(3)
Department of Pediatrics, UC Davis Children’s Hospital, University of California, Davis, 2516 Stockton Blvd, Sacramento, CA 95817, USA
Educational Objectives
Understand the regulation and physiologic functions of the nitric oxide-cGMP signaling pathway during normal pulmonary development.
Describe dysregulation of NO production and signaling in common neonatal and pediatric pulmonary and cardiac diseases.
Determine the clinical utility of inhaled nitric oxide in pediatric pulmonary and cardiac disease by utilizing physiologic principles and clinical evidence.
29.1.1 Nitric Oxide Physiology
29.1.1.1 NO Physiology in the Normal Respiratory System
Nitric oxide (NO) is synthesized by the enzyme nitric oxide synthase (NOS) as a product of the conversion of l-arginine to l-citrulline. Three NOS isoforms are expressed in the lung, which are characterized by the regulation of their activities and by specific sites and developmental patterns of expression (Shaul 2002). Endothelial NOS (NOS3) is expressed in vascular endothelial cells and is the predominant source of NO production in the pulmonary circulation. Neuronal NOS (NOS1) and inducible NOS (NOS2) are both expressed in the airway epithelium and at low levels in the vascular smooth muscle. Furthermore, the expression of NOS2 can be induced in a variety of pulmonary cell types by exposure to endotoxin or inflammatory cytokines.
NOS is a complex oxidoreductase enzyme that functions physiologically as a dimer (Konduri and Kim 2009). A particularly important aspect for normal NOS function is sufficient availability of substrate (l-arginine) and cofactors. Interaction of eNOS with its critical cofactors such as heat shock protein 90, an intracellular molecular chaperone, and tetrahydrobiopterin, a bioactive form of folic acid, is necessary to facilitate NO production by eNOS (Landmesser et al. 2003). In contrast, depletion of these cofactors or inhibition of hsp90-eNOS interactions leads to “uncoupled” NOS activity, which promotes release of the oxygen radical superoxide instead of NO. In addition to substrate and cofactors, NOS expression and activity are regulated by multiple other factors, including oxygen tension, hemodynamic forces, hormonal stimuli (e.g., estrogen), paracrine factors (e.g., VEGF), and reactive oxygen species (Grover et al. 2003; MacRitchie et al. 1997; Mata-Greenwood et al. 2006; North et al. 1996).
NO is a gas molecule that can diffuse freely from the endothelium to the vascular smooth muscle cell. The biologic effects of NO in vascular smooth muscle are mediated primarily through activation of soluble guanylate cyclase, which promotes conversion of GTP to cGMP (Fig. 29.1). cGMP relaxes vascular smooth muscle through activation of cGMP-gated ion channels and activation of cGMP-dependent protein kinase (PKG). However, recent studies indicate that alternative NO signaling pathways likely also exist through reaction of NO with protein thiols to form S-nitrosothiols (SNO), which may induce vasodilation or protein modification (Gaston et al. 2003).
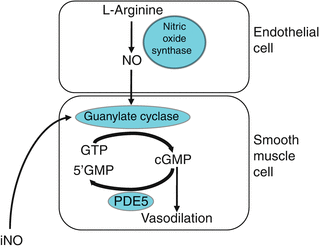
Fig. 29.1
Nitric oxide (NO) signaling pathways in the regulation of pulmonary vascular tone. NO is synthesized by nitric oxide synthase (NOS) from the terminal nitrogen of l-arginine. NO from endogenous sources, or delivered as an inhaled gas, stimulates soluble guanylate cyclase (sGC) to increase intracellular cGMP, which indirectly decreases free cytosolic calcium, resulting in smooth muscle relaxation. Specific phosphodiesterases such as PDE5 hydrolyze cGMP, thus regulating the intensity and duration of its vascular effects. Inhibition of cGMP-phosphodiesterases with agents such as sildenafil may enhance pulmonary vasodilation
NO is detectable in expired gas, and studies in both animals and humans suggest that the principle source of expired NO is the lung epithelium rather than the pulmonary vasculature (Shaul 2002). The functions of NO in the airway include neurotransmission, smooth muscle relaxation, and bacteriostasis, and also the modulation of mucin secretion, ciliary motility, and in the perinatal period, regulation of lung liquid production.
Phosphodiesterases serve to catalyze the breakdown of cGMP, and are therefore able to regulate the magnitude and duration of vasodilation observed in response to NO. Each family of PDEs has a distinct pattern of specificity for cAMP and/or cGMP, and each PDE isoform has specific tissue and cellular distributions (Soderling and Beavo 2000). The prevalent PDE within the lung is the cGMP-specific PDE5, although significant amounts of PDE1, PDE3, and PDE4 are also present (Maclean et al. 1997; Pauvert et al. 2002). As the primary enzyme responsible for regulating cGMP, PDE5 potentially represents the most important regulator of NO-mediated vascular relaxation in the normal pulmonary vascular transition after birth (Abman et al. 1990; Lakshminrusimha and Steinhorn 1999).
29.1.1.2 Developmental Regulation of NO Physiology
The fetus must maintain a state of pulmonary hypertension to survive and optimally use the oxygen provided from the placental circulation. Because the placenta, not the lung, serves as the organ of gas exchange, most of the right ventricular output crosses the ductus arteriosus to the aorta, and only 5–10 % of the combined ventricular output is directed to the pulmonary vascular bed. Pulmonary vascular constriction plays a key role in maintaining high pulmonary vascular tone during fetal life. There are multiple pathways involved in maintaining high pulmonary vascular resistance in utero, including hypoxia (a defining feature of fetal development), as well as endothelin-1 (ET-1), thromboxane, acidosis, and various mediators of inflammation (Lakshminrusimha and Steinhorn 1999). At the same time, the fetal lung and pulmonary vasculature must prepare for the dramatic adaptation to air breathing at the time of birth. This complex orchestration of fetal and postnatal lung development is regulated in part by important maturational changes in expression and activity of nitric oxide synthase (NOS) isoforms.
Lung eNOS mRNA and protein is present in the early fetus and increases with advancing gestation and during the early postnatal period in multiple species (North et al. 1994; Parker et al. 2000). This increase in lung endothelial NOS content occurs at the same time the fetal pulmonary vasculature develops the capacity to respond to endothelium-dependent vasodilator stimuli, such as oxygen and acetylcholine (Tiktinsky and Morin 1993). Recent data indicate that low fetal NOS activity may be maintained during fetal life in part through increased levels of asymmetric dimethyl arginine (ADMA), an endogenously produced arginine analogue that acts as a competitive NOS inhibitor (Arrigoni et al. 2003; Pierce et al. 2004).
The neuronal and inducible NOS isoforms have been also been identified in the fetal lung of multiple species and appear to be primarily localized to the airway epithelium. Their expression and activity appear to be similarly regulated in specific developmentally driven patterns (Rairigh et al. 1998; Shaul et al. 2002; Sherman et al. 1999; Tzao et al. 2000). Similar to vascular NO, epithelial NO production increases during the third trimester and occurs in parallel with rises in nNOS and eNOS expression in the proximal lung (Shaul et al. 2002). NO is now recognized as important to epithelial development and function during lung development and appears to regulate critical functions such as lung liquid clearance by the respiratory epithelium at the time of birth (Cummings and Wang 2001), an essential component of the transition of the fetus to air breathing. Epithelium-derived NO is also critical to the regulation of bronchomotor tone in the early newborn period, playing an important role in the opposition of airway contraction (Steinhorn and Martin 2002).
Dramatic cardiopulmonary events at birth facilitate the transition to gas exchange by the lung; these include a rapid fall in pulmonary vascular resistance and pulmonary artery pressure and a tenfold rise in pulmonary blood flow. Birth-related stimuli, such as ventilation, increasing oxygen tension, and increasing shear stress serve to acutely increase NOS activity and NO production. Acute or chronic inhibition of NOS in fetal lambs produces pulmonary hypertension following delivery, indicating the fundamental importance of endogenous NO-cGMP signaling in the normal pulmonary vascular transition (Abman et al. 1990; Fineman et al. 1994). Additional data from human infants also indicate that sufficient supplies of the NOS substrate l-arginine are necessary for optimal NOS function during the neonatal period (Pearson et al. 2001).
The enzymes responsible for the downstream vascular responses to NO are also developmentally regulated. Soluble guanylate cyclase, which produces cGMP in response to NO activation, is expressed and active by late gestation (Bloch et al. 1997). Interestingly, the developmental patterns of PDE5 expression are not as clearly defined as for NOS and sGC. For instance, in rats, PDE5 expression and activity steadily increase through the end of gestation, peak shortly after birth, and then drop dramatically into adulthood. In contrast, in neonatal lambs, PDE5 activity and expression acutely decrease at birth and then rise again at 4–7 days of life (Farrow et al. 2010; Hanson et al. 1998a; Okogbule-Wonodi et al. 1998; Sanchez et al. 1998).
29.1.1.3 Dysregulation of Nitric Oxide Production and Response in the Diseased Respiratory System
29.1.1.3.1 General Concepts of NO-cGMP Dysregulation in Pulmonary Vascular Disease
Decreased expression of nitric oxide synthase and its downstream effectors, such as soluble guanylate cyclase and protein kinase G, contributes to vascular dysfunction in a number of pulmonary diseases. In addition, insufficient substrate or abnormal interactions with cofactors can lead to NOS dysfunction through “uncoupling” of the enzyme, even when protein levels are preserved. Furthermore, there is increasing awareness that a primary mechanism for the loss of bioavailable NO is its interaction with oxygen radicals such as superoxide. If levels of superoxide (O2·−) rise, NO can combine with it in a reaction which is diffusion limited and many times faster than dismutation of O2·− by superoxide dismutases (Beckman and Koppenol 1996). This reaction not only reduces bioavailable NO; it also produces the potent oxidant, peroxynitrite (ONOO−), which can nitrate target proteins, such as guanylate cyclase or K+ channels, preventing their activation by NO or other physiologic signals. Increased expression and/or activity of cGMP-phosphodiesterases can also disrupt NO-cGMP signaling, and recent data indicate that increased levels of reactive oxygen species rapidly activate PDE5 activity and expression (Farrow et al. 2008a, 2010, 2012).
29.1.1.3.2 Persistent Pulmonary Hypertension
Neonatal respiratory failure affects 2 % of all live births and is responsible for over one third of all neonatal mortality. Pulmonary hypertension complicates the course of more than 10 % of all neonates with respiratory failure, and the most severe PPHN has been estimated to occur in 2 per 1,000 of live-born term infants (Walsh-Sukys et al. 2000). Even with appropriate therapy, the mortality for moderate–severe PPHN remains just under 10 %, and up to 25 % of surviving infants have evidence for long-term neurodevelopmental disability (Steinhorn 2008, 2010).
PPHN occurs in association with a diverse group of illnesses but can be generally characterized as one of three types: (1) the abnormally constricted pulmonary vasculature due to lung parenchymal diseases such as meconium aspiration syndrome, respiratory distress syndrome, or pneumonia; (2) the lung with normal parenchyma and remodeled pulmonary vasculature, also known as idiopathic PPHN; or (3) the hypoplastic vasculature as seen in congenital diaphragmatic hernia. While “pure” idiopathic pulmonary hypertension occurs in the minority (~20–25 %) of infants with PPHN, severe cases are almost certainly affected by both parenchymal and vascular disease. Severe, early pulmonary vascular disease is believed to be the result of antenatal events that lead to significant pulmonary vascular remodeling in utero (Geggel and Reid 1984).
Because of the inherent difficulties in studying the pulmonary vasculature in the fetal and neonatal period, much of the knowledge about alterations in NO-cGMP signaling has been derived from animal models. Ligation or constriction of the fetal ductus arteriosus results in rapid antenatal remodeling of the pulmonary vasculature, which has been extensively studied in the neonatal lamb. After birth, ductal ligation lambs exhibit the increased fetal pulmonary artery pressure, severe hypoxemia, and high mortality, characteristic of human PPHN. Decreased expression of eNOS mRNA and protein and diminished eNOS activity have been documented in umbilical venous endothelial cells isolated from infants with PPHN as well as in pulmonary vascular tissue from the ductal ligation lamb model (Shaul et al. 1997; Villanueva et al. 1998). There is increasing evidence that reactive oxygen species produced by vascular NADPH oxidase and other sources likely contribute to suppressed NOS expression and may also interfere with NOS activity through inhibiting tetrahydrobiopterin synthesis (Brennan et al. 2003; Farrow et al. 2008b). Recent studies have confirmed decreased hsp90-eNOS association in PPHN lambs, which promotes “uncoupled” eNOS activity that could further amplify superoxide production (Konduri et al. 2007a). Expression and activity of vascular soluble guanylate cyclase are diminished in PPHN lambs (Steinhorn et al. 1995), and activity of PDE5 is increased (Farrow et al. 2010; Hanson et al. 1998b), both of which promote decreased cGMP concentrations (Tzao et al. 2001).
There is also mounting evidence that oxidant stress from mitochondria and NADPH oxidase isoforms plays a central role in the antenatal events that culminate in the PPHN phenotype (Afolayan et al. 2012; Wedgwood et al. 2013). New data raise the possibility that current therapeutic practices also play an important role in promoting postnatal oxidant stress, abnormal pulmonary vascular reactivity, and vascular remodeling. In particular, the use of high concentrations of therapeutic oxygen has recently become controversial in numerous settings. Hyperoxic ventilation is assumed to facilitate pulmonary vasodilation and thus remains a mainstay in the treatment of PPHN. However, surprisingly little is known about what oxygen concentrations will maximize benefits and minimize risk. The extreme hyperoxia routinely used in PPHN management may in fact be toxic to the developing lung by the formation of reactive oxygen species such as superoxide and reduced activity of endogenous antioxidants (Farrow et al. 2008b; Wedgwood et al. 2011). New data indicate that even brief periods of exposure to 100 % O2 increase reactivity of normal and remodeled pulmonary vessels (Lakshminrusimha et al. 2006a, 2007a, 2009a), diminish the response of the pulmonary vasculature to endogenous and exogenous nitric oxide (Lakshminrusimha et al. 2007b), and increase activity of cGMP-specific phosphodiesterases (Farrow et al. 2008a, 2010, 2012).
29.1.1.3.3 Congenital Diaphragmatic Hernia
Congenital diaphragmatic hernia (CDH) is a serious birth defect defined as abnormal development of the diaphragm. Severe CDH develops early in the course of lung development, and as a result, airway divisions, alveolarization, and vascular branching are significantly disrupted. Due to the complexity of their disease, mortality for infants with CDH remains very high (30–40 %) and has not changed significantly over the past few decades despite medical advances that have improved outcomes for other neonatal respiratory diseases.
Several investigators have utilized autopsy material to explore the role of NOS expression in infants that died of CDH, but results have yielded inconsistent answers (Shehata et al. 2006; Solari et al. 2003). Others have utilized animal models of CDH, including exposure of fetal rats to the herbicide nitrofen, and surgical creation of a diaphragmatic defect in fetal lambs. Results have again varied, with some groups showing decreased vs. normal eNOS expression in CDH. Recent data showed that treatment with exogenous NO enhanced lung branching and growth in lung explants from nitrofen-exposed rat fetuses, which suggests that even when NO expression is normal, NO production or bioavailability may be reduced (Muehlethaler et al. 2008). Abnormalities in downstream signaling mechanisms also occur, including decreased pulmonary artery relaxations to NO donors or cGMP analogues, possibly due to increased PDE5 expression or activity (Vukcevic et al. 2005).
29.1.1.3.4 Bronchopulmonary Dysplasia
In the United States alone, more than 10,000 babies develop bronchopulmonary dysplasia (BPD) each year, a serious disease characterized by persistent abnormalities of lung structure, including abnormal vascular growth and impaired alveolarization. Mice deficient in eNOS have abnormal lung architecture at birth, suggesting a critical role for NO in early lung development. New evidence indicates that impaired production of endogenous NO contributes to the pathogenesis of BPD, with multiple studies demonstrating that exhaled NO, as well as lung epithelial eNOS and nNOS expression, are decreased in models of BPD induced by prematurity (Afshar et al. 2003). Exposure of neonatal rats to hyperoxia produces alterations in lung alveolarization and vascularization similar to BPD. Increases in NOS expression have been observed in the hyperoxia model, although some have suggested that NOS dysfunction may contribute to its pathophysiology, perhaps due to inadequate substrate (Vadivel et al. 2010). Exogenous NO or sildenafil given during the recovery period improves growth of lung parenchyma and blood vessels, supporting this theory (Ladha et al. 2005; Lin et al. 2005).
29.1.1.3.5 Congenital Heart Disease
Infants with congenital heart lesions associated with increased pulmonary blood flow, such as truncus arteriosus or atrioventricular canal, will develop progressive vascular remodeling and secondary pulmonary hypertension. These children, as well as infants that undergo surgical correction in the first week of life, are at risk for significant postoperative pulmonary hypertension. A lamb model has been used to delineate how chronic increases in pulmonary blood flow after birth alter pulmonary vascular development. Aortopulmonary graft shunts are placed prior to birth in lambs, a model that mimics the human patterns of excessive postnatal pulmonary blood flow and produces slowly progressive changes in NO-cGMP signaling. By 4 weeks of age, eNOS mRNA and protein are increased, but NO-mediated dilation is diminished. This seems to be due in part to “uncoupling” of NOS, such that superoxide rather than NO is produced (Lakshminrusimha et al. 2007b; Steinhorn et al. 2001). By 4 weeks, PDE5 expression is also increased, representing another mechanism that may inhibit NO-cGMP-mediated vasodilation (Oishi et al. 2007). Acute changes in pulmonary vascular reactivity can arise following surgical correction of CHD, especially if functional or structural vascular responses to excessive pulmonary blood flow have occurred.
29.1.2 Inhaled Nitric Oxide
Inhaled nitric oxide (iNO), initially approved for clinical use in neonates with hypoxemic respiratory failure in 1999, has been one of the most significant advances in neonatal intensive care. Its use has now extended to other pediatric populations with pulmonary hypertension or other respiratory illness. Inhaled NO is well suited for the treatment of pulmonary hypertension: it is a rapid and potent vasodilator that can be delivered as inhalation therapy to airspaces approximating the pulmonary vascular bed. NO that reaches the blood stream binds avidly to hemoglobin, which further localizes its effect to the lung vasculature. Inhaled NO can also improve oxygenation by redirecting blood from poorly aerated or diseased lung regions to better-aerated distal air spaces, thus improving ventilation/perfusion matching. In contrast, intravenous dilators such as prostacyclin, tolazoline, and sodium nitroprusside often produce nonselective effects, leading to systemic hypotension, worsening ventilation/perfusion matching, and impaired oxygenation.
29.1.2.1 Delivery Methods and Interactions with Mechanical Ventilation
NO is a colorless and odorless gas; delivery systems must minimize contact of NO with oxygen to prevent formation of the toxic gas NO2. In early studies, nitric oxide was delivered by relatively simple two-stage regulators that introduced the gas into the circuit of continuous flow ventilators and measured inhaled concentrations of nitric oxide and its oxidative products at the endotracheal tube by chemiluminescence analyzers (Roberts et al. 1997). While inexpensive and relatively reliable, these methods lacked alarm systems to detect gas delivery outside the intended range. Currently, in most parts of the world, NO gas and delivery systems are provided by a single manufacturer (Ikaria Inc, Clinton, NJ). Available devices deliver consistent NO concentrations in the range of 0–80 ppm in a wide range of ventilator systems, including those that do not use continuous flow throughout the inspiratory/expiratory cycle. They also maintain continuous integrated monitoring using electrochemical NO/NO2 alarm sensors.
There are relatively few contraindications to iNO, which mainly consist of congenital heart disease with left ventricular outflow tract obstruction, such as interrupted aortic arch, critical aortic stenosis, and hypoplastic left heart syndrome. A relative contraindication is severe left ventricular dysfunction. These children are likely to have pulmonary venous hypertension due to high left atrial pressures, and use of iNO may aggravate pulmonary edema unless there is also optimization of cardiac performance.
The available evidence best supports the initiation of iNO at a dose of 20 ppm. Early studies in animals and infants used iNO doses as high as 80–100 ppm (Roberts et al. 1997). However, large clinical trials showed inhalation of higher iNO doses produced no better outcomes than lower doses of 20 ppm (Neonatal Inhaled Nitric Oxide Study Group 1997b), and sustained treatment with 80 ppm NO increased the risk of methemoglobinemia (Davidson et al. 1998). Pulmonary arterial pressure was measured in neonates with persistent pulmonary hypertension exposed to 0–40 ppm iNO, and revealed that optimal improvement in the pulmonary-to-systemic arterial pressure ratio was observed at an NO dose of 20 ppm (Tworetzky et al. 2001).
Severe parenchymal lung disease may be associated with poor responsiveness to iNO in newborns with PPHN. In newborns with severe lung disease, high-frequency oscillatory ventilation (HFOV) is frequently used to optimize lung inflation and minimize lung injury. The combination of HFOV with iNO often enhances the improvement in oxygenation in newborns with severe PPHN complicated by diffuse parenchymal lung disease and underinflation. A randomized, multicenter trial demonstrated that when PPHN was complicated by severe lung disease, response rates for HFOV + iNO were better than HFOV alone or iNO with conventional ventilation (Kinsella et al. 1997). Improved responses to combined treatment with HFOV + iNO likely reflect reduced intrapulmonary shunting as well as augmented NO delivery to its site of action.
During iNO therapy, adequate oxygen saturation and hemoglobin levels should be maintained to provide appropriate oxygen delivery. Once oxygenation and perfusion targets are achieved, a stable response to iNO should be followed by efforts to wean both iNO and FiO2. Inhaled NO can usually be easily weaned to 5 ppm within 24 h, and further weaning and discontinuation of iNO can generally be accomplished in 4–5 days.
Discontinuation of iNO is typically attempted once the FiO2 is weaned to <0.40–0.60 and the PaO2 is >60. Mild or transient decreases in PaO2 necessitating an increase in inspired oxygen are sometimes seen after discontinuation, but are generally well tolerated. However, stopping iNO can be complicated by “rebound pulmonary hypertension,” a more dramatic worsening of hypoxemia that responds only to reinstitution of iNO. Rebound pulmonary hypertension is more likely to be observed in infants exposed to higher iNO doses for several days and can usually be avoided by stepwise weaning to 1 ppm prior to discontinuation. Prolonged need for iNO therapy should lead to further evaluation to determine whether previously unsuspected anatomic lung or cardiovascular disease is present, such as pulmonary venous stenosis, alveolar capillary dysplasia, or severe lung hypoplasia.
A recent study evaluated the role of delivering iNO noninvasively, by nasal cannula, in newborns with prolonged pulmonary hypertension due to congenital diaphragmatic hernia (Kinsella et al. 2003). This study not only established the feasibility of this approach but also found that nasopharyngeal NO concentrations were approximately 50 % of the NO levels measured proximally in the delivery device. While development of a pulsed delivery system has been attempted, it has not reached broad-based use, which currently confines the use of iNO to the hospital setting.
29.1.2.2 Side Effects and Safety Issues
The reaction of NO with oxygen produces NO2, which is highly toxic to pulmonary epithelial cells. Current delivery devices measure NO2 continuously using electrochemical cells, so toxic levels can be avoided. After inhalation, NO diffuses into the bloodstream and rapidly reacts with oxyhemoglobin to form methemoglobin (metHb) and nitrate. Activity of methemoglobin reductase is often reduced in the neonatal period, and high levels of methemoglobin could aggravate hypoxia. Methemoglobin levels should be followed closely in the first few hours of therapy and then at 24-h intervals. Fortunately, NO2 levels higher than 2 ppm and methemoglobin levels higher than 5 % have generally been reported only in infants receiving high doses of iNO, in the range of 80 ppm (Davidson et al. 1998). An additional concern is that NO increases platelet cGMP, which inhibits platelet aggregation and could theoretically increase bleeding complications. While iNO increases bleeding time in healthy adults (Hogman et al. 1993), bleeding complications, including intracranial hemorrhage, have not been increased in infants treated with iNO.
Inhaled NO is usually delivered with high concentrations of oxygen. While hyperoxic ventilation is used to promote pulmonary vasodilation in PPHN, there is little to indicate that increasing oxygen concentrations beyond greater than 50–60 % further enhances pulmonary vasodilation in the normal or remodeled pulmonary circulation (Lakshminrusimha et al. 2007a, 2009a, 2011). High concentrations of oxygen may be toxic to the developing lung through formation of reactive oxygen species, such as superoxide anions (Lakshminrusimha et al. 2006b, 2011). Superoxide may combine with NO to form peroxynitrite (Lakshminrusimha et al. 2006b), a particularly potent oxidant with the potential to produce vasoconstriction, cytotoxicity, and damage to surfactant proteins and lipids.
One potential concern is that use of iNO could delay initiation of extracorporeal support (ECMO), which is the only therapy proven to decrease PPHN mortality. Fortunately, a recent review of registry data from the Extracorporeal Life Support Organization found that use of iNO did not significantly delay initiation of extracorporeal support (ECMO) or negatively influence survival in neonates that require ECMO (Fliman et al. 2006). However, in centers that do not have immediate availability of ECMO support, use of iNO in the neonatal period must be approached with caution. Inhaled NO cannot be abruptly discontinued because rebound pulmonary hypertension is likely (Davidson et al. 1998), therefore transport with iNO is needed if referral for ECMO is necessary. This capability should be determined in collaboration with the ECMO center before iNO treatment is started. The use of iNO with high-frequency ventilation creates particular problems for transport, and transport strategies should be considered before these therapies are combined in a non-ECMO center.
29.1.2.3 Indications and Outcome Analysis
29.1.2.3.1 PPHN
Large, multicenter placebo-controlled trials have established the safety and efficacy of iNO in neonates with severe persistent pulmonary hypertension, as defined by an oxygenation index (OI; calculated as (mean airway pressure × FiO2 × 100)/PaO2) of >25. These studies provided clear evidence that iNO acutely improves oxygenation and significantly decreases the need for ECMO support in newborns with diverse causes of hypoxemic respiratory failure and/or PPHN, as shown in Table 29.1 (Clark et al. 2000; Neonatal Inhaled Nitric Oxide Study Group 1997b). While these studies paved the way to FDA approval, it is equally important to note that iNO did not reduce the mortality, length of hospitalization, or the risk of significant neurodevelopmental impairment associated with PPHN (Clark et al. 2003; Neonatal Inhaled Nitric Oxide Study Group 2000). Moreover, a subsequent multicenter trial studied the potential benefit of beginning iNO at a milder or earlier point in the disease course (for an oxygenation index of 15–25). Early use of iNO did not decrease the incidence of ECMO and/or death, improve other patient outcomes, or reduce the incidence of severe neurodevelopmental impairment (Konduri et al. 2004b, 2007b).
Table 29.1
Summary of the large, multicenter randomized trials of inhaled NO in term newborns with hypoxemic respiratory failure and/or PPHN, showing the effect of iNO on ECMO utilization and mortality
% ECMO |
% Mortality | |||||
---|---|---|---|---|---|---|
n |
Initial OI |
Control |
iNO |
Control |
iNO | |
NINOS (1997b) |
235 |
44 |
55 |
39a |
17 |
14 |
Roberts et al. (1997) |
58 |
44.4 |
71 |
40a |
7 |
7 |
Clark et al. (2000) |
248 |
39 |
65 |
48a |
8 |
7 |
Davidson et al. (1998) |
155 |
24.7 |
34 |
22 |
2 |
8 |
Konduri et al. (2004b) |
299 |
19.2 |
12 |
10 |
9 |
7 |
These clinical trials also revealed that as many as 40 % of infants will not respond or sustain a response to iNO. The reasons for an inadequate response are diverse and require the clinician to carefully analyze the relative roles of parenchymal lung disease, pulmonary vascular disease, and cardiac dysfunction for each infant. For instance, if severe airspace disease is associated with PPHN, strategies such as high-frequency ventilation that optimize lung expansion are likely to be effective, and the two therapies used together are more effective than either used individually (Kinsella et al. 1997).
While initial case reports suggested that iNO improved oxygenation in infants with congenital diaphragmatic hernia, the above multicenter trials demonstrated that early administration of iNO did not reduce death or ECMO utilization in CDH (Neonatal Inhaled Nitric Oxide Study Group 1997a). However, data from the registry maintained by the Extracorporeal Life Support Organization suggest that iNO decreases cardiopulmonary arrest in infants with CDH that require ECMO (Fliman et al. 2006), which may improve later morbidity. Use of iNO in young, unstable infants with CDH should be accompanied by consideration for transfer to a center that can provide ECMO support.
29.1.2.3.2 Other Neonatal Respiratory Failure
Over the last decade, the use of iNO in the treatment of premature infants has been widely investigated as a preventative and rescue strategy. Early case reports of NO inhalation in premature newborns with severe respiratory failure demonstrated improvement in pulmonary hypertension, accompanied by marked improvement in oxygenation. Five large randomized, masked clinical trials focusing on iNO administration in preterm infants have now published their outcomes and include a wide range of patient populations, disease severity, and treatment strategies (Table 29.2).
Table 29.2
Randomized, masked trials of iNO for prematurity and respiratory failure
Patients enrolled |
Gestational age (weeks) |
Birth weight (g) |
Age at study entry |
Initial dose |
Duration of treatment |
BPD risk reduction | |
---|---|---|---|---|---|---|---|
Schreiber et al. (2003) |
207 |
27.2 |
983 |
13 h |
10 ppm |
7 days |
0.77 [0.6–0.98]a |
Van Meurs et al. (2005) |
420 |
26.0 |
838 |
26 h |
5 ⇒ 10 ppm |
76 h |
0.97 [0.86–1.06] |
Kinsella et al. (2006) |
793 |
25.6 |
792 |
30 h |
5 ppm |
14 days |
0.95 [0.87–1.03] |
EuNO (2010) |
800 |
26.5 |
~850 |
<26 h |
5 ppm |
21 days |
1.05 [0.78–1.43] |
582 |
26.0 |
763 |
16 days |
20 ppm |
24 days |
0.89 [0.78–0.99]a |
Three of the trials used an early, protective strategy, randomizing infants to receive placebo gas or iNO for 7–21 days (Kinsella et al. 2006; Mercier et al. 2010; Schreiber et al. 2003). The smallest, single-center trial showed that iNO significantly improved survival without BPD (Schreiber et al. 2003), but this finding was not confirmed in the two larger, multicenter trials. The trial conducted by Van Meurs et al. (2005) focused on an early rescue strategy by targeting infants with severe respiratory failure (mean oxygenation index of 22–23) and found that iNO did not reduce death or BPD. Furthermore, post hoc analysis of the 316 infants with birth weights of <1,000 g suggested the worrisome finding that iNO was associated with an increased risk of death and brain injury (grade 3 or 4 IVH or PVL).
Ballard et al. (2006a, b) studied the effects of iNO in older preterm infants still requiring mechanical ventilation, thus targeting a population at particularly high risk for BPD. In this trial, iNO was begun later (7–21 days), given at a higher dose (20 ppm, followed by stepwise weaning), and delivered for a longer time period (24 days). iNO administration resulted in a 23 % increase in survival without BPD at 36 weeks corrected gestational age. A follow-up study through 1 year of age showed that infants randomized to iNO were significantly less likely to require home oxygen or use bronchodilators, steroids, or diuretics after NICU discharge (Hibbs et al. 2008). Ballard’s findings raise important questions about the timing and potential reversibility of lung injury, as well as whether higher doses or longer exposures to iNO are significant factors in supporting lung development. In addition, post hoc analysis indicated that African American infants were more likely to respond than Caucasians, raising interesting questions about the effect of race and ethnicity on response to iNO.
All three trials will continue to report neurodevelopmental outcomes through at least age 5. However, an NIH consensus statement and an individual patient meta-analysis concluded that iNO does not produce consistent improvements in pulmonary outcomes, survival, and neurodevelopmental outcomes in premature infants with respiratory failure (Askie et al. 2011; Cole et al. 2011). Inhaled NO can be lifesaving for preterm babies with pulmonary hypertension that is not due to parenchymal lung disease, as seen following prolonged rupture of membranes and/or oligohydramnios (Aikio et al. 2012). However, this disorder affects only 2 % of all preterm babies, which would create a substantial barrier to the conduct of a randomized clinical study (Ball and Steinhorn 2012). Further study is also needed to address the potential role for iNO in preterm infants managed with noninvasive modalities such as CPAP.
29.1.2.3.3 Pediatric Respiratory Failure
In initial clinical studies of adult and pediatric patients with severe acute respiratory distress syndrome (ARDS), inhaled NO produced selective pulmonary vasodilation and improved systemic oxygenation (Rossaint et al. 1993). However, subsequent randomized controlled trials have not shown any beneficial effect on mortality or duration of mechanical ventilation in pediatric or adult patients (Dobyns et al. 1999; Sokol et al. 2003). While a transient improvement in oxygenation was observed in most of these studies, it has been speculated that since most patients dying from acute respiratory distress syndrome suffer from multiple organ systems failure, lung-selective therapy such as inhaled NO may not improve the overall survival rate (Coggins and Bloch 2007).
29.1.2.3.4 In Primary and Secondary PHT
While iNO would theoretically benefit children with chronic primary or secondary pulmonary hypertension, its practical application is limited by its expense, lack of home-based continuous delivery devices, and risk for rebound pulmonary hypertension. However, an important use of iNO has emerged for testing acute pulmonary vascular reactivity during cardiac catheterization. Inhaled NO has several advantages in this setting, including rapid onset of action and fewer potential adverse effects (e.g., systemic hypotension). For instance, Balzer et al. reported that the use of inhaled nitric oxide in combination with oxygen during preoperative evaluation allowed for identification of a greater number of appropriate candidates for corrective cardiac surgery or transplantation (Balzer et al. 2002). Others have shown that response to iNO during evaluation for pulmonary hypertension provides a safe, effective tool for predicting response to other agents such as calcium channel blockers and sildenafil.
One of the most common causes of secondary PHT in infants and children is bronchopulmonary dysplasia (Ivy et al. 2013; Mourani and Abman 2013). Mourani et al. demonstrated that the disease has a reactive component and that pulmonary pressures in patients with BPD improved to near-normal levels with acute exposure to iNO during cardiac catheterization (Mourani et al. 2004). However, the benefit of prolonged treatment with iNO in BPD-associated PH has not been well studied, mostly due to the logistical challenges of continuous long-term treatment.
Pulmonary hypertension after cardiopulmonary bypass can precipitate acute pulmonary hypertensive crises and contribute to excess morbidity and mortality after surgery for congenital heart disease. A number of small single-center studies indicate that iNO can attenuate pulmonary hypertension in at-risk postoperative patients, reduce the number of pulmonary hypertension crises, and shorten time on mechanical ventilation (Barr and Macrae 2010). In one larger randomized trial of pediatric patients, iNO (20 ppm) significantly decreased PVR and pulmonary hypertensive crises and shortened the time to extubation readiness (Miller et al. 2000).
Essentials to Remember
A complex set of enzymes including nitric oxide synthase, soluble guanylate cyclase, and phosphodiesterases control production of, and response to, nitric oxide in multiple lung cell types. Expression and activity of these enzymes change during fetal, neonatal, and pediatric development.
Persistent pulmonary hypertension of the newborn (PPHN) complicates the course of more than 10 % of all neonates with respiratory failure. Multiple abnormalities are now recognized in NO production and downstream signaling.
Inhaled nitric oxide (iNO) reduces need for extracorporeal support (ECMO) in PPHN but does not reduce mortality or improve long-term neurodevelopmental outcomes.
Several other pediatric diseases, including bronchopulmonary dysplasia and congenital heart disease, involve dysregulation of pulmonary NO-cGMP signaling pathways.
Inhaled nitric oxide is the optimal agent for assessing pulmonary vascular reactivity during cardiac catheterization. There may also be a role for iNO in the management of pulmonary hypertension after repair of congenital heart disease and for prevention of bronchopulmonary dysplasia in select populations of premature infants.
Poor responsiveness to iNO may result from inadequately treated parenchymal lung disease, poor cardiac function (particularly left ventricular dysfunction), or as a result of abnormal downstream signaling in remodeled pulmonary vessels.
Ventilation with high concentrations of oxygen may increase production of reactive oxygen species, such as superoxide anions, which can reduce vascular reactivity to iNO.
29.2 Nitric Oxide Pathway
Maurice Beghetti4
(4)
Pediatric Cardiology Unit, Department of Pediatrics, Children’s University Hospital Geneva, 6 rue Willy Donzé, Geneva 14, 1221, Switzerland
Educational Objectives
-
Describe the nitric oxide pathway and the potential therapeutic possibilities beyond inhaled nitric oxide.
-
Describe the current use of phosphodiesterase inhibitors.
The nitric oxide pathway is an important regulator in the cardiovascular system and is present in vascular cells and platelets. Impairments of the nitric oxide/sGC/cGMP pathway provoke acute and chronic vascular injury, including endothelial dysfunction, vasoconstriction, and vascular remodeling in the pulmonary circulation. Inhaled nitric oxide produces selective pulmonary vasodilatation in well-ventilated lung regions by stimulating sGC and increasing cGMP in pulmonary vascular smooth muscle cells. However, the mode of delivery through inhalation as well as the very short half-life of inhaled NO led to the search of other mode of increasing cGMP levels and promoting pulmonary vasodilation and inhibition of smooth muscle cells proliferation.
Phosphodiesterase type 5 inhibitors, such as sildenafil and tadalafil, prevent the breakdown of cyclic GMP thereby raising cyclic GMP levels resulting in pulmonary vasodilation. Soluble guanylate cyclase stimulators may offer a new target for therapeutic intervention in pulmonary arterial hypertension by producing cGMP independently of nitric oxide.
29.2.1 Nitric Oxide Pathway
It has been well recognized that nitric oxide (NO) plays a major role in the cardiovascular system. The NO pathway is an important regulator in the cardiovascular system and is present in vascular cells and platelets. Endothelial cell-derived NO, which acts in a paracrine fashion, is the endogenous stimulus of the soluble guanylate cyclase (sGC) (Fig. 29.2).
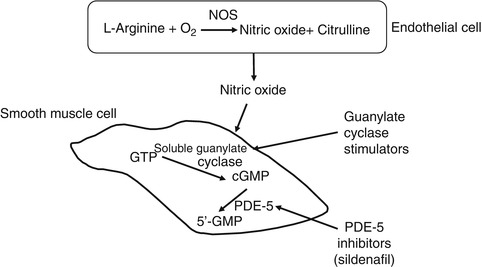
Fig. 29.2
Nitric oxide pathway. Abbreviations: NOS nitric oxide synthase, PDE-5 phosphodiesterase inhibitors
In the underlying smooth muscle cells and in platelets, NO activates the sGC, resulting in increased intracellular cyclic guanosine monophosphate (cGMP) levels, which induce vasorelaxation, inhibition of cell proliferation, and migration, by activating protein kinase C.
Impairments of the NO/sGC/cGMP pathway provoke acute and chronic vascular injury, including endothelial dysfunction, vasoconstriction, and vascular remodeling in the pulmonary circulation. As described before, inhalation of NO is used to treat subjects with pulmonary hypertension of various etiologies (Kinsella and Abman 2005). Inhaled NO produces selective pulmonary vasodilatation in well-ventilated lung regions by stimulating sGC and increasing cGMP in pulmonary vascular smooth muscle cells. cGMP is the second messenger that indeed regulates contractility of smooth muscle cells through the modulation of kinases, phosphodiesterases, and ion channels. However, the mode of delivery through inhalation as well as the very short half-life of inhaled NO led to the search of other mode of increasing cGMP levels and promoting pulmonary vasodilation and inhibition of smooth muscle cells proliferation (see Sect. 29.1).
29.2.2 Phosphodiesterase Inhibitors
Keeping cGMP levels high in the smooth muscle cells would be of interest to sustain pulmonary vasodilation and inhibition of proliferation, and this may be obtained through the inhibition of the enzyme that degrades cGMP, the phosphodiesterase 5(Ghofrani et al. 2004a). It has been shown that in pulmonary arterial hypertension (PAH), phosphodiesterase type 5 gene expression and activity are increased (Hanson et al. 1998b). Since the pulmonary vasculature is thought to contain substantial amount of PDE-5, the role of molecules acting on this enzyme is of major interest in pulmonary hypertension. Phosphodiesterase type 5 inhibitors prevent the breakdown of cyclic GMP thereby raising cyclic GMP levels resulting in pulmonary vasodilation (Croom and Curran 2008). All three PDE-5 inhibitors used in erectile dysfunction, such as sildenafil, tadalafil, and vardenafil, can cause significant pulmonary vasodilation (Ghofrani et al. 2004b).
Phosphodiesterase 5 inhibitors are as effective a pulmonary vasodilator as inhaled nitric oxide (Michelakis et al. 2002) and potentiate pulmonary vasodilation induced with nitric oxide (Abrams et al. 2000; Prasad et al. 2000). They may be particularly beneficial in conjunction with nitric oxide where withdrawal of nitric oxide may lead to rebound PAH (Atz et al. 2002; Namachivayam et al. 2006). In addition, phosphodiesterase type 5 inhibitors may be sometimes preferred because they do not increase pulmonary wedge pressure. However, in some settings, they can sometimes worsen oxygenation and induce significant systemic vasodilation (Stocker et al. 2003).
29.2.2.1 Sildenafil
A significant number of uncontrolled studies have shown beneficial effect of sildenafil in diverse forms of PAH (Garg 2008; Mourani et al. 2009; Hrometz and Shields 2006; Saygili et al. 2004; Vida et al. 2007; Humpl et al. 2005; Noori et al. 2007). Sildenafil was indeed the first drug of this class and is still the most commonly used, particularly in young children with pulmonary arterial hypertension. Sildenafil can be used orally or intravenously but is currently only available orally. The efficacy of sildenafil has been shown to be the same when compared to nitric oxide on pre- and postoperative patients with elevated pulmonary vascular resistance undergoing cardiac surgery for congenital heart defects (Schulze-Neick et al. 2003). In addition to iNO, sildenafil has been shown to reduce significantly pulmonary artery pressure with no significant effect on systemic arterial or central venous pressure in children with PAH following cardiac surgery. But this remains controversial with others having shown systemic effects as well as VQ mismatch changes (Stocker et al. 2003). No significant dose effect was seen, a 0.5 mg/kg/dose every 4 h being as efficacious compared to a 2 mg/kg/dose (Raja et al. 2007). In neonates with PPHN associated with a congenital diaphragmatic hernia resistant to inhaled NO, sildenafil has been shown to improve cardiac output by reducing pulmonary hypertension (Noori et al. 2007). Recently, in a study assessing the effect of intravenous sildenafil in neonates with persistent pulmonary hypertension, it has been reported that intravenous sildenafil was well tolerated and induced acute and sustained improvements in oxygenation at the higher dose studied. In children with PAH, sildenafil has been shown to improve saturation and exercise capacity without significant side effects (Karatza et al. 2005). Moreover, phosphodiesterase type 5 appears to be highly expressed in the hypertrophied human right ventricle, and acute inhibition of phosphodiesterase type 5 with oral sildenafil has been shown to improve right ventricular contractility (Nagendran et al. 2007). The most relevant side effects of sildenafil include erections and systemic hypotension. The dose administered is 0.5–1 mg/kg/dose given three to four times a day, but some unpublished data suggest the need to raise to six times a day. While sildenafil has been approved for the treatment of functional class II–IV PAH adult patients, the data in children remain limited. Preliminary safety and efficacy trials are under way in pediatric patients, and results should be available very soon. Addition of sildenafil to long-term intravenous epoprostenol therapy in patients with PAH has been shown to be beneficial (Simonneau et al. 2008). Some reports have shown some synergistic effects also with prostanoids; this may be due to potential inhibition of phosphodiesterase type 3 induced by a cross talk with PDE5 (Ghofrani et al. 2003).
29.2.2.2 Tadalafil
Tadalafil is also a selective phosphodiesterase type 5 inhibitor with a longer duration of action (Affuso et al. 2006). Few data are available in children, but tadalafil has been shown to improve oxygenation in an animal model of newborn PAH (Tessler et al. 2008). In adults with severe PAH resistant to prostacyclin therapy, tadalafil has been used in addition to prostacyclin with some improvement in the functional class (Bendayan et al. 2008). The results of a randomized controlled adult trials (PHIRST-1) have shown beneficial effects on exercise capacity, symptoms, hemodynamics, and time to clinical worsening (Galie et al. 2009). The side effect profile is similar to sildenafil. The dose administered is 5, 10, 20, or 40 mg once daily. However, even if the drug has recently been approved for chronic treatment of PAH in adults, so far data on acute pulmonary hypertension and in pediatric patients are very limited.
29.2.3 Guanylate Cyclase Stimulators
Alterations of the nitric oxide receptor, soluble guanylate cyclase (sGC), may contribute to the pathophysiology of PAH (Fig. 29.2). Expression and activity of sGC are abnormal in animal models of persistent pulmonary hypertension of the newborn, and this may lead to abnormal response to administration of nitric oxide (Deruelle et al. 2005). Direct pharmacological stimulation of sGC, either alone or in combination with NO, may provide a novel approach for the treatment of pulmonary hypertension (PH). Moreover, in the presence of NO, it seems to enhance the effects of NO.
BAY 63-2521, also called now riociguat, is a novel, orally available compound that directly stimulates sGC and sensitizes it to its physiological stimulator, nitric oxide (Mittendorf et al. 2009; Evgenov et al. 2006). Upregulation of soluble guanylate cyclase in pulmonary arterial smooth muscle cells has been noted in human idiopathic PAH lungs (Schermuly et al. 2008). Stimulation of sGC may reverse right heart hypertrophy and structural lung vascular remodeling.
In a rat model of monocrotaline-induced pulmonary hypertension, chronic treatment with BAY 63-2521 significantly decreased RV/(LV + S) ratio, resulted in a significant reduction of fully muscularized arteries as compared to both monocrotaline groups, and increased the percentage of non-muscularized pulmonary arteries (Schermuly et al. 2008). Recently another guanylate cyclase stimulator, cinaciguat, has shown beneficial effects in a model of neonatal pulmonary hypertension (Chester et al. 2009).
The first results in adult patients have recently been reported showing that the drug was well tolerated and that it was superior to NO in efficacy and duration (Grimminger et al. 2009). However, it must be noted that the drug has significant systemic effects, and this may be taken into account in the future.
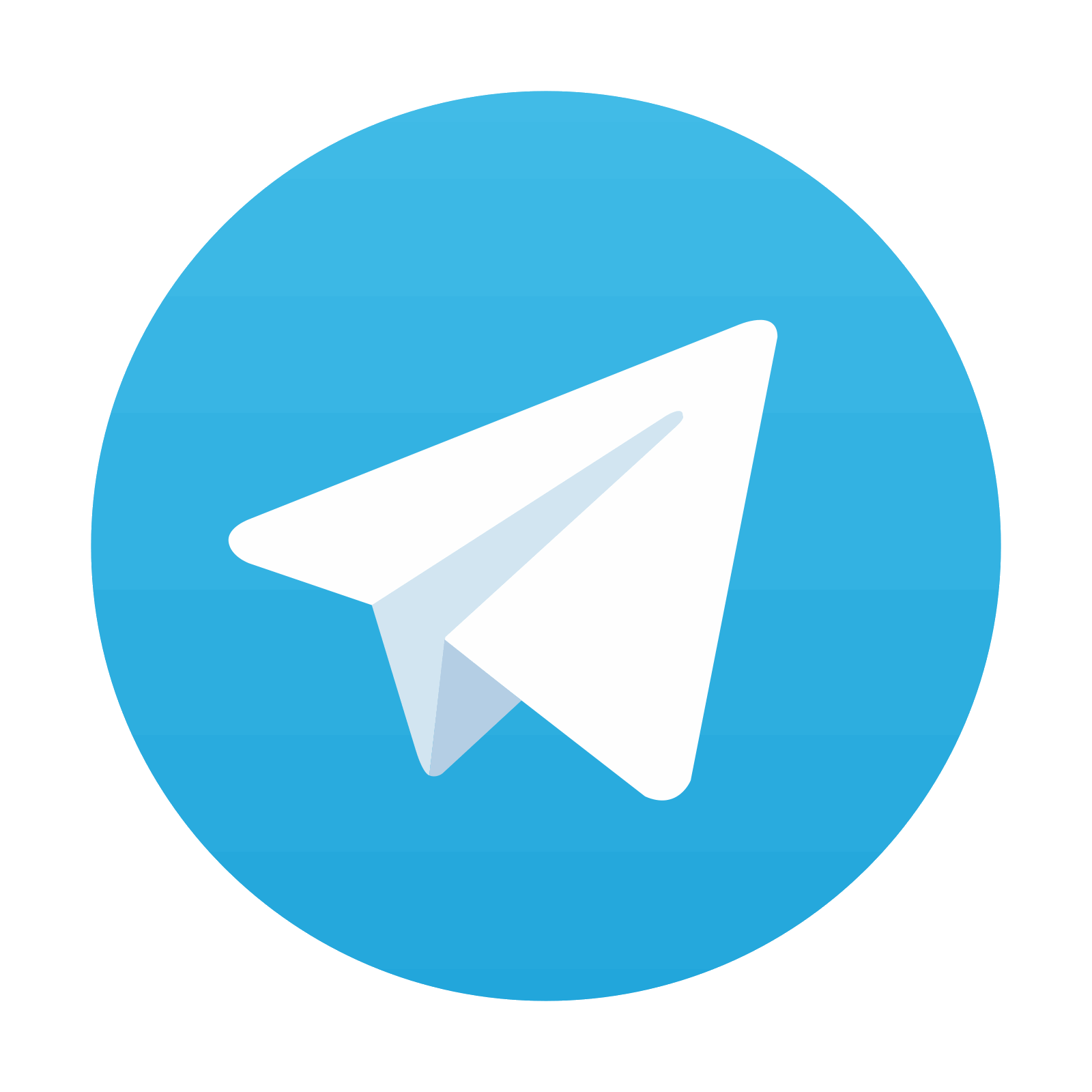
Stay updated, free articles. Join our Telegram channel
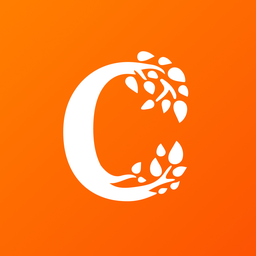
Full access? Get Clinical Tree
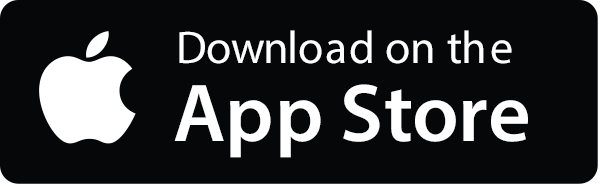
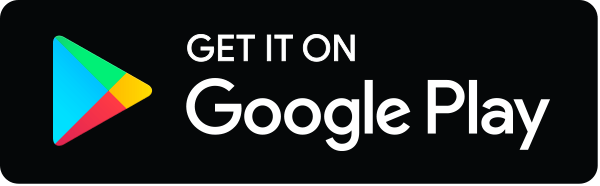