There are tiny, puny infants with great vitality. Their movements are untiring and their crying lusty, for their organs are quite capable of performing their allotted functions. These infants will live, for although their weight is inferior … their sojourn in the womb was longer. Pierre Budin, The Nursling
As indicated in the above quotation, a newborn infant’s problems and prognosis are determined by birth weight and gestational age. The designation low birth weight (LBW) is applied to all infants weighing less than 2500 g at birth, regardless of the duration of gestation. Subsequently, the terms very low birth weight (VLBW) and extremely low birth weight (ELBW) have been used to categorize those infants with birth weights less than 1500 g and 1000 g, respectively. The classification of infants as preterm is reserved for those having completed less than 37 weeks of pregnancy, whereas term gestation refers to those infants delivered between 37 and 41 completed weeks of pregnancy, and postterm indicates birth after or equal to 42 completed weeks of pregnancy. The proportion of LBW infants who are preterm versus those with abnormal intrauterine growth varies around the world. In developed countries, the majority of LBW babies are premature, whereas in developing countries, the major contributor to the LBW rate is growth-restricted term infants. As the standard of living improves in developing countries, there is a shift toward the pattern of developed nations with regard to LBW infants.
Infants are classified as small for gestational age (SGA) if their birth weight is below the 10th percentile and large for gestational age (LGA) if their birth weight is above the 90th percentile ( Fig. 5-1 ) . Intrauterine growth restriction (IUGR) describes failure of a fetus to reach its genetic growth potential. The limitations and complexity of these concepts are considered further in this chapter.

Determinants of Fetal Growth
Normal fetal growth requires contributions from the mother, the placenta, and the fetus. Numerous maternal metabolic adjustments are made during pregnancy, the unifying goal of which appears to be provision of an uninterrupted supply of nutrients to the developing fetus. Foremost among these are adjustments in carbohydrate metabolism. Mild fasting hypoglycemia and postprandial hyperglycemia associated with an increased basal insulin level and relative insulin resistance characterize the normal pregnancy. Maternal glucose use is attenuated, with ketones and free fatty acids increasingly serving as fuels for maternal tissues. The mechanisms for these alterations are not entirely clear. However, the effect is the provision of a continuous supply of glucose, the primary source of fetal oxidative metabolism, to the fetus, particularly during periods of maternal fasting. During relatively extended periods of fasting, the fetus uses ketones to serve his or her energy and synthetic needs as well. Maternal serum levels of lipids increase during gestation. In midpregnancy, fat is stored for fetal use during late pregnancy when demands increase. These, and a variety of other adjustments, are so effective in supplying the fetus with required nutrients that only with severe maternal malnutrition (e.g., wartime famine), and only then if starvation occurs during the third trimester, is birthweight reduced. If starvation occurs in the first trimester, the placenta grows larger to compensate for the reduced energy supply to the fetus, and if nutrition is restored in the second and third trimester, birth weight is actually increased. The human placenta, in addition to its role of transmitting nutrients from mother to fetus, functions as an incredibly active endocrine organ, producing an array of hormones unsurpassed in the animal kingdom. Among those products with direct growth-promoting action are growth factors and human placental lactogen (HPL), also known as chorionic somatomammotropin. HPL is produced by the syncytiotrophoblast cells of the placenta. Its growth-promoting effects are mediated by the stimulation of fetal insulin-like growth factor (IGF) production and increasing nutrient availability. The previously mentioned elevation of maternal serum lipids plays a role here as well. The expression of the HPL gene is regulated, in part, by apoprotein A1, the major protein component of high-density lipoprotein. The fetus plays a role in his own growth by producing a variety of polypeptide IGF molecules and modulating binding proteins. These substances are produced by a spectrum of fetal tissues, with site, timing, and control of expression varying with each IGF. The biologic and clinical significance of serum levels of the various growth factors and binding proteins in the fetus and newborn is an area of active research.
The Concept of Intrauterine Growth Restriction
The growth trajectory of any individual fetus results from the combined effects of its genetic programming and the growth support it receives from its mother. Clearly, the genetic growth potential of a fetus is determined by the contribution of the mother and the father and how they interact. This does not happen on the basis of equality of parental contribution. For example, approximately 30 genes are known to be active only if they are acquired from the mother, or from the father. This phenomenon is known as “genomic imprinting.” Paternal imprinting tends to encourage fetal growth, whereas maternal imprinting tends to restrict fetal growth. Although maternal and paternal genes have an approximately equal contribution to adult height and weight, the height and weight of the mother contribute more than 90% of the influence on birth weight compared with the father. This was dramatically illustrated in the 1930s by Walton and Hammond who crossed very large Shire horses with very small Shetland ponies, and demonstrated that birth weight was appropriate to the mother’s size, whereas adult size was intermediate (although foals born to the small mothers never quite reached the same size as those born to large mothers, showing that severe intrauterine growth restriction can result in reduced adult size). A similar phenomenon has been demonstrated in humans, such that the birth weight of babies born from ovum donation is appropriate to the size of the birth mother rather than that of the genetic mother. Although a convenient definition of intrauterine growth restriction is failure of the fetus to reach its genetic growth potential, in practice this simple definition is not comprehensive because the genetic potential is not invariable, but is modifiable according to nutrient supply. In addition, some babies’ genetic growth potential is abnormal in the first place, for example, a high proportion of babies with Down syndrome exhibit many of the characteristics of intrauterine growth restriction.
So how should we decide whether a fetus is growth restricted? Ideally, we should base our classification on functional measures, the most obvious of which is the risk of stillbirth or neonatal death. Other typical indicators of inadequate growth status include an inability to cope with the hypoxia of labor, resulting in fetal acidosis and neonatal depression, the passage of meconium during labor, and neonatal dysfunction such as hypoglycemia and hypothermia. In the longer term, catch up growth may be incomplete, resulting in reduced adult size. Alternatively, catch-up growth can be excessive, leading to adult obesity, hypertension, and insulin resistance with an increased risk of diabetes. The concept of intrauterine growth restriction leading to long-term sequelae has led to the “fetal origins of adult disease” hypothesis proposed by Barker and his colleagues.
Intrauterine growth restriction does not relate directly to percentile birth weight. Although babies that are small for gestational age are at increased risk of the dysfunction typical of intrauterine growth restriction, many of them will be normal small babies. There is no clear threshold of percentile birth weight below which the risk of dysfunction increases; instead the risk rises steadily as the percentile birth weight falls. One approach that can improve the correlation of percentile birth weight with function is that of using “customized birthweight percentiles,” in which the percentile birth weight of a particular baby is adjusted to take into account the mother’s height, weight, racial origin, and other relevant factors. However, these variations can be pathologic as well as physiologic. For example, severely underweight or overweight mothers are more likely to have babies that are born preterm or become macrosomic (with all its attendant problems), and it would be inappropriate to correct percentiles to this extent. Mothers from some racial groups are more likely to have small babies that are twice as likely to be stillborn, and again, correction for this would clearly be inappropriate. It has also been argued that using percentile charts based on estimated fetal weights of fetuses growing normally instead of percentiles based on actual birth weights may give a better indication of the incidence and role of fetal growth restriction on neonatal disease. However, this approach is limited by the difficulty to obtain accurate measurements to establish growth charts based on estimated fetal weights.
Perhaps the most appropriate way of defining intrauterine growth restriction is using serial ultrasound measurements of fetal size. Scans from 22 weeks’ gestation onward can be used to establish the “normal” growth velocity for an individual fetus, and a subsequent decline in this growth trajectory clearly fulfills the requirements for the definition of growth restriction. Prospective studies have shown that such measurements are at least as good a predictor of intrapartum dysfunction as percentile birth weight (and the latter cannot be known accurately before birth anyway). Some babies initially growing on the 90th percentile show slowing of growth typical of growth restriction, and sustain perinatal problems despite having a birth weight within the “normal” range. This phenomenon is sometimes called normal weight growth restriction .
Pattern of Fetal Growth
With the use of anthropometric measurements, including fetal weight, length, and head circumference, fetal growth standards have been determined for different reference populations from various locations. From these data, it is apparent that there are variations in “normal” weight at any given gestational age from one locale to another. This variation is related to a number of factors including sex, race, socioeconomic class, and even altitude. Of course, the key issue here is what is meant by “normal.” For example, babies born at high altitude are, on average, smaller than those born at sea level. Thus, when percentile birth weights for babies born at altitude are constructed, the 10th percentile (commonly used as the boundary between “normal” and “abnormal”) will be at a lower birth weight than that for babies born at sea level. For example, babies that are on the 11th percentile for altitude might be on the 8th percentile for sea level and would be categorized as “normal weight” for altitude but as “below normal weight” for sea level. However, we also know that babies born at altitude have a higher stillbirth rate. Should we also regard it as “normal” for more babies to be stillborn? It is vital to remember that, ideally, the purpose of customized percentiles is to correct for variations in birth weight due to physiologic variations in the mother and her environment that are associated with variations in birth weight but not associated with a worse outcome.
For example, the Colorado data, presented by Lubchenco et al. in the 1960s, summarized standards of intrauterine growth for white (55%), black (15%), and Hispanic (30%) newborns born between 1948 and 1961 in the vicinity of Denver. The graphic display of this relationship provides a useful and simple method for determining the appropriateness of growth with respect to gestational age and with respect to the local population. What such standards cannot do, however, is indicate whether the population as a whole (e.g., Hispanics compared with whites) is disadvantaged. This can only be determined by looking at perinatal mortality and morbidity rates. Ideally, to be most useful, equivalent measures of weight for gestational age between two different populations should be based on equivalent risk of poor outcome, rather than simply the population distribution of birth weight.
Ten years after the Colorado data were published, Brenner et al. presented fetal weight curves based on more than 30,000 pregnancies, including terminations of pregnancy and miscarriages as well as spontaneous births, with correction factors for parity, race, and sex. Although these and other such curves differ in details, all demonstrate nearly linear growth between 20 and 38 weeks of gestation, with slowing thereafter. Using such nomograms, one can plot fetal growth and detect a decline in growth velocity, indicating growth restriction. Although the use of customized reference ranges for normal fetal growth can highlight intrauterine growth restriction by making a decline in growth more obvious, it must be emphasized that failing growth indicates increased risk to the fetus—irrespective of the percentile birth weight that the baby is currently on. So, for example, a fall in relative intrauterine size from the 80th percentile to the 40th percentile can be as significant as a fall from the 10th percentile to the 5th percentile. Thus, many workers consider that the best descriptor of intrauterine growth restriction is “a fall in growth velocity,” rather than being on, or falling below, a particular percentile.
Antenatal Assessment of Intrauterine Growth
Clinical Assessment of Gestational Age
Optimal management of the pregnant woman and her fetus is highly dependent on an accurate knowledge of the gestational age of the fetus. Knowledge of the gestational age is important for interpretation of common tests (e.g., nuchal translucency screening for Down syndrome), scheduling invasive procedures (e.g., amniocentesis), planning the delivery of high-risk fetuses (e.g., assessing the risk of respiratory distress syndrome [RDS]), and assessing fetal size for gestational age. Determination of the expected date of delivery (due date) can be made with varying degrees of certainty by history of menstrual cycles, physical examination of the pregnant woman, and a variety of clinical obstetrical milestones. Regular ultrasound examination of the developing fetus is the most accurate (unless of course the date of conception is precisely known, as with in vitro fertilization and associated techniques).
The average duration of pregnancy is 280 days from the first day of the last menstrual period in white mothers. Because conception occurs on average on day 14 of the menstrual cycle, the true duration of pregnancy is 266 days. There is now good evidence that gestational age varies in different racial groups, being 5 to 7 days shorter, for example, in South Asians and black Africans. Between 22 and 34 weeks’ gestation, there is a reasonable correlation between the age of the normally growing, single fetus in weeks and the height of the uterine fundus in centimeters when measured as the distance over the abdominal wall from the upper border of the symphysis pubis to the top of the fundus. This can be used for screening, but it is very unreliable in the 30% or more of western populations who are obese. For this reason, the efficiency of screening for growth restriction is particularly poor in obese women (obesity also makes ultrasound measurements more difficult and therefore less reliable). The size of the uterus changes more slowly in late pregnancy because as the fetus grows, the relative proportion of amniotic fluid decreases. Although physical examination estimates of gestational age have a standard deviation of plus or minus 2 weeks in the first trimester, this extends to 4 weeks in the second trimester, and 6 weeks in the third trimester.
Assessment of Gestational Age
Forty percent of pregnant women have an uncertain last menstrual period (20% have no idea of the date), making accurate estimation of gestational age by history difficult at best. Since the 1970s, antenatal determination of gestational age using serial ultrasound studies of the fetus has become universal in developed countries. The type of ultrasound, the parameters measured, and the accuracy of the study vary with the progression of pregnancy. In the first trimester, although it is possible to visualize the early gestational sac as early as 5 weeks, the optimal time for scanning is between 7 and 9 weeks, with measurement of the crown-rump length using a high-resolution vaginal probe. Routine ultrasound screens for dating, however, are usually carried out during the second trimester, typically between 11 and 14 weeks’ gestation, which is when the nuchal translucency assessment of Down syndrome risk is most accurate. It is also usual to perform a further scan at 20 to 22 weeks’ gestational age, for comprehensive fetal anomaly screening. Measurements at this stage of pregnancy are less reliable for assessing gestational age, because to estimate gestational age from the size of the fetus, one has to assume that the fetus is of average size. Some babies will be small and some will be large for any particular gestational age. When the baby is growing rapidly in the first trimester, the change in size from 1 week to the next is substantial; therefore, the standard deviation of likely gestational age is small. Typically, 2 standard deviations are only 3 to 4 days different from the mean. It can be assumed that the fetus is likely to be of the average gestational age for a particular size, plus or minus 3 to 4 days. Fetuses smaller than 2 standard deviations below the average size for gestational age are likely to be heading for demise. However, the normal range of size increases as gestation advances, and accuracy of dating in the second trimester is generally no better than plus or minus 7 days. Ultrasound measures size and not gestational age. A very small baby on ultrasound examination may be just that, rather than having an incorrect gestational age assignment and, similarly, a very large baby may be macrosomic. The most commonly used parameters for determining estimated gestational age during the second trimester are head circumference, biparietal diameter, abdominal circumference, and femur length. Each of these measurements has its own advantages and disadvantages, but all have in common a decreasing level of accuracy with increasing gestational age, particularly after 20 weeks, because of increasing normal biological variation with advancing gestation. To enhance the accuracy of the assessment, a composite fetal size based on the average of these four measurements is used and incorporated into the software of the ultrasound machine for instantaneous calculations. Estimates of fetal weight and growth patterns are most accurately assessed by measuring the fetal abdominal circumference.
Intrauterine Growth
Substandard growth rates, intrauterine growth restriction (IUGR), can result from a multitude of pathologic and nonpathologic processes (see later discussion). The original term “intrauterine growth retardation” is no longer used, because the use of the word “retardation” often alarmed parents who took it to mean that their baby would be “retarded” or mentally deficient.
At times, the existence of two terms that describe less-than-desired growth (IUGR) and small for gestational age (SGA) can cause confusion. Perhaps the easiest way to think about these terms is that IUGR is a term used to describe a pattern of fetal growth over a period, whereas SGA is the term used by pediatricians to describe a baby’s weight compared with its contemporaries born at the same gestational age. The significance of the label “small for gestational age” depends on the cut-off percentile used to define small. It is common to use the 10th percentile in population studies, because this gives a substantial number of babies to study while including probably 70% of babies that are genuinely growth restricted. However, most babies less than the 10th percentile will be “small normal,” and will therefore function normally. If a 3rd or 2nd percentile cut-off is used (approximately 2 standard deviations below the mean), a much higher proportion of babies will actually show dysfunction secondary to growth restriction. The term small for gestational age , despite its lack of specificity in relation to growth restriction, is still widely used, and is useful because it can be defined for all babies, whereas the growth trajectories of most babies remains unknown.
Epidemiology and Etiology of Fetal Growth Restriction
As previously discussed, normal fetal growth is dependent on the contributions of the mother, the placenta, and the fetus. The corollary to this is that aberrant fetal growth may result from disturbances in any of these same areas.
Race
Almost without exception, studies in the United States have demonstrated a significantly higher rate of LBW and its subcomponents, IUGR, and preterm birth, in African Americans when compared with their white contemporaries. However, the differences between the patterns of birth weight and mortality in the different races are not simple. First, studies in Europe have shown that black African mothers have an average gestational length that is about five days shorter than that of white mothers. This is compensated for by accelerated maturity in black Africans. An important study was carried out in South Carolina over a 20-year period, which showed that between 1975 and 1979, African-American babies younger than 37 weeks consistently had a lower perinatal mortality for gestational age than did white babies. However, this relationship reversed at term, a particular problem for African American babies being obstructed labor and meconium aspiration. Analysis of data between 1990 and 1994 showed that although gestation-specific perinatal mortality had reduced in both groups, the same pattern of lower mortality before 37 weeks, and higher mortality after 37 weeks in the black babies, persisted. A more recent study on 22 million births in the United States of mortality from 1989 to 1991, and then from 1999 to 2001, gave a similar result. In contrast to the findings with African Americans in the United States, European studies have shown that babies of South Asian origin have a raised perinatal mortality at all gestations compared with white babies. This is likely to be due to the fact that South Asian babies have a lower birth weight across the gestational age range. Babies born in India are on average approximately 600 g lighter than those born in Europe. However, studies of babies of South Asian racial origin born in developed countries shows that the deficit reduces to 300 g, but it persists. This highlights one of the potential pitfalls in correcting for racial origin in relation to birth weight by using “customized birth-weight percentile.” The reason there should be a systematically lower birth weight in South Asian babies remains conjectural, but it seems likely that it is an adaptation to the average smaller maternal size, thus minimizing deaths from obstructed labor. However, the long-term sequelae in this population include a very high incidence of diabetes and cardiovascular disease.
Prior Obstetric and Family History
Women who are younger than 15 years of age, older than 45 years of age, have a history of miscarriages or unexplained stillbirths after 20 weeks’ gestation, or have prior preterm deliveries, are at increased risk for delivering a growth-restricted baby. Familial factors also appear to play a role in the birth weight of babies. Mothers of LBW infants were frequently LBW infants themselves and are more likely to have subsequent LBW babies than other mothers, as are their siblings. However, the pathological implications of being small depend on the context. Being small in an otherwise large family cohort is likely to be pathologic, whereas being small in a family that is usually small is likely to be less of a problem.
Altitude
When comparing growth curves, most authors note that Lubchenco’s data were generated in Denver, the “mile-high city,” and that the 10th percentile thus generated is lower than the 10th percentile of data collected from centers closer to sea level. Yip was able to demonstrate a “dose-dependent” effect of altitude on the LBW rate, with a two- to threefold greater rate of LBW seen at altitudes greater than 2000 meters than at sea level.
Maternal Factors Contributing to Intrauterine Growth Restriction
In developed countries, a handful of maternal characteristics and behaviors have consistently been associated with an increased risk of growth restriction. In addition to race and prior obstetric history, the list includes maternal nutritional status (prepregnancy weight and weight gain during pregnancy), short stature, smoking, preeclampsia/hypertension, multiple gestation, and female sex of the infant. In developing nations, malaria is a significant factor.
Maternal Nutritional Status
Prepregnancy weight and weight gain during pregnancy, although indicators of maternal nutritional status, are independent variables. There is some evidence of the potential benefits of nutritional intervention in the mother who is poorly nourished before pregnancy but this remains controversial. Nutritional supplements provided to well-nourished women do not provide additional benefit. An obese mother is unlikely to deliver a growth-restricted baby, even if her pregnancy weight gain is low.
Smoking
Cigarette smoking, a habit practiced by 20% of pregnant Americans, has consistently been identified as a dose-dependent contributor to abruptio placentae, late fetal death, LBW, and IUGR. In developed nations, it is by far the single most important contributor to LBW. Rates of IUGR in smokers are 3 to 4.5 times that of nonsmokers, with average birth weights decreasing by 70 to 400 g. These adverse effects are particularly pronounced in babies born to older mothers. Elimination of smoking would diminish SGA rates by 20% to 30%. Multiple mechanisms may contribute to the detrimental effect of smoking during pregnancy. Nicotine and subsequent catecholamine release along with reduced synthesis of prostacycline result in placental vasoconstriction and elevated vascular resistance, decreasing delivery of nutrients and oxygen across the placenta. Levels of fetal carboxyhemoglobin are increased, further interfering with delivery of oxygen to the developing fetal tissues. Indirect effects by way of suboptimal nutritional status both before and during pregnancy have been suggested and are likely due to an increased rate of maternal metabolism rather than decreased maternal caloric intake. Smoking mothers consume more calories than their nonsmoking counterparts, and supplementing the diet of smoking mothers is ineffective in offsetting the detrimental effects on the fetus. If smoking mothers can be convinced to stop smoking before the third trimester, their infant’s birth weight will be indistinguishable from those babies whose mothers did not smoke at all.
A variety of other recreational drugs, including alcohol, marijuana, cocaine, and amphetamines, have likewise been associated with adverse fetal effects. With the exception of the fetal alcohol syndrome, the effect of these agents is not as well established or as pervasive as is tobacco. Certain prescription drugs, particularly the anticonvulsants, can result in fetal growth restriction and specific malformation syndromes.
Preeclampsia/Hypertension
Maternal chronic hypertension is an independent risk factor for SGA infants. Infants born to older mothers are at increased risk of being SGA ( Table 5-1 ). The worst perinatal outcome in hypertensive pregnancies is seen in those complicated by the superimposition of preeclampsia. Preeclampsia is not only a contributor to fetal growth restriction, but it also carries the most unfavorable prognosis in terms of severity of growth deficit. Both of these vascular-based problems are likely to produce their effects through a common placental disorder.
SGA Births (%) | ||
---|---|---|
Maternal Age | Normotensive | Chronic Hypertension |
<26 years | 10 | 6 |
26-30 years | 7 | 14 |
>30 years | 5 | 18 |
Multiple Gestations
The presence of more than one fetus in the uterus often results in SGA offspring. The onset of the growth restriction is determined by the number of fetuses: the more fetuses, the earlier growth restriction is likely to be observed.
Other
Finally, a variety of other maternally related factors have been proposed to play a role in the development of an SGA infant. Chronic medical conditions that interfere with maternal nutrition (inflammatory bowel disease, short gut syndrome), fetal oxygenation caused by decreased amounts of saturated hemoglobin (sickle cell disease, cyanotic heart disease), or oxygen and nutrient delivery caused by vasculopathies (advanced diabetes mellitus, chronic renal failure) can result in IUGR. The role of psychosocial stressors in IUGR is unclear. A summary of the relative contributions of the various factors with direct causal impact is provided in Figure 5-2 .

Placental Contributions
Placental tissue is fetal tissue. It follows that if circumstances exist that ultimately result in abnormal fetal growth, then the placenta will likewise be similarly affected. This has certainly been observed, with a significant correlation between birth weight and both placental weight and villus surface area. Likewise, there are placental pathologic correlates of known causes of IUGR (intrauterine infections, chromosomal anomalies, hypertensive disorders, twins) and gross placental and cord abnormalities (chronic abruptio placentae, choriohemangioma, extensive infarction, and abnormal cord insertions), which are likely to result in restricted fetal growth. On the other hand, the majority of cases of IUGR are idiopathic, with the epidemiologic risk factors discussed earlier (e.g., previous fetal losses, extremes of maternal age, previous preterm or SGA infant, substance abuse) as the only clue. The cause of growth failure in these infants is presumed to be the result of the ill-defined uteroplacental insufficiency. Human and animal in vivo studies, Doppler ultrasound investigations, and pathologic evaluations have identified an array of placental abnormalities that may well shed a unifying light on these apparently disparate groups of mother-infant dyads ( Box 5-1 ). As a result of these investigations, the central role of the placenta in the development of the growth-restricted baby is coming to the forefront.
Uteroplacental blood flow
-
Diminished blood flow
-
Increased vascular resistance
-
Absent spiral artery remodeling
-
Atherosis of vessels of parietal decidua
Fetoplacental blood flow
-
Increased irregularity of luminal size
-
Abnormal umbilical Doppler flow studies
-
Decreased number of placental arterial vessels
-
Decreased size of placental vessels
-
Decreased artery to villus ratio
Interface of maternal and fetal circulations
-
Cytotrophoblastic hyperplasia
-
Thickened basement membrane
-
Chronic villitis
Diminished Potential: Fetal Contributions
As described earlier, the genetic potential for growth is inherited from both parents and is the major determinant of early fetal growth, which is subsequently modulated by environmental factors. IUGR can also result from a variety of conditions (e.g., congenital infections) in which an otherwise normal fetus is prohibited from growing normally or if there is a genetic aberration that precludes the fetus from growing normally.
Congenital Infections
During the rubella pandemic of 1962 to 1964, IUGR was found to be the most consistent characteristic of congenitally infected infants. In this episode, 60% of the affected infants were less than the 10th percentile for weight at birth and 90% were less than the 50th percentile. Cytomegalovirus (CMV) is the infective organism most commonly associated with IUGR, although 90% of infants congenitally infected with CMV are asymptomatic. Hepatosplenomegaly and microcephaly with paraventricular calcifications are common findings in the symptomatic infant. Diagnosis is made most reliably with viral cultures of the urine obtained after birth. Human immunodeficiency virus has not been consistently associated with IUGR because other confounding variables have been difficult to separate. Although numerous other bacterial, protozoal, and viral pathogens are known to invade the developing fetus, most of these infants develop appropriately.
Genetic Factors
About 8% of all SGA infants have a major congenital anomaly. Conversely, the incidence of growth restriction in infants with significant congenital anomalies is 22%, nearly three times that of the general population, and a correlation exists between the number of malformations and frequency of IUGR. A wide array of chromosomal aberrations (aneuploidy, deletions, translocations) are associated with IUGR. The likelihood of finding a chromosomal disorder in an SGA infant with a congenital anomaly is approximately 6%. Chromosomal disorder, uniparental disomy, wherein a pair of homologous chromosomes are inherited from the same parent, has been associated with IUGR. Single-gene disorders and inborn errors of metabolism (maternal and fetal phenylketonuria) are likewise represented in this population. In addition there are well over 100 nonchromosomal syndromes associated with IUGR.
Identification and Management of Growth Restriction
A major problem is knowing which babies are at risk of growth restriction and should, therefore, have their growth monitored. Using classic risk factors, and even including regular fundal height measurements of the uterus, at best two thirds of babies with growth restriction can be detected antenatally, and in routine clinical practice the proportion is often much lower, typically about 30%. Attempts have been made to detect intrauterine growth restriction in low-risk populations using a single assessment at 32 to 34 weeks’ gestation; however, these have proved to be inefficient because a single measurement cannot indicate growth trajectory as opposed to size. Indeed, a Cochrane review showed that the harm from false-positive ultrasound diagnoses exceeds the benefit when screening is done in this way. Regular growth velocity profiling for every baby would be prohibitively expensive.
Currently, usual policy is to measure the fetuses thought to be at risk of growth restriction from maternal (e.g., hypertension) and epidemiologic factors (e.g., a previous growth-restricted baby) every 2 weeks. Measurements at more frequent intervals are not reliable indicators of poor growth because the change in fetal size is within the error of the measurement. If growth slows, then the next step is to measure umbilical artery blood flow velocity waveforms. A raised pulsatility index (ratio of systolic velocity to diastolic velocity), or even worse, absent or reversed end-diastolic flow, indicates increased resistance to perfusion of the placenta, putting the baby at risk of hypoxia. This investigation is well established as valuable and is part of routine surveillance in most tertiary centers; it can be carried out reliably by trained ultrasonographers. More expert fetal medicine specialists can move on to assessing fetal vascular redistribution as a response to early hypoxia. This redistribution can be detected by Doppler studies of key fetal organs, including the brain (by study of the middle cerebral artery), to detect the maintenance of the oxygen supply to them, thereby protecting their vital functions. At the same time, flow to less vital organs is reduced. Impaired cardiac function in the fetus can be demonstrated using venous Doppler examination of the precordial veins (ductus venosus, inferior vena cava, or superior vena cava), hepatic veins, and head and neck veins. Combining the umbilical artery, middle cerebral artery, and venous Doppler examinations provides information about the degree of placental disease, the level of redistribution, and the degree of cardiac compromise respectively. Changes in the venous Doppler usually precede abnormalities of the fetal heart rate pattern. However, at present, the use of venous Doppler remains largely a research tool, and data from prospective trials of its utility in preventing unexpected intrauterine demise are required before it is widely adopted.
There is no currently known effective treatment to improve the growth pattern of a fetus. If it is likely, because of failing growth, that delivery will be necessary at <34 weeks’ gestation, antenatal steroids should be given to improve pulmonary maturity before elective delivery. Prenatal management is aimed at determining the best time and mode of delivery. Gestational age is a critical factor in this decision. Deciding on the appropriate time of delivery is particularly difficult before 30 weeks’ gestation, when the risks to the neonate are substantial. Early delivery of growth-restricted fetuses with an abnormal umbilical artery waveform results in a high liveborn rate but at the cost of a high neonatal mortality and morbidity. Alternatively, delaying delivery until the fetal heart rate pattern is abnormal has been reported to result in a nearly fivefold increase in stillbirths; neonatal deaths before discharge fall by more than one third; overall, the total mortality is unchanged; and there is some evidence that long-term outcome may be improved. Therefore, if a fetus with growth restriction has an abnormal umbilical artery waveform, twice daily monitoring of the fetal heart rate pattern is recommended, and delivery should be undertaken as soon as the heart rate pattern becomes abnormal.
Small for Gestational Age Infants
Lubchenco et al. defined SGA as being birth weight less than the 10th percentile. By definition then, 10% of all newborns in a given population are too small. Others have proposed or have indeed used other cut-off values (e.g., the 25th, 15th, 5th, or 3rd percentile) or 2 standard deviations from the mean, which would correspond to approximately 2.5% of the population. No matter what cutoff is used, there may be multiple factors that result in a large discrepancy in absolute weights seen at the lowest “normal” percentile. Goldenberg reviewed several reports wherein the 10th percentile was used as the definition of IUGR. More than a 500-g difference was noted across these studies. This observation, however, may have been a reflection of the disparate methodologic approaches among the studies. Goldenberg’s plea for a concerted effort by a variety of national professional academies and federal agencies to endorse a national standard has, so far, gone unheeded. The approach of using any percentile as an absolute criteria has been thoughtfully challenged by Chard et al., who point out that not only is there no statistical evidence of a subpopulation of growth-restricted babies at term, but also, by using the 10th percentile, many cases of IUGR (defined as a failure to achieve growth potential) will be missed. Although these may be seen as interesting, if somewhat arcane academic issues, the practical importance becomes apparent when one is responsible for making decisions regarding expensive and painful laboratory evaluations; when there is the need to monitor the infant in a more costly special care nursery; and when there are future referrals for formal developmental evaluations.
Excluding SGA infants who have significant congenital anomalies and infections, there is a group of SGA infants with a relatively characteristic physical appearance. Their heads are often disproportionately large for their trunks, and their extremities typically appear wasted. The nails are long. The facial appearance has been likened to that of a “wizened old man.” The anterior fontanelle is often larger than expected, and the cranial sutures may be widened or overlapping. The umbilical cord is typically thin with little Wharton’s jelly and may be meconium stained; the abdomen is scaphoid, which may mislead the examiner into considering a congenital diaphragmatic hernia. Subcutaneous fat and tissue are diminished, resulting in loose skin on the arms, legs, back, abdomen, and buttocks. Like the umbilical cord, the skin may be stained from meconium passed in utero and be unusually dry and flaky with little protective vernix caseosa present.
It is often suggested that measuring the weight, length, and head circumference allows further classification of the SGA infant as either symmetrically growth restricted (those infants with decreased length and head circumference) or asymmetrically growth restricted (relatively normal length with relative “head sparing”). Such a distinction has been proposed as both a diagnostic tool and prognostic marker. The symmetrically growth-restricted newborn, historically representing 20% of all SGA infants, is thought to result from an injury or process (congenital infection, genetic disorders) that occurred or began in the early stages of the pregnancy, during the phase of growth primarily characterized by cellular hyperplasia. The prognosis for eventual growth and development of these infants is somewhat guarded, in large part because of the underlying etiology. The asymmetrical (“wasted”) SGA baby, on the other hand, has been proposed to result from a third trimester insult interfering with delivery of oxygen and nutrients (the effect of maternal hypertensive disorders, maternal starvation, advanced diabetes) during the cellular hypertrophy phase of fetal growth. This latter group has been projected to expect a much brighter future than their symmetrical brethren. Various indices such as the Ponderal Index (PI = Birth weight × 100/Length ) have been used to further describe or quantify the relationship between length and weight and identify these subgroups.
Whereas such an outline may appeal to one’s sense of logic, recent data have necessitated a reevaluation of this approach. Chard et al. demonstrated that there is a continuous relationship between the PI and weight throughout the entire range of normal birth weight. Infants in the lower half of the population have a lower PI than those in the upper half. In other words, smaller infants tend to be thinner, and larger infants tend to be fatter. Kramer et al. when excluding infants with evidence of major malformations and congenital infections, likewise found a direct relationship between severity of growth restriction and a decreasing PI, arguing against distinct subgroups of proportional and disproportional infants. Similarly, a normal frequency distribution of head-to-abdominal circumference ratio is seen in antenatal ultrasound assessments of growth-restricted fetuses, with increased severity of growth restriction being associated with increased asymmetry. Even the relative frequency of the two groups has come under question; in some populations, symmetrical SGA infants are found more frequently than asymmetrical infants. The concept of asymmetry serving as a diagnostic tool has been further challenged by Salafia, who found that IUGR preterm infants born to mothers suffering from preeclampsia were far more likely to be symmetrical than asymmetrical, and David, who found an equal distribution of a small number of chromosomal abnormalities between the symmetrical and asymmetrical populations. In summary, although it may be premature to completely discard the framework of symmetry and asymmetry in intrauterine growth restriction, one should feel uncomfortable with a dogmatic approach to its use in the SGA infant.
Clinical Problems
Perinatal and Neonatal Morbidity and Mortality
The growth-restricted fetus and newborn have a higher perinatal mortality at each gestation compared with those of appropriate weight for gestation infants, whether preterm, term, or postterm ( Fig. 5-3 ). They also have a variety of other adverse outcomes, reflecting the underlying plethora of diagnoses and chronic and acute deprivations of oxygen and nutrients. Overall, the perinatal mortality among growth-restricted infants is eight to ten times greater than for infants who have grown normally. The risk of perinatal morbidity and mortality increases markedly with the degree of growth restriction ( Fig. 5-4 ).
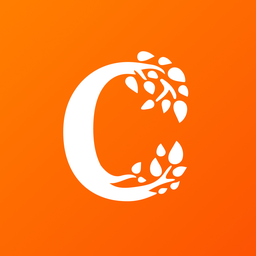
Full access? Get Clinical Tree
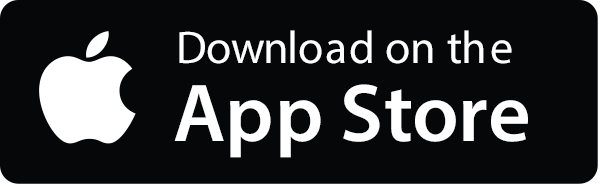
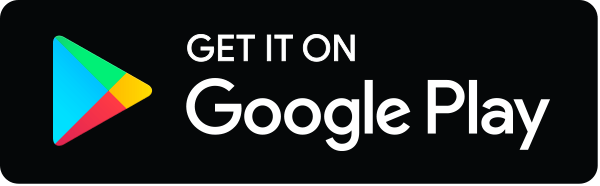