizures in normal immature monkeys (96). Following a unilateral infusion of bicuculline in the entorhinal cortex and the production of limbic-like seizures, histologic changes that resembled hippocampal sclerosis developed, and these included granule cell dispersion, in addition to hippocampal cell loss and mossy fiber sprouting (96). Thus, many of the changes observed in humans with TLE can be produced by an initial episode of status epilepticus in a normal, immature primate brain. Although spontaneous seizures were not detected up to 1 year after the initial seizure episode in these animals, it seems plausible that a longer postseizure period (years) might be required for such seizures to develop.
Renewed interest in neurogenesis in the dentate gyrus during postnatal life and evidence that this process can be stimulated by seizure activity (97) have led to speculation about the possible contributions of newborn neurons to granule cell disorganization as well as mossy fiber sprouting. The results of recent studies to determine whether newly generated neurons contribute to granule cell dispersion in patients with TLE have differed. In some studies, immunohistochemical markers of neural stem cells and progenitors, as well as immature glia, have been observed in the dentate gyrus and in regions of dispersion (98,99). In contrast, other investigators, using similar markers for newly generated neurons in human surgical tissue, have found no evidence for such neurons in regions of dispersion (92) and have concluded that displacement of mature neurons, rather than newly generated neurons, leads to granule cell dispersion in the epileptic hippocampus (63,92). Some of the discrepancies could be related to the lack of reliable markers of neurogenesis in human tissue.
When considering neurogenesis as well as other types of morphologic plasticity in TLE, a distinction between changes that occur at the time of an initial precipitating event and those that may occur as a result of later, spontaneous seizures could be particularly important. The initial event, such as a severe, prolonged seizure, is likely to stimulate neurogenesis acutely, as has been demonstrated in numerous animal models [eg, (97,100)]. Although the extent of neurogenesis in humans appears to be considerably less than that in rodents (101), any increased production of granule cells could be significant. The newly generated neurons may then require a period of time to mature and become completely integrated into the hippocampal circuitry, that is, several months in rodents (102,103) and perhaps longer in humans. Events during this period could be of considerable importance for the functional integration of the newborn neurons (104) and could determine whether granule cells reach normal or abnormal positions, form proper or aberrant connections, and thus compensate for or contribute to an epileptic state.
The effects of spontaneous seizures are likely to differ from those of the acute events. Indeed, several studies have suggested a reduction of neurogenesis in the chronic pilocarpine and intrahippocampal kainate models of recurrent seizures (63,105,106), as well as in human pediatric patients with frequent extratemporal seizures (107). Evidence for marked depletion of granule cells in some TLE patients with long seizure histories also suggests that adult neurogenesis may be compromised in patients with TLE. The possible relationships between adult neurogenesis and epilepsy remain intriguing and are discussed in detail in several recent reviews (108–110).
NEOCORTICAL LESIONS ASSOCIATED WITH SEIZURES
Structural lesions (aside from TLE, see previous discussion) associated with epilepsy can be grouped into the following categories: (a) malformative, (b) neoplastic, (c) familial/metabolic, (d) vascular/traumatic, and (e) infectious/inflammatory (111–113). Investigators have suggested that some of these pathologic processes may overlap in epilepsy patients (114,115) and “dual pathology” (eg, a cortical resection specimen showing features of both a malformative or neoplastic and destructive lesion) is occasionally encountered (116). Individuals with HS/TLE may also have a neocortical lesion such as a low grade neoplasm, hemangioma, or a focus of dysplasia; hippocampal pyramidal neuron loss in such instances may be less severe than in “pure” HS (117). Some causal lesions—as with TLE, see previous discussion—are amenable to definitive surgical treatment (eg, low grade neoplasms, malformations of cortical development). This section will focus on developmental malformations and destructive or inflammatory lesions of the neocortex that account for the majority of structural lesions seen in infants and children with intractable epilepsy, especially those with infantile spasms (IS). More subtle malformations are also recognized with increasing frequency in adults with epilepsy; on rare occasions, severe dysplastic lesions may, for unknown reasons, only present to a neurologist or epileptologist in adulthood. The advent and growth of epilepsy surgery beginning in the mid-20th century led to the opportunity for neuropathologists to examine brain lesions that were presumed to be the cause(s) of a seizure disorder, rather than the result of same. Table 5.1 summarizes the overarching goals of studying surgically resected cortical tissue from a given patient with seizures; some special stains and immunohistochemical procedures that are helpful in characterizing (even quantifying) neuropathologic abnormalities are mentioned in this table. Autopsy studies of brains originating from patients with seizures are valuable, to be sure, but present difficulty to the neuropathologist in deciding what the cause of seizures is vs. what changes in the CNS are the result of prolonged seizures and the treatment thereof.
TABLE 5.1
GOALS OF STUDYING BRAIN TISSUE FROM EPILEPSY SURGERY |
1 What Is the Lesion? H&E, Kluver–Barrera stains for routine histology Immunohistochemistry: GFAP (gliosis), NeuN (neuronal architecture and cytologic features), neurofilament epitopes, inflammatory markers (CD3, CD20, Iba1+, CD68) Cytoskeletal markers (neurofilament, tau) Synaptic proteins (especially synaptophysin) may assist in localizing neuronal heterotopias within subcortical white matter. 2 What Caused the Lesion? Usually difficult to answer Immunohistochemistry, in situ hybridization, viral culture, or PCR analysis may help or provide clues. 3 How Was the Lesion Producing Seizures? May be impossible to answer Correlative electrophysiologic recordings and further neuropharmacologic evaluation may be helpful. |
MALFORMATIONS OF CORTICAL DEVELOPMENT (MCD)
Malformations of cortical development (MCDs) have usually been considered to represent disorders of neuronal migration (or vascular formation) and have variously been classified with regard to morphology or putative etiology (112,118,119). The classification of these lesions is increasingly being influenced and modified by (a) the availability of high-resolution multimodality neuroimaging techniques—including metabolic imaging (PET scanning) using a variety of tracers and ligands—that can be used to predict pathologic abnormalities in a given patient (119–124), (b) molecular genetic clues to pathogenesis, based upon techniques such as gene expression profiling following laser capture microdissection of surgical specimens (125), and (c) our ability to study them using increasingly novel immunoreagents and molecular probes. The genetic basis of some types of lissencephaly (eg, Miller–Dieker syndrome, obviously not a surgically treatable lesion) is now well understood (125,126). [Genetic considerations in epilepsy and the genetic basis of cerebral malformations are discussed in detail elsewhere in this volume and in excellent reviews, see 118]. They can be categorized as follows: (a) cortical dysplasia (CD), which accounts for the majority of malformations associated with pediatric epilepsy and encompasses the full spectrum of neuronal migration disorders (NMDs) or MCDs, ranging from the most subtle to the most severe; (b) CNS structural lesions associated with tuberous sclerosis complex (TSC); (c) Sturge–Weber–Dimitri syndrome (SWDS), or encephalotrigeminal angiomatosis; (d) neurofibromatosis type II, which may be associated with meningio-angiomatosis; and (e) vascular malformations, especially cavernous hemangiomas and arteriovenous malformations (AVMs) (111–113,127).
Clinicopathologic Considerations
Seizure disorders have traditionally been subdivided into syndromes based on clinical presentation and electroencephalographic (EEG) findings. However, there is often a marked discrepancy between the clinical phenomenology of a seizure disorder and its neuropathologic substrate(s). Infantile spasms (IS, West syndrome) and Lennox–Gastaut syndrome can be seen with a wide range of cerebral lesions (128–130). The clinical form of epilepsy seen in a given patient appears to depend more on when during cerebral development the lesion occurred than on the specific type or topographic distribution of lesions (128–131). It has been suggested that the CNS lesions associated with IS can be functionally categorized into three groups: (a) diffuse structural abnormalities, (b) focal or multifocal cerebral lesions, and (c) cases with minimal neuropathologic change (130). Diffuse hemispheric lesions include hemimegalencephaly (HME), agyria/pachygyria-lissencephaly, and Aicardi syndrome (132). Focal and multifocal lesions include CD/MCD and destructive lesions (vascular and infectious), as well as cortical tubers seen in patients with TSC.
MCDs can be viewed as a spectrum of CD resulting from derangement of the normal process of cortical development (119–121,133–137)—a brief discussion of which is presented in the following text. This spectrum consists of a range of morphologic features associated with multiple putative etiologic factors, including genetic and environmental (eg, toxic, destructive) influences. CD encompasses a broad range of neocortical malformations, ranging from the most subtle (“microdysgenesis”) to the most severe (HME), and includes such conditions as agyria/pachygyria-lissencephaly, polymicrogyria (PMG), and focal CD. The resultant neuropathologic features may reflect abnormalities that probably occur within well-defined time windows during brain development. Clinically there is an inverse correlation between the extent and severity of CD and the age of the child at clinical presentation (123,133), supporting the notion that there is a predominance of pathologically severe CD in neonates and infants with seizures, including those with IS (128,130). Although CD accounts for the majority of malformations associated with pediatric epilepsy, other malformative lesions are also capable of producing these epilepsy syndromes, and they frequently show neuropathologic changes that overlap significantly with those seen in patients with CD. Jellinger (128) has noted that the clinical severity of a given seizure disorder appears to be more closely related to the timing of the insult (whether genetic or environmental) and its effect on the processes of development than to the nature of the lesion itself. This may explain some of the heterogeneity of neuropathologic lesions seen in children with epilepsy, as the clinical phenotype results not only from the lesion or putative insult to the developing CNS, but also from the developmental processes it subsequently affects and disrupts.
A puzzling feature noted in pediatric epilepsy is the finding, in cases of IS, of diffuse and transient suppression of cortical activity in the presence of focal or multifocal lesions (130). This diffuse cortical suppression has not been linked to particular distributions or types of neuropathologic change. Rather, some have suggested that this feature of IS is associated with neocortical immaturity. The pattern of evolution of IS from transient and diffuse suppression of cortical discharge to ultimately focal or generalized seizures may represent a maturation of the malformed cortex (130).
Nosologic Considerations
Understanding of the full clinicopathologic spectrum of CD is currently evolving and reflects a progressive elucidation of the basis of these complex lesions (131–149). It is of interest that, since the time of the original description of “Focal dysplasia of the cerebral cortex in epilepsy” by Taylor et al in 1971 (144), there was, after a lag time that extended to a few years, an explosion of research on these entities—with a corresponding exponential increase in publications on the topic. CD is the most frequent neuropathologic abnormality encountered among surgical specimens studied in large epilepsy surgery centers—including at least one in the Peoples’ Republic of China (150). Sociodemographic features of affected patient populations and determinants of “time to epilepsy surgery” after seizure onset have been extensively investigated and documented (151,152). Cortical malformations have historically been described largely by their gross characteristics (ie, agyria/pachygyria-lissencephaly, hemimegalencephaly/HME, microgyria). As investigators discovered the range of microscopic cortical malformations that produce epilepsy but show no (or relatively mild) gross abnormalities, additional terms such as microdysgenesis (131,139,140), dysplastic cortical architecture (not otherwise specified) (141,142), focal cortical dysplasia (FCD) (143,144), synaptic dysgenesis, and generalized or diffuse cortical dysplasia (145–149) came into use. The nomenclature of CD has evolved through several schema of classification, each intended to provide correlations with the morphology of seizure disorders or to reflect etiologic mechanisms (117–120,133). The term FCD was initially used to specify focal brain lesions in which cytomegalic and dysmorphic neurons were present (133,144). Some investigators prefer to use a traditional classification of migrational disorders into four main groups: (a) agyria/pachygyria-lissencephaly, (b) microgyria-polymicrogyria, (c) dysplastic cortical architecture, and (d) heterotopias (142). Others, to denote the belief that these lesions are related and reflect developmental abnormalities along a continuous spectrum, have chosen to refer to them as neuronal migration disorders (NMDs) (119,136,137) or cerebral or synaptic dysgenesis (147,149). A consensus conference in the early 2000s resulted in a proposal to subclassify CD or grade its severity (in surgical resection specimens) using simple morphologic criteria identifiable by any experienced neuropathologist (153). This has been superceded by a “consensus” position paper originating from an ad hoc panel charged with revising and updating CD nomenclature (to be discussed in the following text).
Development of the Neocortex
The neuropathologic changes seen in infants and children with epilepsy frequently represent the end result(s) of insults to a rapidly developing brain in utero. Several reviews have summarized the historical evolution in our understanding of the immensely complex molecular and neurobiologic processes that occur to create a normally functioning cerebral neocortex (154–159). Neocortical development after neural tube formation can be roughly considered to be the end result of a series of overlapping processes: (a) cell proliferation in the ventricular and subventricular zones (VZ/SVZ), (b) early differentiation of neuroblasts and glioblasts, (c) programmed cell death of neuronal precursors and neurons, (d) migration of neuroblasts (both radially from the VZ/SVZ and tangentially) to form the mature cortical plate, (e) late neuronal migration, (f) organization and maturation of the cortex, and (g) synaptogenesis/synaptic pruning (132,143,159). A discussion of how derangement of these processes contributes to CD is beyond the scope of this review, but can be found elsewhere (160). Most developmental disorders of the brain associated with epilepsy are thought to originate from the perturbation of developmental events after the embryonic period, that is, after 6 weeks’ gestation, when cell proliferation starts along the wall of the neural tube. This generates a group of “matrix cells” (161), or precursor cells for all neuroblasts and glioblasts, forming the VZ/SVZ in the pallium, as well as the ganglionic eminence in the subpallium. Programmed cell death (PCD, apoptosis) is also an essential mechanism in normal brain development, determining the cellular constituents of the CNS. In normal brain development, there is a 25% to 50% overproduction of neuroblasts, many of which eventually undergo physiologic PCD (149). Synapse elimination is intimately intertwined with the remodeling of cortical connections and is a highly dynamic process (162), demonstrated both in vivo and in vitro (163).
As the neocortex develops, the marginal zone, future molecular layer, or cortical layer I is composed largely of Cajal–Retzius cells. They secrete the extracellular glycoprotein Reelin, which is required for the normal inside-out positioning of neurons as they migrate from the ventricular zone along radial glia. Human Cajal–Retzius cells, characterized by the combined expression of Reelin and p73, are transient cells, present from the preplate stage at 8 weeks of gestation, and gradually increase in number (by tangential migration) until they disappear by the end of gestation (164–166). In mice carrying mutations in RELN (Reeler mice) and in disabled-1 (Dab1), as well as in mice carrying double mutations of both very low-density lipoprotein receptor (VLDLR) and apolipoprotein E receptor 2 (ApoER2), normal neuroblast migration in an inside-out fashion is inverted (167). This suggests a role for these genes in the control of cell positioning in the developing CNS and predicts a pattern of cytoarchitectural alteration in patients carrying alterations in the Reelin/lipoprotein receptor/Dab1 pathway, as well as RELN mutations causing lissencephaly (168). LIS1 also appears to have important effects on neuronal migration, and significant interactions with Cajal–Retzius cells (169). The superficial or subpial granular layer (SGL) is a transient cell layer; it appears beneath the pial surface between 13 and 24 weeks’ gestation (111,112,170). Cells in the SGL originate from the basal periolfactory subventricular zone (170–172) and migrate tangentially beneath the pia to cover the neocortical marginal zone. Cells in this layer express interneuron markers such as calretinin, calbindin, and GABA (173). The biologic significance of the SGL, however, remains to be elucidated. PCD may, at least in part, contribute to its elimination (174). The human subplate contains large multipolar neurons. These neurons in the developing cerebrum, though they are transient and most disappear in early postnatal life, are thought to be important in organizing cortical connections in the developing cerebrum and are thought to act as pioneer corticofugal axons (175–181).
Neuropathologic Significance of Cortical Dysplasia
The Pediatric Epilepsy Surgery program at Ronald Reagan-UCLA Medical Center, active for over 30 years, has enabled us to examine several hundred surgically resected specimens from infants and children with intractable seizures, including a spectrum from partial lobectomies to complete and partial (functional) hemispherectomies. The most common morphologic substrate was CD, this being of etiologic importance as a cause of intractable pediatric seizures in a majority of children younger than 3 years of age. The extent of CD neuropathology can sometimes be predicted by high-resolution neuroimaging studies. Such neuroimaging allows for stratification of CD cases into those that show hemimegalencephaly (HME), with diffuse enlargement of the gray and white matter, including thickening of the cortical ribbon, within an entire cerebral hemisphere; hemispheric CD, with multi-focal CD affecting one cerebral hemisphere (though not causing enlargement of that hemisphere); or multilobar, lobar, or focal CD, the latter affecting as few as one gyrus or two adjacent gyri. The magnetic resonance imaging (MRI) findings of HME include an enlarged cerebral hemisphere and markedly thickened gyri, with loss of sulcation, deformity, and enlargement of the ipsilateral ventricle. Palmini or ILAE type IIB CD is also frequently identified by MRI, with focal thickness of the gyrus (gyri) and associated hyperintense T2-weighted signal changes in the adjacent white matter.
In resection specimens from such patients, macroscopic heterotopia and polymicrogyria (PMG) are occasionally seen. Loss of the normal cortex–white matter junction, best appreciated with a Klüver–Barrera or other myelin stain, is a frequent accompaniment and excellent predictor of severe microscopic CD. Many specimens, however, exhibit no striking gross cortical abnormalities even when severe microscopic lesions are eventually found to be present. CD can be further characterized with regard to specific and easily identifiable microscopic abnormalities, including cortical laminar disorganization, frequent single heterotopic white matter neurons, excess neurons in the neocortical molecular layer, marginal glioneuronal heterotopia, white matter neuronal heterotopia, and neuronal cytomegaly with or without associated dysmorphic features of the cytoplasm (the latter often accompanied by cytoskeletal abnormalities and balloon cell change) (133,179). These microscopic features can be used as the basis for a grading system for CD (see the following text).
Cortical laminar disorganization is a defining histopathologic feature of CD. This can often be dramatically highlighted by immunohistochemistry using primary antibodies to NeuN. Cortical disorganization (as well as cytologic abnormalities in individual neurons) can also be highlighted using immunohistochemistry that incorporates primary antibodies to various neurofilament epitopes (125). Although many neurons still reside in the intermediate zone/white matter in the last trimester of pregnancy and even into postnatal life (180,181), the phenomenon of single heterotopic neurons in the white matter is accentuated in CD (135,149) and has been demonstrated using morphometric techniques (182). Injury to the radial glial fibers may lead to a stranding of the migrating neuroblasts within the white matter, where they further differentiate into mature neurons (149). Alternatively, overproduction of neurons late in neurogenesis may lead to crowding of migrating neurons toward the cortical surface (183). Morphometric analysis has demonstrated a statistically significant increase in the number of neurons within the molecular layer of the cortex in epileptic patients (140); this is considered to be evidence of a slight maldevelopment of the neocortex, microdysgenesis (125,130,131). Persistence of the superficial granular layer (SGL) has been seen in association with many cortical malformations (133). Marginal glioneuronal heterotopia consists of excrescences of disorganized neuroglial tissue extending from the pial surface into the subarachnoid space. These lesions may be associated with a failure of the glia limitans. White matter neuronal heterotopia consists of disorganized masses of neurons in the white matter that usually occur in a periventricular position with a nodular morphology, although rare instances of laminar subcortical bands of heterotopic gray matter (band heterotopia) have been known to produce the appearance of a double cortex. These lesions may be associated with injury to a group of radial glia, leading to failure of a group of neuroblasts to migrate normally (149). Alternatively, a defect in genes controlling neuroblast proliferation, neuroglial interactions, and PCD has been suggested as being of etiologic importance (142). PMG denotes small, meandering gyri, often with bridging of the sulci by fusion of the molecular layers. It consists of two histologic types. Four-layered PMG is most frequently considered to result from a destructive lesion occurring at approximately 20 to 24 weeks of gestation, and an unlayered form is thought to result from an insult earlier in brain development (at approximately 13–16 weeks) (183).
Neuronal cytomegaly denotes enlarged neurons, some of which may also be dysmorphic; these were first described in association with seizure-producing focal cortical malformations by Taylor et al (144). Nerve cell hypertrophy was convincingly shown using quantitative histochemistry in a case of HME (184). The differentiation of cytomegalic vs. dysmorphic neurons is of importance in assigning a severity grade (using the Palmini or ILAE grading scheme) to a given lesion. Neurons that show enlargement and dysmorphism of their cell bodies are, in association with cortical architectural disorganization, typical of ILAE type IIA CD, whereas corticectomies that also contain “balloon cells” are characteristic of ILAE type IIB CD. (ILAE type IIB CD largely corresponds to what has also been described as Taylor-type focal cortical dysplasia, or T-FCD.) Dysmorphic neurons bear an extremely complex dendritic arborization as well as an abundance of perisomatic synapses and a paucity of axosomatic synapses (185,186). Increased neuronal size has been associated with an increased DNA and RNA content, as well as increased nuclear and nucleolar volume suggestive of heteroploidy (187). Argyrophilic, neurofibrillary-like tangles and cytoplasmic vacuoles have been demonstrated within many such neurons (188), as has the existence of paracrystalline intracytoplasmic structures visible on ultrastructural examination (189). These neurofibrillary-like cytoplasmic inclusions differ from the neurofibrillary tangles of Alzheimer disease in that they do not contain paired helical filaments (190).
Balloon cells, showing similarity to gemistocytic astrocytes, have eccentric nuclei and ballooned, glassy, or opalescent eosinophilic cytoplasm. They often demonstrate binucleation or dysmorphic nuclei, sometimes showing bridges of nucleoplasm between two separate islands of nuclear material within a cell. Nuclear inclusions may be present (125). They may cluster at the cortex–white matter junction or be abundant within subcortical white matter. Frequently, they are admixed with dysmorphic and enlarged neuronal cell bodies. Ultrastructurally, they are packed with filaments ranging in size from 400 to 600 nm in length and 30 nm in thickness, interspersed with non-membrane-bound, electron-dense, helical structures (191). Vinters et al (188) and others have demonstrated dual staining of many cells in the dysplastic cortex (including some balloon cells) with antibodies to both neuronal and glial markers, implying either a failure of the cells to commit to a specific phenotype or dedifferentiation (125,133). The resemblance of balloon cells to cells found within the cortical tubers of TSC has suggested the possibility that cases of CD harboring balloon cell change may represent a forme fruste of TSC (125,135,191,192). However, substantial genetic arguments have been made counter to this view—that is, mutations and polymorphisms in the TSC genes (see later text) are not found in specimens from CD patients (193). However, it is being recognized increasingly that CD may result from mutations in genes coding for other proteins such as PTEN, DEPDC5, P13K/AKT, and so on, that feed into the mTOR pathways (194,195). Inflammation may be detected in a resected cortex originating with epileptic patients—especially if one considers microglial and astrocytic activation as a component of neuroinflammation (196). Caution must obviously be exercised in deciding whether neuroinflammation contributes to development of the epileptogenic lesion, or is a consequence of longstanding seizures. Immunohistochemical application of neuronal “layer” markers has been suggested to be of value in making the diagnosis of CD, especially ILAE type I CD; however, it has thus far not gained widespread use (197). One widely experienced group has proposed that apoptosis and neurodegeneration are common events in CD tissue (198).
Coming by way of follow-up to other systems of CD classification proposed by Mischel et al (133) and Palmini et al (153), some years ago an ad hoc task force of the International League Against Epilepsy (ILAE) was charged with the job of developing a “consensus classification” for the neuropathologic features of CD/FCD and HS (199,200). After much discussion and substantial debate, this group stratified CD into three main types: Type I, characterized by dyslamination of the neocortex, which may be more prominent radially or tangentially, but lacking neuronal cytologic abnormalities; Type II, subdivided further into type IIa with cortical dyslamination and dysmorphic neurons (Figures 5.5–5.8) and type IIb, with (in addition to neuronal dyslamination) the presence of balloon cells (see the previous text) (Figures 5.9–5.11). The ILAE Task Force went on to define FCD type III which, by definition, describes CD in the presence of “another [potentially] epileptogenic lesion.” The “other lesions” include hippocampal sclerosis (HS; type IIIa), epilepsy-associated tumors (eg, dysembryoplastic neuroepithelial tumor or DNET, ganglioglioma; type IIIb), vascular malformations (type IIIc), or epileptogenic lesions acquired early in life (type IIId). This classification scheme has been examined in terms of its usefulness for inter- and intraobserver reliability, and appears to be quite a sound and reproducible system (201).
FIGURE 5.5 Histologic sections (stained with hematoxylin & eosin) from two regions of the cortex originating in a corticectomy specimen from a child with intractable seizures. Panel A shows a relatively normal cerebral cortex, with negligible abnormalities of cortical organization or neuronal cytomorphology. Panel B (micrograph magnification is identical to that shown in Panel A) highlights a region with cortical disorganization, as well as enlarged and dysmorphic neurons. Changes are typical of CD, ILAE type IIa.
FIGURE 5.6 Histologic section (same resection specimen as shown in Figure 5.5, but stained with anti-NeuN) shows a striking contrast between two adjacent gyri. The gyrus at the right is relatively normal, whereas the gyrus at the left shows neuronal dyslamination and enlarged neuronal cell bodies (which demonstrated dysmorphic features at higher magnification, see Figure 5.7). Three blue “dots” are for specimen orientation prior to morphometric evaluation.
FIGURE 5.7 Anti-NeuN immuostained sections from corticectomy originating in a child with intractable seizures (same subject as shown in Figures 5.5 and 5.6). Panel A is from a region of relatively normal neocortex, Panel B from a focus of CD, ILAE type IIa. Both panels are shown at identidal magnification. Note the striking enlargement and atypia of neurons illustrated in panel B, which also demonstrates neuronal disorganization. “Balloon cells” were not seen in any region of the specimen despite multifocal CD.
FIGURE 5.8 Astrocytic gliosis in CD ILAE type IIa. Micrographs are from sections immunostained with anti-GFAP. Panel A shows a typical region of the cortex, shown at low magnification. Panel B shows a magnified view of large astrocytes with variably prominent cell bodies. Whether the gliosis is part of the neuropathologic change of CD, or a “response” to chronic seizures and their treatment, is unclear in a given case.
FIGURE 5.9 CD, ILAE type/grade IIb, surgical specimen, section stained with H&E. Panel A shows a field with dysmorphic neurons, many showing abnormal polarity (arrows). Panel B shows balloon cells (arrow) distributed among the dysmorphic neurons.
FIGURE 5.10 CD, ILAE IIb, surgical specimen, from sections stained with H&E. Both panels show dysmorphic neurons distributed “randomly” and in a disordered fashion, among many balloon cells (arrows, panel A). Arrows in Panel B highlight dysmorphic neurons.
FIGURE 5.11 CD, ILAE IIb, surgical specimen, sections immunostained with anti-GFAP. Unlike the reactive astrocytes shown in Figure 5.8, many of the dysmorphic and atypical cells show GFAP immunoreactivity, shown at low magnification (panel A) and higher magnification (panel B).
Pathogenesis of Cortical Dysplasia
Although it is accepted that cortical dysplasia involves abnormal cerebral cortical development, it is unclear when the causal “lesions” occur and how the cortical abnormality produces seizures. Most MCD classification systems are based on speculating when the first developmental steps involving cell proliferation, neuronal migration, and cortical organization become deranged to produce the malformed cortex. Previously, the presumed mechanisms of CD pathogenesis were thought to reflect defects in neuronal migration, as one explanation for subcortical heterotopic neurons, and altered periventricular neuroglial differentiation to account for cytomegalic and dysmorphic neurons and balloon cells (202–204). Although such mechanisms appear to be operant in genetic forms of CD, clinicopathologic and electrophysiologic investigations (using corticectomy specimens) have challenged this singular interpretation (118,122,123,205,206).
In several studies, the UCLA group has found evidence that surgically treated CD seems to involve retention of cells of the human pre- and subplate, along with an overproliferation of cortical neurons. Prenatal human subplate cells have morphologic similarities to dysmorphic neurons found in postnatal CD tissue (206). Normal human subplate contains large multipolar neurons similar to cytomegalic neurons, and polymorphous and fusiform neurons with thick primary dendrites along with inverted pyramidal-shaped neurons, a feature noted in dysmorphic CD neurons (125,191). Most human subplate cells degenerate in the 4 to 6 weeks before birth (175,207), which coincides with increasing definition of the gray–white matter junction and secondary gyral folding (208). Near the end of normal neurogenesis, periventricular radial glial cells attach themselves to the tailing processes of the last produced cortical pyramidal neurons and migrate toward the cortex, where they detach and eventually transform into protoplasmic astrocytes (209–211). This may explain why balloon cells in CD tissue have some characteristics similar to those of radial glia and may express stem cell markers (212–214).
Our group has proposed that CD pathogenesis probably involves partial failure of the later phases of neocortical formation. As a consequence, subplate and radial glial degeneration and transformation would be reduced or prevented, giving the appearance of abnormal dysmorphic cells in postnatal CD tissue. A failure of late cortical maturation could explain the presence of abnormally thickened gyri with indistinct cortical gray–white matter junctions in the MRI scans of CD patients (122). The precise timing of these events during cortical development would explain the different forms of CD identified by MRI as well as the severity of CD assessed by histopathology. Developmental alterations during the late second or early third trimester would account for severe CD, such as hemimegalencephaly, whereas events occurring closer to birth might explain milder forms of CD. In addition, there appears to be an overproduction of neurons in the later phases of cortical development. Cerebral hemispheric volumes assessed by MRI were normal or increased in the case of hemimegalencephaly, and cortical thickness was the same or slightly increased (124, 183). Furthermore, neuronal densities were increased in the upper gray matter, molecular layer, and subcortical white matter. The location of excess neurons would be consistent with the idea that this process occurred in later periventricular cell cycles (ie, ones occurring toward the end of neurogenesis). Thus, heterotopic subcortical white matter neurons are likely the result of excessive late-generated pyramidal neurons “attempting” to migrate toward the already overly crowded cortical ribbon, in combination with residual prenatal subplate neurons that failed to degenerate before birth.
It has recently been proposed that viral infection of the developing brain (especially by human papillomavirus/HPV) may be an important factor in the pathogenesis of CD, predominantly CD ILAE type IIb; however, some laboratories have failed to reproduce this observation or have suggested it may represent an immunohistochemical staining artefact (215–217).
Cortical Tubers of the Tuberous Sclerosis Complex (TSC)
Tuberous sclerosis complex (TSC) is an autosomal dominant, multisystem disorder in which the CNS, eyes, kidneys, skin, and heart are most commonly affected by malformative, hamartomatous, or neoplastic lesions (218–223). It has an incidence of 1:9,400 to 10,000 births (224,225); however, accurate estimates are difficult to ascertain, and a high rate of spontaneous TSC gene mutations has been described (218,224). The clinical presentation of an individual with TSC may be with infantile spasms, autism, a non-ISS seizure disorder, or mental retardation. Approximately 85% of TSC patients who come to medical attention have experienced a seizure at some time. Because genetic analysis to confirm the diagnosis of TSC remains unavailable to most physicians, diagnostic criteria for TSC have been enunciated (219,225). In the 1998 criteria, some clinical features previously thought to be pathognomonic for TSC were considered less specific, whereas clinical or radiographic features of the disease were subdivided into major and minor categories, based upon their apparent degree of specificity for TSC. Thus, no single lesion defines the disease.
Neuropathology of Cortical Tubers as a Cause of Seizures. Individuals with TSC may rarely present with HME. However, the characteristic brain abnormalities of TSC include neocortical tubers (Figure 5.12), subependymal nodules (SENs), and subependymal giant cell astrocytomas (SEGAs). Cortical tubers are often associated with ISS and intractable epilepsy in children. Tuber “count,” as assessed by neuroimaging, appears to be a robust biomarker correlating with neurologic severity of TSC in a given patient (226). “Tuberectomy” is now an accepted form of treatment for intractable seizures in affected children (219,227). The lesions manifest as enlarged, sometimes mushroom-shaped gyri, in which the cortex–white matter junction has become blurred, resembling sporadic, severe cortical dysplasia ILAE type IIb (125,133). Histologically, the lesions show disorganized neocortex, with a variety of dysmorphic, markedly enlarged neurons and bizarre gemistocytic astrocyte-like “balloon cells” having eccentric nuclei containing relatively coarse chromatin and glassy, eosinophilic cytoplasm (Figure 5.12). Balloon cells seen in TSC are almost identical to those seen in severe cortical dysplasia (CD). Tubers may show prominent calcification, probably more commonly than sporadic ILAE type IIb CD. Giant cells within TSC tubers show halos of synaptophysin immunoreactivity around neurons, resembling those noted in gangliogliomas, as well as strong immunostaining with antibodies to the microtubule-associated protein 2 (MAP-2) (230). Alpha B-crystallin, a member of the heat shock protein family of peptides, is found within dysgenetic cells of tubers, and within both SEGAs and SENs (231). Dysmorphic cytomegalic neurons express high levels of tuberin, as do individual cells within SEGAs and SENs. Tuberin appears to be present in most neuronal populations of the CNS from at least 20 weeks’ gestation, with an apparent upregulation of its expression after 40 weeks of gestation (222). Hamartin was found, albeit with a weaker signal, in the same cell types during CNS development.
FIGURE 5.12 Tuber of tuberous sclerosis complex. All panels are micrographs from the same specimen, examined using different stains. Panel A (from a hematoxylin and eosin–stained section) shows features akin to those seen in ILAE type IIB cortical dysplasia. Note a markedly disorganized, crowded collection of dysmorphic neurons and balloon-like cells. Panel B is from a section labeled with a digoxigenin-labeled probe for the TSC2 gene product. Note the differential expression of the gene in various cells, but especially prominent expression within cells of neuronal phenotype (228). Panel C is from a section stained (immunoperoxidase technique) with primary antibodies to the TSC1 gene product, hamartin. Note prominent immunoreactivity of clusters of cells (with uncertain phenotype, arrows) and perivascular cells (arrowheads). This tuber originated from a patient in whom it was unknown whether a mutation was present in the TSC1 or TSC2 gene (229).
Although architectural disarray is a defining feature of a tuber, cellularity of a lesion may be extremely variable. When high cell density is noted, a tuber may resemble a ganglioglioma (222,232,233). Although the proliferative potential of these lesions appears low, as judged by immunohistochemistry for Ki-67, other markers of cellular proliferation (eg, collapsin response mediator protein 4/CRMP4, doublecortin/DCX) are expressed within giant cells of cortical tubers and SEGAs, suggesting that they may represent newly generated cells that have migrated into tubers from the SVZ (232,233).
Our current understanding of the molecular pathogenesis of TSC represents a triumph of multidisciplinary, multicenter collaborations focused on characterizing the complex gene defects that cause this disorder (234–238). TSC results from mutations in one of two nonhomologous tumor suppressor genes, TSC1 (237) on chromosome 9 (9q34) encoding a 130-kDa protein, hamartin, and TSC2 (234) on chromosome 16 (16p13.3) encoding a 200-kDa protein, tuberin. About half of TSC families show linkage to each of these two genes. TSC1 mutations, accounting for a minority of mutations identified, are slightly less common in sporadic TSC patients, but more common in familial cases (13%–50%) (237). Putative functions of both TSC1 and TSC2 gene products have been studied using mostly Drosophila and rodent models of TSC, but also using human tissues. This work initially suggested that both TSC1 and TSC2 gene products had growth suppressor properties (239–241). Significant interactions of the TSC gene products with each other, and with intracellular signaling pathways (especially components of the mTOR pathway—which are regulated by tuberin and hamartin), have been implicated in disease pathogenesis (217–219).
Hamartin may also interact with the ERM (ezrin, radixin, and moesin) family of actin-binding proteins to activate small GTPases of the Rho subfamily (Rho GTPases) (242,243). Rho GTPases are important regulators of the actin cytoskeleton and may be involved in neuronal developmental processes including migration, establishment of polarity, synapse formation, and so on (219,220). ERM proteins belong to the band-4.1 superfamily of membrane–cytoskeleton linking proteins (243–245), which are thought to function in multiple different fashions according to their interaction with various membrane proteins, Ras superfamily GTPases, and the actin cytoskeleton; they may be involved in the formation of microvilli, cell–cell adhesion, maintenance of cell shape, and motility (219,220,245). Tuberin and hamartin may be attached to the membrane–cytoskeletal cortex through activated ERM proteins (246,247). Evidence suggests that ERM proteins function at a position upstream and downstream of Rho GTPases to regulate cellular adhesion and motility (248,249). ERM proteins (ezrin and moesin) are expressed in germinal matrix cells, migrating cells, and radial glial fibers in the developing human brain (246), correlating with RhoA expression in proliferating and migrating cells in the developing rat brain (250). Dysfunction of tuberin and hamartin may perturb communication between ERM proteins and Rho GTPase to cause abnormal neuronal migration, polarity, and morphology, resulting in the formation of dysplastic cortex. Hamartin and tuberin are coexpressed within a population of abnormal neuroglial cells (229), and both TSC gene products and ERM proteins are also coexpressed within a subpopulation of abnormal neuroglial cells in TSC tubers (244,229). Abnormalities of radial glia have also been implicated in the pathogenesis of brain lesions of TSC (251).
Tuberin contains a conserved 163-amino acid carboxy-terminal region that exhibits sequence homology to the catalytic domain of a GTPase-activating protein (GAP) for the small molecular weight GTPase Rap1 and for Rab5 (219,220,252,253). TSC2 mRNA was found to be widely expressed in various cell types throughout the body, including epithelia, lymphocytes, and endocrine organs; within the CNS, it was prominently and selectively expressed within neurons (228). Widespread expression of the TSC2 gene within a developing and adult nervous system was noted in another study using various techniques (254). A study in mice showed that tuberin localized to the perinuclear region of cerebellar Purkinje cells, whereas hamartin was noted to distribute along neuronal or astrocytic processes. TSC2 mRNA and tuberin were found in abundance in many CNS cell types, including neurons and ependymal cells (255,256).
TSC1 and TSC2 gene products colocalize within tubers (and sometimes within individual dysmorphic cells) of patients with TSC (229). Tissue culture experiments show that both hamartin and tuberin interact with the G2/M cyclin-dependent kinase CDK1 (257). Hamartin and tuberin may have separable and distinct functions in mammalian cell cycle regulation (258). Tsc1 and Tsc2, Drosophila homologs of TSC1 and TSC2, function together in vivo to negatively regulate cell size, cell proliferation, and organ size in the insulin signaling pathway (PI3Kinase-Akt/PKB-mTOR-S6K-S6) at a position downstream of dAkt (Drosophila Akt) and upstream of dS6k (Drosophila S6 kinase) (259). This has been shown in surgically resected TSC tubers by means of quantitative immunohistochemical evaluation using tissue microarray methodology (260); constitutive activation of S6K has been observed in TSC tubers but not in focal cortical dysplasia of Taylor type (T-FCD or CD ILAE type IIb) (260,261). In recent years, animal models that recapitulate many of the phenotypic features of TSC—including seizures and neuropathologic abnormalities including cortical disarray and neuronal cytomegaly—have been developed (262). Deep sequencing studies of TSC patient tubers (surgically resected) have shown that germline heterozygous mutations (in TSC1, TSC2) could be identified in over 80% of tubers. However, secondary mutations (in TSC2) were found in six tuber samples from one individual, and this “second hit mutation” was found widely distributed throughout the cortex of that subject. However, overall this study concluded that second hit mutations (in TSC1, TSC2, and KRAS) were very rare events in TSC tubers (263). Genetic analysis of hemimegalencephaly and CD specimens has further shown that various mutations in the mammalian target of Rapamycin (mTOR) pathway may cause these disorders (264). Thus, meticulous analysis of the mTOR pathway components has become a major theme of research in both CD and TSC.
STURGE–WEBER–DIMITRI (SWD) SYNDROME/ENCEPHALOTRIGEMINAL ANGIOMATOSIS
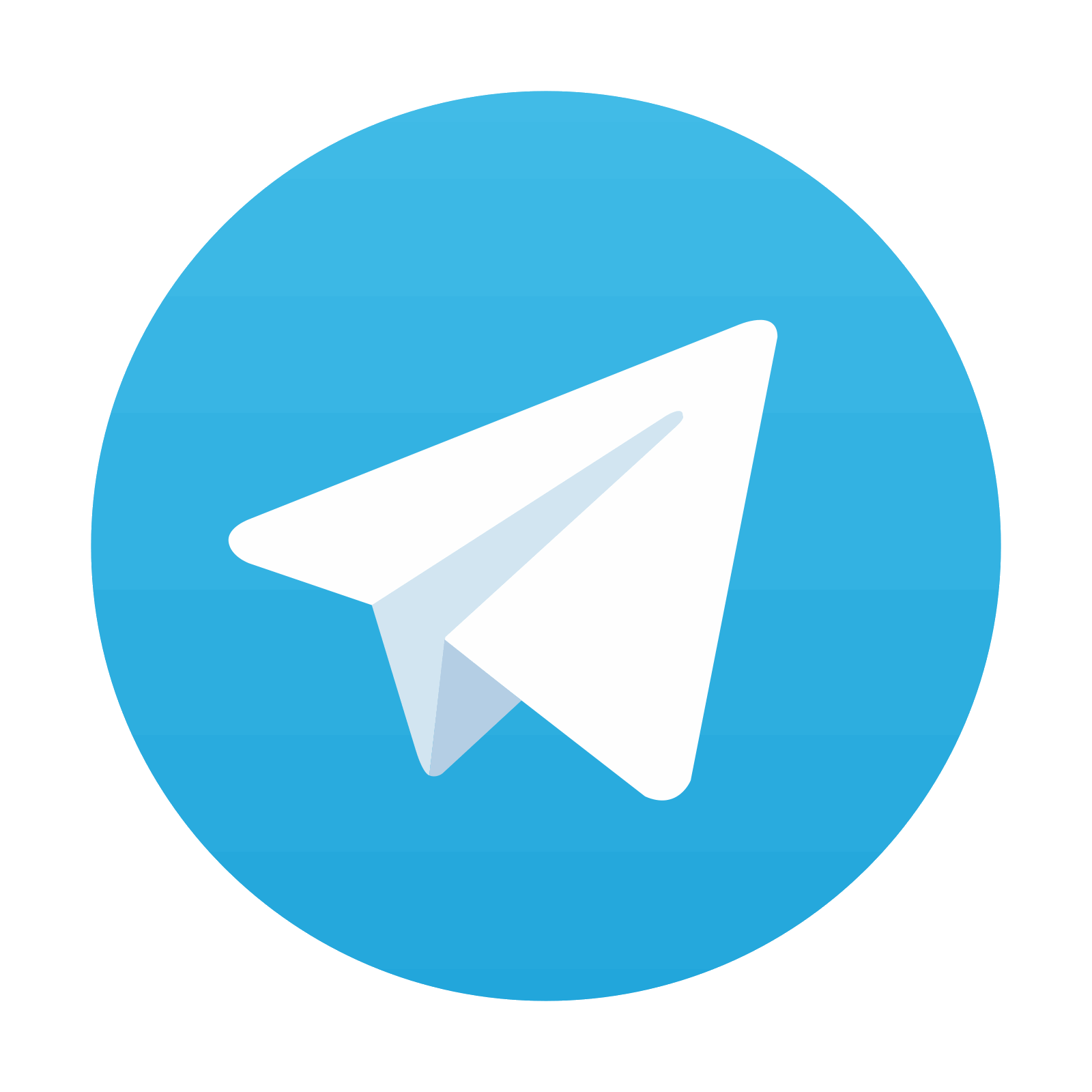
Stay updated, free articles. Join our Telegram channel
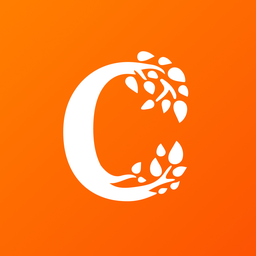
Full access? Get Clinical Tree
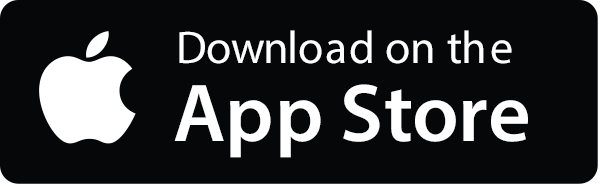
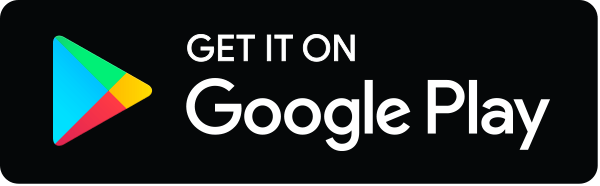