Conventional ventilator settings
Common pitfalls
Mode:
Variable, decelerating inspiratory flow pattern is preferred to (a) optimize patient comfort and (b) augment mean airway pressure while minimizing peak inspiratory pressure
Volume-limited or pressure-limited modes may be safely applied to pediatric patients
Applying adult settings to pediatric patients
Excessively long inspiratory time and/or high set respiratory rates leading to “gas trapping” and intrinsic PEEP
Tidal volume: approximately 6 ml/kg ideal body weight for patients with acute lung injury; 6–10 ml/kg for pediatric patients with normal lungs
Overdistending healthy lungs with excessive tidal volume
Pressure:
PIP < 30 cm H2O
PEEP to maintain an open-lung approach without adversely affecting hemodynamics
Overdistending healthy lungs with excessive airway pressure
FiO2: strive to maintain FiO2 ≤ 0.50
Excessive oxygenation targets
Inspiratory time (T i):
Appropriate T i (based on patient age/size):
Optimize patient comfort
Avoid air trapping
Longer T i may be needed to maintain higher mean airway pressure in patients with significant acute lung injury
Excessively long T i in tachypneic patients, leading to air trapping and intrinsic PEEP
Clinical targets
pH:
Normal pH (7.35–7.45) if minimal ventilator settings
Generally allow lower pH (7.20–7.30) in hemodynamically stable patients on increased ventilator settings; allow elevated PaCO2 (permissive hypercapnia), except in those patients with increased intracranial pressure or significant, reactive pulmonary hypertension
Over ventilating injured lungs to achieve normal pH
PaCO2:
Allow pH targets to dictate PaCO2
Consider disease-specific PaCO2 targets (e.g., pulmonary hypertension, traumatic brain injury)
Over ventilating injured lungs to achieve normal PaCO2
SpO2 :
Wean oxygen to maintain normal oxygenation (generally SaO2 > 93 %)
Tolerate SaO2 > approx. 85–88 % for patients with adequate oxygen delivery and requiring “toxic” ventilatory support (permissive hypoxemia)
Maintaining high levels of FiO2 to maintain supranormal SaO2
It should be noted that there are circumstances in which invasive mechanical ventilation may be avoided by the utilization of noninvasive ventilation (NIV) as discussed elsewhere in this textbook. Even in the setting of acute lung injury (ALI) and ARDS, patients may be effectively and successfully treated with NIV. However, the use of NIV in infants and children can be complicated by difficulties with both the patient interface and patient cooperation, thus, potentially necessitating intubation and invasive support. The focus of this chapter is on the processes and considerations surrounding implementation of invasive mechanical ventilation in the pediatric critical care environment.
43.2 Mode of Invasive Mechanical Ventilation
The key to an optimal approach to invasive mechanical ventilation is matching the advantages/disadvantages of a specific ventilatory mode to an individual patient’s pathophysiology. From the start, it must be stressed that no clinical neonatal or pediatric study has definitively demonstrated that any one mode is more beneficial than another in improving outcomes, except for high-frequency ventilation in the setting of neonatal respiratory distress syndrome in which the incidence of chronic lung disease is reduced (Campbell and Davis 2002; Branson and Johannigman 2004; Fan et al. 2005; Ortiz 2010; Maxwell et al. 2010; Duyndam et al. 2011; González et al. 2010; Cools et al. 2009; Hofhuis et al. 2002).
Conventional mechanical ventilation includes three general mode types: assist control (e.g., pressure control ventilation), support (e.g., pressure support ventilation), and mixed (e.g., synchronized intermittent mandatory ventilation (SIMV) plus pressure support). No data exist to support the superiority of any of these approaches, and advantages and disadvantages of each type of mode can be debated (Campbell and Davis 2002; Branson and Johannigman 2004; Fan et al. 2005; Ortiz 2010; Maxwell et al. 2010; Duyndam et al. 2011; González et al. 2010).
A control mode is defined by a clinician-set inspiratory time, while a support mode is defined by a patient-determined inspiratory time. Control modes are occasionally used in the Pediatric ICU, but most pediatric patients prefer an inspiratory time (T i) shorter than generally chosen by the clinician. These patients, therefore, tend to be more comfortable in a support mode (i.e., patient-controlled T i) (Banner et al. 1991). However, pediatric patients, especially infants, may become significantly tachypneic in a support mode, further decreasing T i and subsequently decreasing mean airway pressure (P AW). As P AW falls, this clinical scenario may lead to atelectasis and a subsequent need for increasing positive end-expiratory pressure (PEEP). One approach to the use of support modes (e.g., pressure support ventilation) in infants is to set the PEEP at approximately 2 cm H2O above the lower inflection point to maintain the P AW in the event that the patient’s intrinsic inspiratory time were to decrease.
Alternatively, a mixed control/support mode may be utilized. With such an approach, the support breaths improve patient comfort, while a set rate of controlled breaths with a longer T i helps to maintain mean airway pressure and lung recruitment. A key consideration in this context is to set T i for the control breaths relative to the baseline respiratory rate, to allow for adequate expiratory time. The maintenance of adequate expiratory time in this manner avoids air trapping and the development of intrinsic PEEP. It should be stressed that with the lack of definitive data supporting one mode over another, the choice of ventilatory mode is often left to institutional bias and individual clinical choice. The mode chosen for a given patient should be based on the patient’s pathophysiology, keeping in mind that this may change over time.
In an attempt to optimize patient-ventilator synchrony and minimize pharmacologic sedation, several manufacturers have developed novel approaches to conventional mechanical ventilation. Increasing emphasis is being placed on spontaneous breathing and its potential benefits in the management of acute respiratory failure. Such modes include proportional assist ventilation (PAV), neurally adjusted ventilatory assist (NAVA), and adaptive support ventilation (ASV) (Beck et al. 2007; Colombo et al. 2008; Schmidt et al. 2010; Spahija et al. 2010; Terzi et al. 2010; Sinderby et al. 2007; Barwing et al. 2009; Coisel et al. 2010; Alander et al. 2012; Bengtsson and Edberg 2010; Younes 1992; Kacmarek 2011; Giannouli 1999; Kondili et al. 2006; Wrigge et al. 1999; Xirouchaki et al. 2008). These nontraditional approaches are designed to share the ventilating pressure between patient and ventilator to proportionally unload respiratory effort and, thus, theoretically improve synchrony. In these modes of ventilation, the patient maintains control of the ventilatory pattern. From a physiologic basis, this approach both makes sense and is supported by several relatively small studies; however, definitive outcome data are lacking (Beck et al. 2007; Colombo et al. 2008; Schmidt et al. 2010; Spahija et al. 2010; Terzi et al. 2010; Sinderby et al. 2007; Barwing et al. 2009; Coisel et al. 2010; Alander et al. 2012; Bengtsson and Edberg 2010; Younes 1992; Kacmarek 2011; Giannouli 1999; Kondili et al. 2006; Wrigge et al. 1999; Xirouchaki et al. 2008). A comprehensive review of these novel approaches to pediatric mechanical ventilation is beyond the scope of this chapter.
When appropriate clinical targets cannot be achieved with “safe” conventional ventilator settings using the more traditional modes, providers must consider alternative forms of ventilation. High-frequency oscillatory ventilation (HFOV), high-frequency jet ventilation (HFJV), high-frequency percussive ventilation (HFPV), and airway pressure release ventilation (APRV) usually improve oxygenation and/or ventilation, presumably, in a more lung-protective manner.
Each of these modes has advantages and disadvantages, which should be considered when choosing a ventilation strategy. APRV allows spontaneous ventilation throughout the ventilatory cycle and, thus, would be expected to require reduced sedation and neuromuscular blockade requirements as compared to other modalities (Maxwell et al. 2010; Duyndam et al. 2011; González et al. 2010). Although APRV offers an attractive strategy for the pediatric patient with acute lung injury, it has not seen widespread application. Additionally, outcome data have not proven this to be a beneficial approach as compared to more conventional approaches (Maxwell et al. 2010; Duyndam et al. 2011; González et al. 2010; Maung et al. 2012).
HFOV functions at an elevated mean airway pressure with an attenuated pressure amplitude at the alveolar level to augment oxygenation while providing lung-protective ventilation. HFOV has become a standard approach in many neonatal and pediatric intensive care units, although definitive outcome data in the pediatric population remain lacking (Arnold et al. 2000). HFJV is limited to the neonatal and infant population due to the technical limitations of the device (i.e., patient size limitations). The key advantage of HFJV is its continuous passive exhalation, an extremely efficient approach to carbon dioxide elimination (Meliones et al. 1991; Musk et al. 2011; Bunnell 2001). In the small pediatric population, HFJV is most helpful in those patients with pulmonary hypertension in which the partial pressure of carbon dioxide must be closely controlled and/or those patients with acute lung injury in whom permissive hypercapnia would not be acceptable. Lastly, HFPV provides the additional benefit of enhancing secretion removal while improving oxygenation and/or ventilation and providing a lung-protective approach to patient management.
Clinical targets using these modes of ventilation should include both permissive hypercapnia and permissive hypoxia (Arnold et al. 2000; Rotta and Steinhorn 2006; Kavanagh et al. 2006; Peltekova et al. 2010; Cheifetz and Hamel 2006; Cheifetz 2011a; Abdelsalam and Cheifetz 2010) as clinically indicated and remain generally the same as with conventional ventilation as discussed elsewhere in this text. If the patient continues to deteriorate despite “maximal” ventilatory support, extracorporeal membrane oxygenation (ECMO) should be considered for refractory respiratory failure.
43.3 Tidal Volume
In adult patients with acute lung injury, maintaining the tidal volume at 6 ml/kg of ideal body weight (IBW) and maintaining the plateau pressure less than 30 cm H2O significantly reduced mortality when compared with a higher tidal volume strategy of 12 ml/kg IBW (The Acute Respiratory Distress Syndrome Network 2000). This study controlled for both plateau pressure and delivered tidal volume, and despite multiple additional clinical trials, it remains controversial whether lung overdistension (i.e., volutrauma) or excessive pressure (i.e., barotrauma) is more damaging to the lung. It is most likely that both pressure and volume in combination play a significant role in VILI. As pressure and volume are intrinsically linked by compliance, the same strategies that limit one will generally limit the other.
Extrapolating these adult data to children with acute lung injury, it is likely safe to target a tidal volume of 6 ml/kg IBW while keeping the peak inspiratory pressure less than 30 cm H2O. However, it must be stressed that definitive pediatric data are lacking. With the increasing prevalence of obesity in the pediatric population, it is important to stress that these tidal volume calculations must be performed on ideal, rather than actual, body weight (Cheifetz 2011a, b).
If tidal volume targets are maintained and appropriately monitored, different modes of ventilation (i.e., volume-limited vs. pressure-limited) should be equivalent. Either mode can usually deliver the same sized mechanical breaths without substantial differences, and there are no data to suggest one modality is superior to another. It should again be stressed that the specific mode is much less important than the clinical management of the mode based on the physiology and the clinician-set ventilator parameters.
Another important variable which must be considered when setting tidal volume is the location of the tidal volume measurements. The tidal volume set (or measured) on the ventilator may be significantly higher than the tidal volume a patient’s lungs actually receive (referred to as the delivered or effective tidal volume) (MacIntyre 1990; Tobin 1992; Cannon et al. 2000). This difference is a result of the compressible volume of gas in the ventilator circuit and is magnified in neonates and small infants as the quantity of compressible gas is a relatively larger percent of the ventilator-delivered tidal volume (Cannon et al. 2000; Binda et al. 1976; Epstein 1971; Haddad and Richards 1968; Martin et al. 1989; Castle et al. 2002; Chow et al. 2002; Heulitt et al. 2009).
Thus, accurate monitoring of tidal volume can be difficult in neonates, infants, and small children. Expiratory valve measurements, on average, may be 44 % higher for neonatal circuits and 27 % higher for pediatric circuits than the tidal volume measured at the endotracheal tube (Cannon et al. 2000). These inaccuracies are due to both a larger circuit dead space to tidal volume ratio and a greater effect of circuit compliance on tidal volume measurement. Some mechanical ventilators employ a calculation to estimate the effective delivered tidal volume taking into account the compliance of the ventilator circuit. However, such calculations may be affected by changes in the compliance of the ventilator circuit over time (e.g., temperature variations, secretions, circuit adaptors).
For these reasons, it has been recommended that the actual tidal volume delivery be monitored and managed using a pneumotachometer placed between the endotracheal tube and ventilator circuit (Cannon et al. 2000; Castle et al. 2002; Chow et al. 2002; Heulitt et al. 2009). Measuring exhaled tidal volume at the endotracheal tube leads to a more accurate determination of the actual tidal volume delivered to a small pediatric patient’s lungs, allowing providers to better prescribe tidal volume and subsequently follow a lung-protective strategy.
43.4 Positive End-Expiratory Pressure
The application of PEEP is an essential step in providing respiratory support for mechanically ventilated patients of all ages (Dantzker et al. 1979; Pontoppidan et al. 1972a, b, c). If set appropriately, PEEP helps to maintain an appropriate lung volume, minimize atelectasis, improve ventilation/perfusion matching, and reduce right-to-left intrapulmonary shunting (Hammon et al. 1976; Suter et al. 1975; Tyler 1983). Determining “optimal” PEEP remains a clinical challenge. Several methods may be employed to set the “best” PEEP for an individual patient. One approach is to use pressure-volume curves to set the PEEP at approximately 2–3 cm H2O above the lower inflection point (Fig. 43.1). Once the lungs are recruited using this method, clinicians should then wean the PEEP to the minimal value needed to keep the lung open, while closely monitoring the pressure-volume curve. Another method of PEEP titration involves the use of PEEP-FiO2 tables. Unfortunately, generally accepted PEEP-FiO2 tables are only available for the adult population.
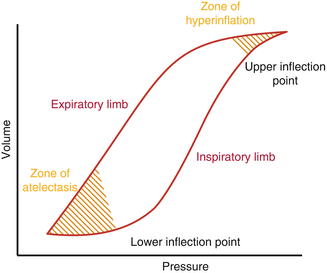
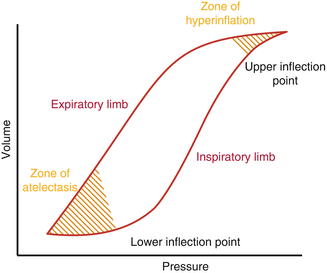
Fig. 43.1
Pressure-Volume curve of the lung
As a general PEEP titration approach, as modeled in Table 43.2, providers may vary PEEP in a stepwise fashion to determine which level of PEEP best optimizes a range of clinically relevant markers. This method of PEEP titration can be important for patients with multiorgan dysfunction in that it considers the effects of PEEP on oxygen delivery and cardiac function. While the impact on nonpulmonary organs and overall oxygen delivery is an important consideration, unfortunately, cardiac output remains challenging to accurately measure in pediatrics. In children and neonates, surrogate measures of oxygen delivery must often be substituted to estimate the impact of PEEP titration. The ultimate goal is to find the “best” PEEP for a given patient that optimizes pulmonary compliance and global oxygen delivery (i.e., the best balance between lung volume, cardiac output, and global arterial oxygen delivery).
Table 43.2
Sample PEEP table
PEEP = 6 | PEEP = 9 | PEEP = 12 | PEEP = 15 | |
---|---|---|---|---|
Lung expansion | Atelectatic | Atelectatic | Ideal | Hyperinflated |
Pulmonary vascular resistance | ++ | + | − | + |
Cardiac output | ++ | + | No change | −− |
Oxygenation | −− | − | + | ++ |
Intracranial pressure | −− | +/− | +/− | ++ |
Oxygen delivery | − | +/− | + | − |
Regardless of the method chosen, an important consideration in setting PEEP is the principle of hysteresis. Hysteresis demonstrates that more energy is required to open the lungs than to maintain lungs open. VILI is worsened by the atelectrauma created by the cycle of alveolar collapse and reopening created by inadequate end-expiratory pressure. Once atelectasis develops, additional energy, delivered in the form of increased airway pressure, is required to reopen the alveoli (Prodhan and Noviski 2004). This need for increased pressure to overcome alveolar collapse exacerbates VILI.
Adequate PEEP and open-lung strategies attempt to maintain appropriate lung inflation during exhalation to maintain the alveoli in their phase of optimal compliance and, hopefully, to avoid (or at least minimize) iatrogenic lung injury (Rimensberger 2009). An additional approach to the prevention of atelectrauma is the use of recruitment or sustained inflation maneuvers. While their use remains controversial in both the pediatric and adult populations, carefully performed lung recruitment maneuvers may be safely used to achieve an open-lung state (Prodhan and Noviski 2004; Turner and Arnold 2007). It should be noted that careful attention must be paid to avoiding pulmonary hyperinflation during recruitment maneuvers and PEEP titration. One mechanism to monitor for hyperinflation/overdistension is the pressure-volume loop (Fig. 43.1). These loops can alert providers of hyperinflation through the presence of decreasing compliance, “beaking,” and an upper inflection point. Careful clinical monitoring is crucial as PEEP is adjusted while balancing optimal lung inflation with the risk of VILI.
43.5 Inspiratory Flow Pattern
The choice of inspiratory flow pattern has an impact on ventilatory mode selection and often depends on the specific ventilator(s) available. There may be several options for inspiratory flow pattern, but in most situations, inspiratory flow is either constant or decelerating. With a constant inspiratory flow pattern, a “square wave” is produced by the constant flow of gas throughout the inspiratory cycle. In many clinical scenarios, this flow pattern may be associated with less comfortable spontaneous respiratory effort and increased patient-ventilator dyssynchrony.
In contrast, a variable inspiratory flow pattern generally provides the maximal flow near the onset of inspiration with flow then decreasing at a variable rate throughout the remainder of inspiration. This variable, decelerating flow pattern is generally preferred over a constant flow pattern in most patients as it is a more natural inspiratory breathing pattern that provides sustained mean airway pressure with a lower peak inspiratory pressure when compared to constant flow (Fig. 43.2) (Cinnella et al. 1996; Wong et al. 2000). This pattern maximizes P AW with lower peak inspiratory pressures, allowing clinicians to achieve oxygenation goals with potentially less risk for VILI (Cheifetz 2003). In addition, the more natural breathing pattern of decelerating flow would be expected to lead to better patient-ventilator synchrony, which may in turn decrease sedation requirements.
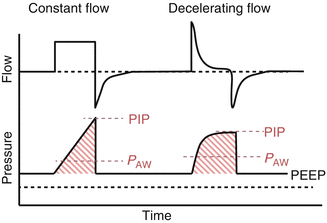
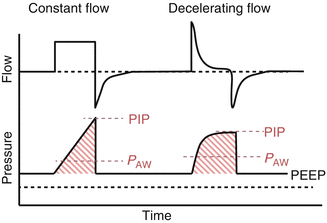
Fig. 43.2
Flow and Pressure vs. Time Scalars comparing constant versus decelerating inspiratory flow patterns
43.6 Triggering
Setting ventilator trigger for infants and children can be challenging given the variation in size and disease processes. Many infants and small children have difficulty triggering ventilators because of their relatively weak respiratory musculature. Thus, flow triggering is generally preferred to pressure triggering.
Flow triggers allow for increased ventilator sensitivity in situations where small patients are unable to generate adequate pressure to trigger the ventilator. A small subset of the weakest infants may require flow triggers less than 0.5 l/min. However, auto-triggering can become a risk, especially in the setting of leaks around the endotracheal tube. While becoming less common, uncuffed endotracheal tubes are still frequently utilized in a wide range of clinical scenarios, and the air leak around these tubes can be quite variable. Patients with large air leaks may require higher flow triggers (up to 2.0 l/min) to maintain clinical targets and achieve gas exchange goals without autocycling.
43.7 Inspired Oxygen Concentration
While oxygen is required by the body for cellular respiration and metabolism, elevated levels can have damaging effects on the lung by producing free radicals and reactive oxygen species (Vento et al. 2009; Yee et al. 2009; Frank et al. 1978; Carvalho et al. 1998). The toxicity of high levels of inspired oxygen can lead to direct damage to cell membranes, cellular proteins, and DNA, thus, leading to cell necrosis, apoptosis, and further inflammation (Auten and Davis 2009). Minimizing FiO2 in the setting of mechanical ventilation is an important consideration, and there are limited data to suggest that an FiO2 less than 0.50 will ameliorate the toxic effects (Dos Santos 2007). However, increased ventilator settings (e.g., mean airway pressure) may be necessary to decrease the FiO2. In patients with refractory hypoxemia, substantial increases in ventilatory support may be needed, and the balance between oxygen toxicity and potential VILI may become increasingly difficult to manage.
43.8 Ventilatory Frequency
In infants and children, the normal physiologic respiratory rate varies significantly depending on patient age. The patient’s expected physiologic rate should largely dictate the set ventilatory frequency. As a general guideline, deviation from normal physiologic respiratory patterns with regard to rate and inspiratory time should be avoided.
When spontaneous or mixed modes of ventilation are utilized, the frequency may be set below the patient’s physiologic rate, allowing the patient to breathe above the set minimum (or backup) rate. In clinical scenarios in which the predominant pathophysiology is prolonged expiration (i.e., asthma, bronchiolitis), it is often required that the respiratory frequency be decreased to avoid premature termination of exhalation and gas trapping (i.e., auto-PEEP or intrinsic PEEP). Gradually decreasing the set frequency with an increased dependence on spontaneous ventilatory effort is often used as an approach to ventilator weaning.
In contrast, an increase in respiratory frequency above the “normal” physiologic rate is rarely necessary unless hyperventilation is a specific clinical goal. Therapeutic hyperventilation may be necessary in certain clinical circumstances, and refractory respiratory acidosis may also necessitate an increased ventilator frequency. When increased frequency is necessary to achieve clinical endpoints, close monitoring and analysis of airway graphics are necessary to prevent inadvertent inverse ratio ventilation or “gas trapping.”
43.9 Inspiratory Time
Inspiratory time is generally set at 0.40–0.65 s in infants, 0.5–0.75 s in children, and 0.7–1.0 s in adolescents. Inspiratory time is generally not reduced below approximately 0.40 s in the pediatric critical care environment due to the possibility that shorter times may not allow adequate time for the distribution of gas to the alveolar units. It is important for the bedside clinician to monitor the flow-time scalar to ensure that expiratory flow returns to baseline (i.e., zero flow) prior to the initiation of the next breath. Such an approach will avoid the occurrence of gas trapping and intrinsic PEEP.
Inspiratory time may be increased to augment P AW and improve oxygenation, but prolongation of the inspiratory time may result in an excessively high inspiratory-to-expiratory ratio and “gas trapping.” An excessive prolongation of inspiratory time can also result in significant elevations in the P AW resulting in an impedance to systemic venous return, a reduction of right ventricular filling, and a resultant decrease in cardiac output (Cournand et al. 1948). Historically, a prolongation of the inspiratory time in this manner with an increased, or reversed, inspiratory/expiratory ratio had been advocated as a means of increasing P AW and recruiting low ventilation/perfusion compartments in diseases involving decreased compliance and lung volumes (Reynolds and Taghizadeh 1974). More recently, the use of APRV has provided for significant elevations in the mean airway pressure, while still allowing spontaneous ventilation and avoiding gas trapping.
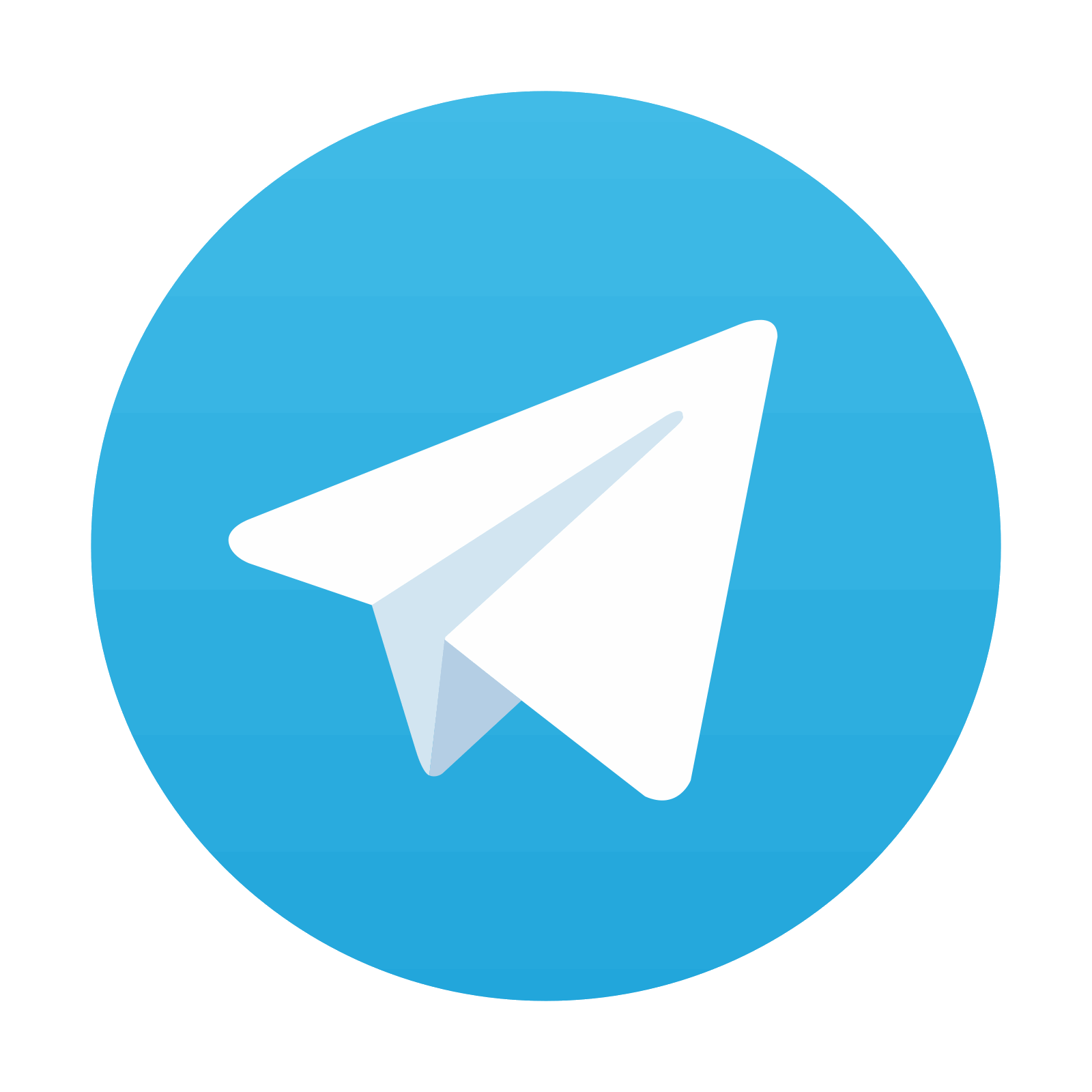
Stay updated, free articles. Join our Telegram channel
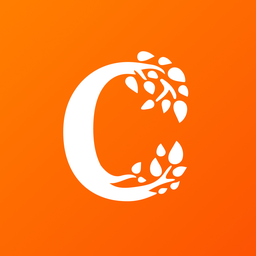
Full access? Get Clinical Tree
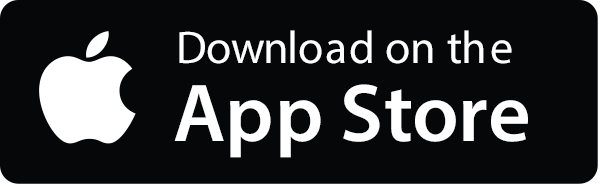
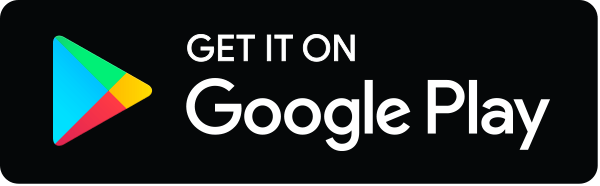