One of the critical early steps in the development of the central nervous system (CNS) is the closure of the neural tube and subsequent coverage by mesenchymal and epithelial components. Failure of these steps results in dorsal axis anomalies of the CNS (1). Furthermore, coverage of the CNS by mesenchymal and epithelial components is necessary for the development of the skull and vertebral column, which are in turn necessary for the protection of the CNS. It is not always clear if the main abnormality is one of actual neural tube closure, or a failure of the CNS coverings to properly form and contain the CNS. Factors external to the fetus, such as adherent amniotic membranes, can cause injury or malformation to the developing CNS.
Embryology of Neural Tube Closure and Bony Coverings
Early development of the CNS is addressed in Chapter 19. Additional details of neural tube closure are considered here because they are necessary for understanding these defects. The neural tube is classically thought of as beginning fusion at the rhombencephalon level and progressing zipper-like in the rostral and caudal directions with the closure of the respective neuropores on postfertilization days 30 and 31 respectively (2). This view was refined when it was shown that closure of the rostral neuropore was in fact bidirectional with another initiation point at the most rostral limit of the embryo (the “terminal lip” at the lamina terminalis). During Carnegie stage 11, closure proceeds caudally from the terminal lip and rostrally from the dorsal lip, with the two meeting dorsal to the lamina terminalis (3). In mice and chickens, it was shown that there is a third closure site over the hindbrain (4, 5). In order to explain the circumstantial evidence of a variety of human neural tube closure defects (e.g., anencephaly, occipital encephalocele, combinations of multiple defects), the multiple closure site hypothesis was extended to humans (6–9). This prompted re-evaluation of human embryos in the Carnegie collection by O’Rahilly and Muller, who maintained that “No convincing embryological evidence of a pattern of multiple sites of fusion … is available for the human” (10); this conclusion was accepted by investigators who examined embryos from the same collection (11) and by many authorities (4, 12). However, the examination of embryos from the Kyoto collection showed an “initiation site of [neural tube] closure … at the boundary between the mesencephalon and rhombencephalon (Site B), in addition to the first closure initiating at Site A at the prospective cervical level. Thus, it was confirmed that [neural tube] closure in human embryos does initiate at multiple sites” (13). Pigs have been shown to have two rhombencephalic closure sites, which have no identical counterpart in the mouse (14). It is therefore likely that the human neural tube has three closure sites, located at the anterior and posterior neuropores and in the nuchal region (Figure 37.1).
Figure 37.1 Diagrammatic representation of multisite neural tube closure model superimposed on to a photograph of a Carnegie stage 11 human embryo. Black triangles show the three closure initiation sites (A cervical; B mesencephalic-rhombencephalic boundary; C rostral tip of the neural groove). Arrows indicate the directions of neural tube closure toward the rostral/anterior neuropore (AN) and caudal/posterior neuropore (PN). Note the third closure site in the nuchal region between A and B. Based upon work by Nakatsu et al. and van Allen et al. (6, 13, 84).
Shortly after closure, the neural tube is entirely surrounded by mesoblasts and covered dorsally by skin ectoderm. Meningeal layers differentiate from the mesenchyme with the dura mater and arachnoid layers almost complete by the end of the embryonic period (15). During the 6th week, the skull begins to develop, consisting of the neurocranium, which encloses the brain, and the viscerocranium, which supports the face and upper airway. The neural crest supplies chondrocytes, osteoblasts, and osteocytes for intramembranous and endochondral bone formation (16). The cartilaginous portion of the neurocranium (chondrocranium) forms the skull base (17). Bones of the calvarium (i.e., frontal, parietal, and squamous temporal) develop from ossification centers in the surrounding mesenchyme beginning in the 8th week and extending radially outward becoming near complete except at sutures by 18–20 weeks gestation (18–20). In parallel, the vertebral bodies appear as loose mesenchymal structures between the dense intervertebral discs at 6 weeks, with cartilaginous differentiation in the 7th week and ossification beginning in the 9th week. The neural processes of the vertebrae encircle the spinal cord by 10 weeks at the cervical and thoracic levels, by 11–12 weeks at the lumbar level, and by 15 weeks at the sacral level (21).
Epidemiology and Risk Factors
Neural tube defects (NTD) are among the most common malformations of the CNS. In England and Wales in the 1960s, approximately 1.5/1000 births had anencephaly and a similar number had spina bifida. Terminations increased considerably in the 1970s reaching about 80% by the 2000s. At that time, the total incidence of NTDs had dropped by half to 1.6/1000 pregnancies (22). Similar declines have been demonstrated in other countries (23). However, European data from 1991 to 2011 indicate that the incidences of anencephaly and spina bifida have remained steady at approximately 3 and 4–5 (live births + terminations) per 10,000 pregnancies, respectively (24).
The molecular drivers of neural tube formation are complex (25) and the biology of NTD is not entirely understood (26, 27). Risk factors include maternal diabetes, maternal obesity, maternal hyperthermia, drugs, and toxins (e.g., valproate, ethanol), and inadequate maternal nutrition (1). In the 1980s, it became clear that low maternal folate level is a major risk factor for NTD (28). In 1992, the US Public Health Service recommended that all women at risk of becoming pregnant ingest 400 µg of folic acid daily. In 1998, the United States required fortification of enriched cereal grain products with folate (29). Folate supplementation of wheat flour and corn maize flour, to provide adequate maternal levels prior to conception, has reduced the incidence of total NTDs and anencephaly in North America by up to 50% (29). However, most nations in Europe, Africa, and Asia do not have mandatory food fortification and therefore rely on public education and voluntary self-administered vitamin supplements (see http://ffinetwork.org/regional_activity/americas.php) (30).
The underlying molecular role of folate in neural tube closure is unclear, likely because it is multifactorial. The folate one-carbon metabolism and methionine cycles, which interact through vitamin B12, regulate multiple metabolic pathways vital to cell survival, growth, proliferation, and differentiation (31). Not all NTDs have been eliminated by folate supplements. An additional genetic component clearly plays some role, but no chromosomal loci appear to have a major influence (32). However, targeted sequencing of candidate genes shows possible contributions to anencephaly by variants in PDGFRA (a growth factor receptor), GLDC (involved in folate metabolism), CELSR1 (involved in the control of planar cell polarity), and other genes (33).
Nomenclature and Pathology of NTDs
The pathology and nomenclature of NTDs depend on the rostral-caudal location and the involved tissue layers (CNS, meninges, bone, skin) of the lesion (Table 37.1). Defects in the skin and bone (“open” lesions) allow exposure of the meninges to the damaging effects of amniotic fluid (34, 35). Secondary damage to the CNS can complicate a primary failure of development. The significance of bone abnormalities alone depends on whether the CNS and meninges have herniated out of their normal confines. If they have done so, they are at risk of secondary mechanical injury. Cranial anomalies that involve the CNS include anencephaly, exencephaly, encephalocele, and acrania/acalvaria; the boundaries between these designations are not always clear. Spinal anomalies include the spectrum of spina bifida (bifid refers to a structure split into two parts) ranging from severe (complete rachischisis) to incidental minor anomalies (spina bifida occulta).
Table 37.1 Defects in the closure of the neural tube and coverings
NTD type | Embryologic level(s) involved | CNS primary defect | CNS secondary defect | Meninges defect | Bone defect | Skin defect |
---|---|---|---|---|---|---|
Nasal/frontal/orbital encephalocele | rostral (anterior) neuropore | + | +/- | +/- | + | +/- |
Nasal/frontal/orbital meningocele | rostral (anterior) neuropore | − | − | + | + | +/− |
Anencephaly | head | + | + | + | + | + |
Exencephaly (primary or secondary) | head | − | + | + | + | + |
Acrania/acalvaria | head | − | + | − | + | − |
Occipital encephalocele | nuchal closure region | +/- | +/- | |||
Occipital meningocele | nuchal closure region | − | − | + | + | +/− |
Agenesis of basioccipital bone | basi-occiput | − | − | − | + | − |
Rachischisis | nuchal closure region + spinal cord | + | + | + | + | + |
Myelomeningocele | caudal neuropore | + | +/− | +/− | + | + |
Spinal meningocele | spinal column | − | − | + | + | +/− |
Spina bifida occulta | lumbar spinal column | − | − | − | + | − |
Cranial NTD and Cranial Coverage Defects
Encephalocele is a herniation of the brain and meninges through a deficiency in the skull that may or may not be covered with skin. Nasal/frontal/ethmoidal/orbital encephaloceles and meningoceles, located near the anterior neuropore (see Figure 37.1), are designated according to their site and direction of herniation (Figure 37.2; 36–38). These must be distinguished from so-called nasal gliomas, which represent heterotopic brain tissue external to the meninges (39–43). Very rarely, lipomas arise in the vicinity of the lamina terminalis or corpus callosum; these might represent rests of mesenchymal cells trapped within the meninges at the time of closure (44).
Occipital encephaloceles and meningoceles are located in the vicinity of the nuchal closure site (see Figure 37.1). Depending on the exact location, they may contain the occipital lobe, cerebellum, or both (Figure 37.3; 45, 46). Some have postulated that a mesenchymal defect might be the primary anomaly in encephaloceles, in contrast to a primary problem with neural tube closure (47). CNS tissue within the encephalocele may be histologically normal, disorganized, or degenerate as a consequence of ischemia. If the skin is deficient, these lesions are subject to infection and therefore there may be considerable inflammation. Distant from the herniated tissue, distortions of the midbrain can occur. These may be associated with secondary changes in the cerebral aqueduct and consequent hydrocephalus. Because smaller occipital encephaloceles are compatible with life, they may be submitted as surgical resection specimens (36, 48). Occipital encephalocele may be a component of Meckel-Gruber syndrome, which also includes polydactyly and cystic enlargement of the kidneys; it is caused by mutations in genes that encode protein components of the primary cilium (49, 50).
Figure 37.3 Occipital encephalocele. A) In utero magnetic resonance image (T2-weighted using single-shot fast spin echo / SSFSE) at 23 weeks gestation showing fetus with occipital encephalocele (arrow). B) Same fetus after delivery at 24 weeks gestation. Skin on the encephalocele is partially ulcerated. C) Same fetus. Removal of skin and opening the meninges shows soft distorted brain tissue herniating through the occipital bone defect. D) Same fetus. Distorted cerebral aqueduct with hemosiderin in the tissue (arrow; hematoxylin and eosin (H&E) stain; 400x original magnification). There was mild hydrocephalus. E) Twenty-one week gestation fetus with small skin-covered encephalocele above the occipital protuberance. F) Hindbrain specimen from the same fetus (lateral view, after fixation) showing distorted cerebellum that had herniated (arrow). G) Photomicrograph showing horizontal slice of the same specimen. The herniated cerebellum is to the left and the narrow cerebral aqueduct to the right (arrow; H&E stain; 12.5x original magnification). H) Multiple small ependymal channels in aqueduct from the same case (H&E stain; 400x original magnification). I) Basal view of the brain from a 22-week gestation fetus with occipital meningocele. The cerebellum is slightly small but had not herniated. J) Lateral view showing leptomeningeal adhesions (arrow) to the cerebellum of a 37-week fetus with a hair-covered meningocele.
Anencephaly is characterized by an open defect in the scalp and calvarium such that the underlying cranial neural tube is exposed and damaged (Figure 37.4). These occur when closure does not occur in the region of the mesencephalic initiation site (see Figure 37.1). Anencephalic fetuses have abnormal notochords and cranial bases (51–55). The exposed brain is reduced to a flattened vascular mass (the “area cerebrovasculosa”) covering the skull base. Microscopic examination shows remnants of glial tissue, choroid plexus, leptomeninges with congested blood vessels. Portions of the lower brainstem may be relatively intact and the pituitary is atrophic (56, 57). The margins merge with surrounding skin, leading some to suggest that the primary defect is mesenchymal (58). While the brain may be malformed to some extent, the tissue typically available for examination reflects a destructive process, possibly as a consequence of exposure to the amniotic fluid or due to compromise of the arterial anatomy with subsequent ischemic damage (56, 59, 60).
Figure 37.4 Anencephaly. A) Posterior view of the head of a 20-week gestation fetus with anencephaly. B) Lateral view of the head of a 21-week gestation fetus with anencephaly. C) Top view of the head of a 27-week gestation fetus with anencephaly. Note the lobulated brain remnants on the skull base. D) Face view of a 40-week gestation fetus with anencephaly. Note that the eyes are of roughly normal size; this suggests that early prosencephalon development is normal. E) Midline sagittal section of the head of the same fetus. Note the vascular tissue along the skull base (arrow). F) Photomicrograph showing vascular leptomeninges and small fragments of the brain (arrow) from the middle fossa of an anencephalic fetus (H&E stain; 200x original magnification).
Exencephaly refers to herniation of the brain tissue outside of the normal confines of the cranium anywhere from the frontal to the occipital region (Figure 37.5; 61). Some have postulated that it is the embryologic predecessor of anencephaly; herniated brain tissue is subject to considerable degenerative change (62, 63). The main difference may be the size of the cranial defect. In some cases, exencephaly may be a secondary lesion, for example due to amniotic band adhesions (64–66). Amniotic band damage to the head can be associated with ischemic lesions in the brain, even when the calvarium is intact (Figure 37.5).
Figure 37.5 Exencephaly and amniotic band-associated brain damage. A) Large exencephalic protrusion in an 18-week fetus with complete acalvaria (face view). B) Small exencephalic protrusion in an 20-week fetus with calvarial defect confined to the posterior parasagittal region (posterior view). C) Large exencephalic protrusion in a 29-week fetus with amniotic band adhesions that were associated with complete calvarial defect (posterior view), a large omphalocele, missing left arm, and many constrictions on fingers and toes. D) Amniotic band damage to the face of a 30-week fetus with anencephaly. A deep cleft crosses the face and is associated with a cleft lip and abnormal eye (arrow). E) Face of a term infant with extensive damage secondary to amniotic bands. The calvarium was intact, but there was a cleft crossing the head (arrow). F) Coronal slice through the brain of the same infant. The brain had developed normally, but there was a large “porencephalic” cyst secondary to ischemic damage in the left middle cerebral artery distribution.
Acalvaria is the complete or partial absence of flat bones in the cranial vault (Figure 37.6). This can occur as an isolated phenomenon or as a component of a generalized bone disorder such as osteogenesis imperfecta. The scalp and meninges are intact, and the primary development of the brain is normal. In some cases, there may be only minimal brain abnormalities such as vascular congestion on the surface. Others may have surface hemorrhage or infarction as a consequence of vascular distortion and injury.
Figure 37.6 Acalvaria. A) Vertex view of the skull of a 22-week gestation fetus with generalized skeletal dysplasia and incomplete calvarial bone formation; black pen outlines the extent of firm skull. The underlying brain was normal. B) Face of a 23-week gestation fetus with osteogenesis imperfecta type 2 and absence of mineralization in the calvarium. C) Brain of the same infant showing subarachnoid hemorrhage. D) Skull X-ray of a 36-week gestation infant with trisomy 21 and absence of calvarial calcification. E) Lateral view of the head of the same infant after reflection of the scalp. The underlying brain was normal.
From the provided descriptions, it becomes apparent that the distinction between encephalocele, exencephaly, and anencephaly is not always clear. These disorders might simply represent a spectrum of anomalies with varied contributions by failures of neural tube closure and mesenchymal coverage, the manifestations of which are determined by the location or extent of the head that is involved (Figure 37.7).
Figure 37.7 Cranial coverage defect spectrum. Diagram illustrating the theoretical and potential spectrum of open and closed defects of the head. In acalvaria, the bone is deficient but the underlying meninges and brain are anatomically normal; the brain can be mechanically damaged (black granules). In meningocele, the bone defect is associated with meningeal herniation. In encephalocele, the brain also herniates and can undergo secondary damage. In exencephaly, the bone and scalp are deficient and the herniated brain is subject to injury. In anencephaly, the extent of deficient covering is greater (yellow = scalp; black = skull; red = meninges; green = brain).
Spinal NTD and Spinal Coverage Defects
Spina bifida broadly refers to vertebral defects with a split in the midline. The term dysraphism refers to malformations involving the dorsal surface of the embryo. Closed spinal dysraphism (“spina bifida occulta”) is characterized by a cleft in the vertebral column, without a corresponding skin defect and no exposure of neural tissue. Such cases may not be entirely normal; they may have mesenchymal abnormalities such as a skin dimple or hairy patch, lipoma (subcutaneous or intradural), or tethered spinal cord (67; Figure 37.8). Meningoceles are herniations of the meningeal coverings through a vertebral defect. The meningocele sac may be ballooned by filling with cerebrospinal fluid, but does not contain CNS tissue. Myelomeningocele is characterized by a cleft in the vertebral column, with a corresponding defect in the skin that exposes meninges and spinal cord to the external environment. This is also known as “open spinal dysraphism,” or “spina bifida aperta.” Such lesions are classified by the rostral-caudal location and the extent. The term “rachischisis” is reserved for complete or near-complete defects in the spine (Figure 37.8). When contiguous with features of anencephaly, this is called craniorachischisis (68, 69). Iniencephaly is a form of craniorachischisis wherein there is extreme retroflexion of the neck (Figure 37.8; 70). Similar retroflexion is often present in cases of cervical myelomeningocele.
Figure 37.8 Spinal neural tube defects. A) Hairy patch in the sacral area of a young adult with spina bifida occulta and scoliosis. B) Photomicrograph showing small intradural lipoma (arrow) associated with the filum terminale (H&E stain; original magnification 40x). C) Lumbosacral myelomeningocele in a 20-week gestation fetus. The meningeal sac is open so that dura covering the body of the vertebra is showing (arrow). D) Newborn infant with thoraco-lumbar myelomeningocele and large cyst. E) Section through decalcified lumbar spine of a 24-week fetus with lumbosacral myelomeningocele. F) Same case. Photomicrograph showing open meninges in continuity with skin (arrow; Masson trichrome stain; original magnification 12.5x). G) Cervical myelomeningocele in a 38-week fetus. H) Craniorachischisis (cervical-thoracic myelomeningocele + anencephaly) in a 26-week fetus. I) Lateral view of the same fetus showing extreme retroflexion of the neck (iniencephaly).
Myelomeningoceles distant from the cranium are often associated with intracranial abnormalities. The Arnold-Chiari (or Chiari type 2) malformation refers to caudal displacement of the cerebellar tonsils and medulla oblongata into the foramen magnum (Figure 37.9; 71). Hydrocephalus occurs in ~80% because CSF egress from the fourth ventricle is impaired by impaction of the posterior fossa contents (72). It has been debated for decades whether this represents part of the primary malformation (73) or a secondary effect caused by anchoring of the spinal dura and cord to the elongating vertebral column, which causes caudad traction on the brainstem (74). In favor of a secondary effect is the fact that in utero repair of the myelomeningocele is associated with a significant reduction in the incidence of hydrocephalus and hindbrain herniation (75).
Figure 37.9 Chiari type 2 deformity associated with myelomeningocele. A) Posterior view of the head (occipital bone removed) of a 20-week fetus with myelomeningocele and Chiari type 2 deformity. Note the lip (arrow; referred to as a “kink” by some writers) on the deformed medulla oblongata lying below the level of the foramen magnum (arrowhead). B) Lateral view of the brain from a 19-week fetus with lumbar myelomeningocele and Chiari type 2 deformity. Note the lip deformity of the brainstem (arrow). C) Brain and spinal cord specimen (posterior view) from a 22-week fetus with thoracic meningocele (not myelomeningocele) and associated cone-shaped deformation of caudal cerebellum (arrow) caused by impaction into the foramen magnum. D) Complementary specimen from the fetus’s twin that was not affected. Note the normal configuration of the cerebellum (arrow).
Autopsy for NTD
NTD cases are a significant component of many fetal and perinatal autopsy services, making up about half of the CNS malformations that will be encountered routinely (76–78). In a detailed examination of 340 specimens from missed abortions, 223 embryos/fetuses could be identified and analyzed. Among these, 37 had an NTD (16.6%), including 27 with encephalocele, 5 with anencephaly/exencephaly, 3 with spina bifida, 1 with caudal regression syndrome, and 1 with iniencephaly (79).
In simple NTD cases (i.e., anencephaly, spina bifida), the value of performing an autopsy is debatable. In elective terminations where the prenatal sonographic information is clear, parents might object to a complete internal examination. If the external findings are uncomplicated, the most common internal findings are adrenal, pituitary, and pulmonary hypoplasia, hydrocephalus, and urogenital abnormalities (80). Fewer cases have cardiovascular malformations (81). Autopsy provides additional useful information that is not provided by in utero sonogram (82). However, if fetal ultrasound and post-mortem MRI are concordant, conventional autopsy does not provide much additional information about the nature of the malformation (83).
When the pathologist examines these lesions, the most important matter is to document and distinguish all primary malformative phenomena (which might be important in diagnosis and genetic counseling) from secondary developmental and destructive changes. Histologic evaluation remains helpful in distinguishing subtle abnormalities.
References
Among the most dramatic malformations of the body and central nervous system (CNS) are those that involve abnormal lateral separation of the neural tube along its long axis. Often referred to as “monsters” in the old literature, these include the spectra of conjoined (or conjoint) twinning and holoprosencephaly. In the former, the body axis splits inappropriately, conceivably anywhere along the long axis, leading to duplication of body parts. In the latter, the prosencephalon fails to separate normally (Figure 38.1). Close embryologic connections between the brain and face dictate that many of these disorders have abnormalities of the face (Figure 38.2).
Figure 38.1 Diagram showing the basic midline pattern anomalies involving the CNS (dorsal views; rostral end at top). At Carnegie stage 11 (approximately 30 postfertilization days; top left) the neural tube exhibits three expanded regions (primary vesicles) at the rostral end (1 – prosencephalon, 2 – mesencephalon, and 3 – rhombencephalon). By Carnegie stage 14 (approximately 33 postfertilization days; top right), neural tube segmentation is associated with the appearance of five secondary brain vesicles – the prosencephalon is subdivided into the telencephalon (1a) and diencephalon (including retina, 1b), and the rhombencephalon is subdivided into the metencephalon (3a) and myelencephalon (3b). If the caudal neural tube divides at the time of caudal neural tube closure and onset of secondary neurulation (Carnegie stage 12), split cord deformities result (bottom-left). If the prosencephalon fails to differentiate bilaterally and split, holoprosencephaly is the result often with a fused, single midline eye. If the prosencephalon splits prematurely, duplications of the prosencephalon occur (diprosopus, from Greek, “two-faced”); the midline eyes are not always well developed.
Figure 38.2 Drawings showing the facial abnormalities associated with failed splitting and abnormal spitting of the prosencephalon. I to VI show the spectrum of holoprosencephaly ranging from cyclopia with rostral proboscis, to fused eyes, to mild hypotelorism. VII to IX are variants of normal. X to XIV show the spectrum of diprosopus from duplicated nose and hypertelorism, to midline third eye, to duplicated head.
Holoprosencephaly
Holoprosencephaly (HPE) is a disorder spectrum in which the prosencephalon fails to develop into two hemispheres. Basal forebrain areas that become established at Carnegie stage 14 (33 days postfertilization), including the olfactory region and optic stalks, are also underdeveloped (1). The range of brain abnormalities is encapsulated in the terms alobar, semilobar, and lobar HPE (Figures 38.3 and 38.4; 2). In alobar HPE, the hemispheres and ventricles are fused with no interhemispheric fissure and a horseshoe-shaped posterior rim. The basal nuclei are represented by an ill-defined central mass. Facial findings may include absence of the eyes, a single eye (cyclopia), fused eyes, or closely spaced eyes. A tubular proboscis above the eyes is present instead of a nose in the severe forms and cleft lip is common. In semilobar HPE the frontal lobes are fused and the interhemispheric fissure is only present in the parietal-occipital region. Facial anomalies include hypotelorism, microphthalmia, or anophthalmia. In lobar HPE the frontal lobes are fused but two distinct lateral ventricles are present. Facial anomalies are less pronounced but may include cleft lip, closely spaced eyes, or depressed nose. In the middle interhemispheric variant of HPE, the posterior frontal and parietal lobes are fused along the dorsal surface and the thalami may be fused, but the basal nuclei are usually separate. The architecture of the cortex, including gyration, may approach normal along lateral surfaces of the brain, but there tends to be some disorganization in the midline. The hippocampi are present but may be underdeveloped. Although the olfactory bulbs and tracts are absent or rudimentary in most cases of HPE, it is not clear if isolated olfactory agenesis is part of the HPE spectrum or a distinct entity (e.g., Kallmann syndrome; 3, 4).
Figure 38.3 Photographs showing the spectrum of facial abnormalities associated with holoprosencephaly (HPE). A) 22-week gestation fetus with trisomy 13 and alobar HPE. Note rudimentary proboscis above a single eye and absence of nose. B) 30-week gestation fetus with alobar HPE. Note large proboscis above a fused midline eyes and absence of nose. C) 24-week gestation fetus with semilobar HPE. Note single eyebrow, left side anophthalmia, absent nose, and cleft lip. D) 21-week gestation fetus with semilobar HPE. Note narrow spacing of eyes, and nose with single nostril. E) 22-week gestation fetus with semilobar HPE. Note wide spacing of eyes, absent nose, and cleft lip. F) 32-week gestation fetus with lobar HPE. Note wide spacing of eyes and broad nose.
Figure 38.4 Photographs showing the spectrum of brain abnormalities characteristic of holoprosencephaly (HPE). A) Alobar HPE (posterior view) in a 20-week gestation fetus. B) Semilobar HPE (posterior view) in a 21-week gestation fetus. C) Semilobar HPE (posterior view) in a 36-week gestation fetus. D) Semilobar HPE (coronal slice through frontal lobes) in a 23-week gestation fetus. E) Semilobar HPE (coronal slice through frontal lobes) in a 40-week gestation fetus. F) Semilobar HPE (coronal slice through frontal lobes) in a 1-year-old infant. Note the well-developed basal nuclei (arrows). G) Posterior view of semilobar HPE in a 21-week gestation fetus. H) Anterior view of the same case showing a large basal encephalocele (arrows). I) Lobar HPE (coronal slice through frontal lobes) in a 32-week gestation fetus. Note separation of the frontal horns of the lateral ventricles (arrows).
HPE is one of the most common brain malformations, with an incidence of approximately 1–2/10,000 births (including elective terminations; 5–9). However, evaluation of first trimester terminations and miscarriages in Japan suggests that the incidence of HPE is much higher (possibly 50/10,000 pregnancies; i.e., >5 times more common than neural tube defects), but that the likelihood of spontaneous early fetal loss is high (10, 11). Risk factors for nonsyndromic HPE include maternal diabetes, twinning, female sex, family history of a midline facial defect, and possibly maternal thyroid hormone supplementation or certain racial origins (6, 12).
Genetic causes are well established but not fully understood (13). Anomalies (mainly deletions) in at least 12 different chromosomal loci are associated with HPE in a range of syndromic forms (14). The four major causative genes (and their regulatory pathways) in nonsyndromic HPE include SHH, ZIC2, TGIF1, SIX3, and many others (15–19). Sonic hedgehog (Shh) protein is produced by the notochord and floor plate of the neural tube. The Shh signaling pathway is involved in ventral midline induction; failure of induction can result in HPE (20). Shh is also critical for maintaining Nkx2.1 (also known as “thyroid transcription factor 1”), a driver of interneuron differentiation in cells arising from the medial ganglionic eminence (21–23). In most fetal cases of HPE, Nkx2.1 is not detectable in the periventricular germinal cell populations by immunostaining, while it is preserved in lung and thyroid (Del Bigio, unpublished data). In syndromic HPE (e.g., trisomy 13, trisomy 18, Smith-Lemli-Opitz syndrome; 14), genetic abnormalities affecting the fibroblast growth factor (FGF) pathway (among others) are common (18).
Volpe, a neurologist, introduced the term “ventral induction” in reference to the interaction between the rostral neural tube and the ventral mesoderm, which lies between the neural tube and the notochord (24). The term has been used in the clinical literature concerning forebrain and face anomalies (25, 26). However, the term is seldom used in embryology (27, 28) and is not used in reference to the CNS as Volpe suggested, therefore it is best to avoid it.
Conjoined Twinning of Body Structures
Spencer proposed several mechanisms for the production of conjoined twins. Although she favored “secondary union of two originally separate embryonic discs” as the most likely explanation for situations where the bulk of the bodies exist separately with only focal sites of union (29, 30), fusion is not a satisfactory explanation for situations where only limited regions of the body are duplicated in the lateral plane. The partial fission hypothesis suggests that incomplete separation of the embryonic axis can lead to abnormally split structures (31–35). The molecular mechanism of body axis splitting is not known. Most cases are sporadic with no environmental causes or candidate genes identified. Mice with mutated Noto, a gene involved in caudal notochord development, may develop duplicated spinal cords (36).
The notochord is critical in the development of the body axis, along with regulation of the midline barrier and development of left-right asymmetry (37–39). The notochord appears in the human embryo at stages 11–12 (26 to 30 days post-fertilization), arising from the notochordal plate beginning at the trunk level and propagating in cranial and caudal directions (40). While the notochord is usually depicted as a cylindrical structure, it begins as a loose collection of cells that must converge (41). It is conceivable that failed convergence could create Y-formation notochords at either end of the body axis. This, in turn, is postulated to drive abnormal splitting of rostral and caudal neural structures and might be involved in other more subtle malformations such as duplication of the pituitary gland (42–45). Note that laterality defects are much more common in conjoined twins (46).
Split Prosencephalon
If the neural tube and surrounding mesencephalon undergo pathological mid-sagittal splitting early in embryogenesis, a range of duplications may result. Parapagus dicephalous twins have two discrete heads on a single body (33). If the split is confined to the prosencephalon, abnormal complete or partial duplication of the face and telencephalic structures can occur (e.g., diprosopus; Figure 38.5). Many case reports document the spectrum of these rare anomalies (47–49). Histologic characteristics in any given brain region are generally normal except near the sites of separation where there are non-specific features of disorganization (50). Unless the head is almost entirely duplicated, the midline eye(s) are usually not normal.
A) Fetus with an enlarged head and two nearly complete faces (“Janus” deformity), with the exception of a partially fused intermediate eye (arrow).
B) Brain of the same case showing complete duplication of the telencephalon and diencephalon (including two optic chiasms (arrows) branching from a single brainstem with rudimentary cerebellum.
C) Face of a 22-week fetus with duplicated nose and a rudimentary midline third orbit.
D) Ventral surface of the brain from the same fetus. The brainstem (bs) and slightly small cerebellum (ce) are situated between two asymmetric temporal lobes (tl). The frontal lobes (fl) are abnormally segmented with duplicated interhemispheric fissures (arrows) radiating in a Y pattern from the infundibulum. A third interhemispheric fissure runs in the midline (arrowhead).
Split Spinal Cord
The spinal cord develops from the neural tube caudal to the myelencephalon. The caudal neuropore at the lumbar level closes at Carnegie stage 12 (31 postfertilization days). The spinal cord at the sacral level develops by secondary neurulation, which continues to Carnegie stage 17 (40 postfertilization days; 1, 51–53). Split cord malformations are also referred to as diastematomyelia and diplomyelia, depending on whether the cord is split or duplicated (Figure 38.6). Like malformations of the prosencephalon, they exist as a spectrum. They are postulated to occur when adhesions between the ectodermal and endodermal layers of the embryonic plate condense and bisect the developing notochord (54). However, the caudal neural tube of human embryos has been shown to be duplicated in the presence of a single notochord (55). The split cord may be situated within a single or split dural sac, the latter often with a sagittal spur of vertebral bone (54, 56). The split cord is often asymmetric (57). Caudal duplication syndrome is designated when structures derived from the embryonic cloaca and notochord are duplicated to various extents (58, 59).
B) Same case. At the lower lumbar and sacral level, the spinal cord anatomy is duplicated within a single dural sac.
C) Apparently duplicated spinal cord within a single dural sac of a child with caudal duplication (from low thoracic level downward; sacral to right).
D) Same case. Histology shows the malformation is mainly splitting with only partial duplication of the spinal cord at the lower lumbar level. Solochrome cyanin (blue, myelin) and eosin stain.
Figure 38.6 Examples of split spinal cord.
References
Introduction
Hydrocephalus is defined as active distension of the brain’s ventricular system caused by the inadequate passage of cerebrospinal fluid (CSF) from its point of production to its point of absorption (1). It must be distinguished from the passive expansion of the ventricles secondary to the destruction of the brain (e.g., hydrocephalus ex vacuo or hydranencephaly). Although hydrocephalus can occur at any age, here we focus on the pathology in fetuses and infants (2). Enlargement of the cerebral ventricles is common, multifactorial, and non-specific. When identified on prenatal ultrasound, elective termination of a pregnancy may be recommended (3). Subtle enlargement of the ventricles is difficult to verify in brains of stillborn infants because the brains are very soft.
Anatomic Considerations
The cerebral ventricles (two symmetric lateral ventricles along with midline third and fourth ventricles) and the subarachnoid space (SAS) constitute the linked CSF spaces. During embryonic and early fetal life, the ventricles are lined by the ventricular (VZ) and subventricular (SVZ) germinal zones, which are replaced by ciliated ependymal cells. Most CSF is produced by the choroid plexus (~80%), a specialized ependyma-covered vascular structure. The remaining CSF comes from brain tissue via fluid flow through the extracellular compartments. The SAS is confined internally by cells of the pia mater (adjacent to the glia limitans at the brain surface) and externally by arachnoidal cells (adjacent to the subdural space and dura mater; 4). CSF is absorbed into venous sinuses via arachnoid villi, and into connective tissue lymphatics through the dura and along exit sites of cranial and spinal nerves (5, 6). The relative contribution of these reabsorption areas is not known; early in development lymphatic absorption might predominate (7).
Internal hydrocephalus is an enlargement of the cerebral ventricles (Figure 39.1), and external hydrocephalus is an enlargement of the SAS. Rekate considered almost all forms of hydrocephalus obstructive, with very rare exceptions being due to overproduction of CSF by choroid plexus papilloma. He offered a simple classification of hydrocephalus according to the anatomic site of CSF obstruction (1, 8). In this context, “congenital” hydrocephalus simply indicates that the insult occurred prior to birth and does not specify a particular pathology. Note that anatomic obstruction is not necessarily complete. Relative strictures can retard the pulsatile movement of CSF, which is driven by arterial pulsations (9). The resulting increase in pulse pressures is sufficient to cause ventricular enlargement (10). Immature fetal brains, which have higher water content and less cellular stability, are especially susceptible to distortion (11).
Figure 39.1 Photographs showing a 42-week stillborn fetus with severe internal hydrocephalus. The cranium is enlarged but the face is normal (left panel). Horizontal slice through the brain showing severe enlargement of the frontal and temporal horns of the lateral ventricles and severe thinning of the cerebral mantle (middle panel). Horizontal slices through midbrain (right upper panel) and pons (right lower panel) showing obliteration of the cerebral aqueduct (arrows).
Several anatomic sites are vulnerable to obstruction by virtue of their narrow geometry: the interventricular foramen (of Monro), the cerebral aqueduct (of Sylvius), and the SAS including the fourth ventricle outlets. Obstruction can be caused by a mass within the pathway, by an extrinsic abnormality that compresses the pathway, or by a pathologic process that causes obliteration of the pathway. The lumen of the entire ventricular system is normally lined by a single layer of columnar to cuboidal, ciliated ependymal cells. A thin layer of subependymal astroglial tissue covers the underlying gray and white matter structures. Where the ependyma is deficient, the astroglial layer is often slightly thickened (12).
Obstruction at the Interventricular Foramen
Obstruction at the foramen can cause unilateral or bilateral ventriculomegaly (13, 14). Any type of brain tumor that grows in the region of the anterior third ventricle can be causative (15). A colloid cyst is a benign lesion of the region; lined by a simple ciliated epithelium and filled with proteinaceous fluid, they likely arise from primitive endodermal rests rather than choroid plexus or ependyma (16–18).
Unilateral hydrocephalus associated with simple congenital atresia of the foramen is rare. When mild unilateral ventriculomegaly is identified in a fetus it often resolves spontaneously or is an ex vacuo phenomenon (19). Simple obliteration of the interventricular foramen is postulated to occur as a result of an inflammatory and /or reactive process (20). Relatively minor in utero infections are capable of damaging the ependymal lining of the ventricles (see Chapter 57), which predisposes to the fusion of adjacent tissue walls (12).
Obstruction at the Cerebral Aqueduct
The cerebral aqueduct in the midbrain connects the third and fourth ventricles. It is approximately 12 mm long at term birth and extends to a mature length of 18 mm by 1 year. Its profile varies by location, being slit-like at the rostral end, ovoid or diamond-shaped in the middle, and triangular at the caudal end. The smallest diameter is about one quarter of the way from the third ventricle (21). The cerebral aqueduct is the most common location of obstruction to CSF flow (22). Obstruction of the cerebral aqueduct can occur in a broad range of pathological processes (23; Figure 39.2).
Figure 39.2 Photomicrographs showing cerebral aqueducts in a range of obstructed forms (all hematoxylin & eosin stain, 40x original magnification). A) Normal scalloped aqueduct in the brain of a 21-week gestation fetus. B) Aqueduct compressed by abnormal veins in an infant with hydrocephalus. C) Multiple small ependymal channels in the midbrain of a holoprosencephalic fetus. D) Scarred aqueduct following bacterial ventriculitis in an infant. Ependymal channels are buried within reactive astroglial tissue. E) Aqueduct compressed by nodular heterotopia in a 24-week fetus with hydrocephalus. The underlying cause was not known, but was suspected to be in utero infection. F) Aqueduct with complete obstruction caused by clotted blood in a fetus with cryptic intraventricular hemorrhage and hydrocephalus (47).
In the category of congenital obstruction, many adjectives are used to describe the aqueductal morphology. Russell proposed a distinction between stenosis (abnormally small single channel with normal ependyma), forking (multiple tiny ependyma-lined channels), gliosis (“proliferation of the subependymal glia and disruption of the ependyma”), and septated; she advised against the term “atresia” (24). These distinctions have been perpetuated for decades (22, 25). However, it is not clear that they are useful from a pathophysiologic perspective. Russell’s own illustrations reveal negligible differences between forking, gliosis, and “maximum stenosis.” Parker and Kernohan wrote, “Chronic ependymitis, periaqueductal gliosis and congenital narrowing of the aqueduct represent minutiae, much argued over but little comprehended. … One type may grade imperceptibly into the other, and dogmatism as to the type is out of place” (26). We agree that these abnormalities likely reflect a spectrum rather than discrete pathologies.
The pathogenesis of aqueductal narrowing has been deliberated, without a solution, for decades. Many authors have hollowly considered a distinction between developmental, congenital, and inflammatory origins (24, 27, 28). Ultimately, in many cases, one can only speculate. The ependymal lining of the ventricular system appears to be important for maintaining patency. Genetic mutations that result in disrupted ependymal cell junctional complexes cause sloughing of ependymal cells and secondary aqueduct stenosis in experimental mice (29–32). Homologous mutants have not yet been revealed in humans. X-linked hydrocephalus associated with mutations in L1 cell adhesion molecule (L1CAM) is associated with brain malformation and abnormalities of neuron migration. Most cases have aqueduct stenosis, but they do not have widespread abnormalities of the ependyma (33; Figure 39.3). Ependymal cilia appear to stir CSF near the ventricle walls and to move cell debris, but they do not create bulk flow (34). Many mutant mouse strains with ciliary abnormalities develop hydrocephalus, but most do not have aqueduct stenosis (35). The hy-3 mouse with a mutation in Hydin develops severe aqueduct stenosis (36), but no human with a HYDIN mutation has developed hydrocephalus (37). Hydrocephalus has only rarely been reported in children with proven structural abnormalities of the cilia (35, 38, 39). The single autopsy examination of such a case did not show aqueduct stenosis (12, 40). To recapitulate, a causal relationship between motility defects in cilia and hydrocephalus remains unclear.
Figure 39.3 X-linked hydrocephalus in a 23-week fetus. A – Photograph shows a large head and micrognathia. B – Photograph shows an adducted thumb (arrow). C – Photograph of the medial surface of the cerebral hemisphere shows a thin cerebral mantle and absence of corpus callosum (arrow). D – Photographs show a distended aqueduct in the upper midbrain and aqueduct stenosis in the lower midbrain (arrow).
The central canal of the spinal cord is histologically similar to the cerebral aqueduct. Asymptomatic collapse of the central canal occurs in almost all people during childhood and teenage years; the leading hypothesis for this process is minor infection with common viruses (41). Focal fusion of adjacent ependymal surfaces is commonly observed in patent aqueducts (42, 43). Therefore, it is possible that maternal viral infections (for example, influenza; 44) during the fetal period could damage the ependyma and lead to abnormalities in the cerebral aqueduct (12; see Chapter 57; Figure 39.4).
Figure 39.4 Suspected post-infectious hydrocephalus in a 21-week fetus. A definite cause was not proven. A) Photograph showing a large head, abnormal hand positions, and club feet. B) Photograph showing a coronal slice of one cerebral hemisphere with an enlarged lateral ventricle. C) Photomicrograph showing obliteration of the cerebral aqueduct with rare small ependymal channels (arrow) and dispersed ependymal cells. Hematoxylin and eosin stain; original magnification 40x. D) Photomicrograph showing lower pons / upper medulla with an irregular fourth ventricle and multiple foci of calcification (arrows). Hematoxylin and eosin stain; original magnification 12.5x. E) Photomicrograph showing calcification in the medulla (black). Von Kossa stain; original magnification 100x. F) Photomicrograph showing reactive microglia and rare lymphocytes (arrow) immunostained for CD45 (brown; with hematoxylin counterstain). Original magnification 200x.
Other types of aqueduct damage are more easily explained. Intraventricular hemorrhage (IVH) following premature birth is a well-known cause, although CSF obstruction at the fourth ventricle and SAS can also occur in this context (45). Rarely, in utero IVH leads to hydrocephalus (46). In some cases, the hemorrhagic site cannot be identified and hemosiderin within the fused aqueduct is the only evidence (47; Figure 39.2). Small vascular malformations in the midbrain can compress the aqueduct leading to hydrocephalus in children or adults; most are demonstrated angiographically (48; Figure 39.2).
Obstruction within the Fourth Ventricle or at the Apertures
The fourth ventricle is a pyramid-shaped space between the cerebellum and the brain stem (49). CSF enters proximally from the cerebral aqueduct and leaves through the lateral (foramina of Luschka) and median (foramen of Magendie) apertures into the SAS. Blood from supratentorial intraventricular hemorrhage (IVH) can pass into the fourth ventricle (45). Post-hemorrhagic hydrocephalus occurs in ~9% of preterm infants with IVH (50). In the acute phase, a blood clot can cause a physical obstruction to CSF flow. In the following weeks, an inflammatory reaction can cause fibrosis and scarring of the fourth ventricle outlets (Figure 39.5).
A) The child was born at 24 weeks gestation and died 1 day after severe (grade 4) intraventricular hemorrhage. The fourth ventricle and its outlets are occluded by a blood clot, and there is associated subarachnoid hemorrhage (arrow). Hematoxylin and eosin stain; 12.5x original magnification.
B) The child was born at 31 weeks gestation and died 13 weeks after grade 4 intraventricular hemorrhage The fourth ventricle apertures are occluded by collagenous, hypervascular scar tissue (arrows). Masson trichrome stain, 100x original magnification.
Medulloblastoma, pilocytic astrocytoma, and ependymoma are common cerebellar tumors of childhood (51) that can cause hydrocephalus by obstruction of the fourth ventricle. Diffuse midline glioma, in the past often called diffuse intrinsic pontine glioma (DIPG), accounts for ~10% of pediatric brain tumors (52, 53) and may cause obstruction of the fourth ventricle or aqueduct. Pineal gland tumors or cysts are rare lesions that can cause extrinsic aqueduct compression (54, 55).
The Chiari malformation type 2 (also called Arnold-Chiari malformation) comprises herniation of the inferior cerebellar vermis and tonsils through the foramen magnum in association with myelomeningocele (56, 57; Figure 39.6). Among aborted fetuses with spina bifida, approximately half have enlarged ventricles (58). Among children born with myelomeningocele, approximately 80% will develop hydrocephalus (59). The cause of hydrocephalus in this circumstance has been the subject of debate. Impaction of the cerebellum against the brainstem with compression of the fourth ventricle and functional occlusion of the fourth ventricle apertures likely occurs in most (60–62). Among 100 autopsy cases of children (newborn to five years) with myelomeningocele and hydrocephalus, the cerebral aqueduct was patent in all cases (63). In smaller studies of affected infants, significant minorities had forking or narrowing of the cerebral aqueduct (64–66). In our experience, most brains from fetuses with myelomeningocele have a normal cerebral aqueduct. Intrauterine repair of the myelomeningocele significantly reduces the likelihood of shunt-dependent hydrocephalus, suggesting that hydrocephalus is indeed secondary to the spinal anomaly (67). It remains to be determined if changes in the aqueduct lumen are a primary component of the neural tube closure defect (68), or a secondary phenomenon due to distortion of the hindbrain.
B) Midsagittal slice through the head of a 20-week gestation fetus with myelomeningocele. The low set, deformed cerebellar tonsils are shown (arrow).
Figure 39.6 Chiari type 2 malformations.
Hydrocephalus is associated with a range of other posterior fossa malformations. The fourth ventricle and the cisterna magna are normally in free communication (69). The Dandy-Walker malformation, defined as hypoplasia of the cerebellar vermis with cystic dilation of the fourth ventricle due to atresia of the fourth ventricle apertures, is usually associated with hydrocephalus (70–72; Figure 39.7). This may rarely be a component of Meckel syndrome type 1 (also known as Meckel-Gruber syndrome; 73–75). Occipital encephaloceles, characterized by herniation of the cerebellar or occipital lobe, are often associated with hydrocephalus (76–78). Usually, the aqueduct is normal, although we have seen cases with distorted midbrain and secondarily obliterated aqueduct.
A) Photograph showing back of child’s head with the occipital bone removed to reveal the narrow, flattened cerebellar hemispheres surrounding a large midline cyst (arrow).
B) Photograph showing the hindbrain after removal and fixation. The midline cyst remains obvious. A piece of the attached scalp (arrow) is adherent to an encephalocele that contains a herniated piece of the cerebellum.
C) Photograph showing a horizontal slice through the pons and upper cerebellum. The vermis is deficient although there are midline adhesions of the leptomeninges (arrow).
Obstruction in the Subarachnoid Space and Absorptive Sites
From the fourth ventricle, CSF moves into the cranial and spinal SAS and eventually to the sites of absorption. The SAS is a generally very narrow space situated between the arachnoid mater and the pia mater, with several large expansion (cisterns) on the ventral surface of the brain. Major blood vessels, cranial nerves, and spinal nerves all pass through the SAS (79–81). CSF is resorbed from the SAS through several routes. The best studied are the arachnoid villi and granulations that penetrate into the venous sinuses and radicular veins (80, 82–84). In experimental animals, up to 50% of CSF is absorbed into cranial (especially nasal) lymphatics along nerve rootlets, with relatively greater importance in young animals compared to adults (7). There is some support for this in humans (5, 85). The dura mater itself also contains pathways that connect with the cranial lymphatics or venous sinuses in humans (6, 86–88).
A broad range of pathological processes can cause scarring of the SAS, with the subsequent impairment of CSF flow. Hemorrhage and infection are the most common (45, 89). In the subacute and chronic phase, inflammatory cells release mediators that activate fibroblasts and endothelial cells to cause fibrosis (4, 90).
It is not clear if any developmental anomalies specifically and directly affect the SAS or absorption sites sufficiently to cause hydrocephalus. Four case reports from the 1970s apparently documented congenital absence of arachnoid villi in hydrocephalic children (91, 92); however, none were well documented. It is not clear if this condition is real but not reported, or if no one has identified more convincing evidence. The diminishing frequency of autopsies may have prevented the recognition of such cases.
Venous hypertension can decrease CSF absorption by altering the pressure gradient across arachnoid villi (93). In infants and young children, this may be associated with hydrocephalus (94). Achondroplasia and other forms of complex craniosynostosis might also cause elevated venous and intracranial pressure (95, 96). Cerebral venous hypertension can be the result of superior vena cava occlusion (97) or congenital heart malformation (98). We have seen a few cases at autopsy in which diffuse venous congestion and mild ventriculomegaly are the only abnormalities.
The pathophysiologic causes of hydrocephalus are summarized in Table 39.1.
Category | Specific causes | Mechanism of CSF pathway obstruction |
---|---|---|
Hemorrhage | In utero intraventricular (large or cryptic); prematurity-associated intraventricular; choroid plexus; brain trauma (birth-related or post-natal) | Mechanical obstruction by blood clot; scarring secondary to inflammation |
Infection | Ventriculitis (resolved cases may be suspected if inflammation and/or calcification evident | Ependymal loss and periventricular tissue damage secondary to inflammation |
Meningitis | Scarring/fibrosis of subarachnoid space secondary to inflammation | |
Malformation / genetic | Myelomeningocele + Chiari 2 deformity | Mechanical compression of fourth ventricle outlets |
Ciliopathy; L1-CAM mutation; occipital encephalocele; Dandy-Walker; VATER-H; Meckel-Gruber; et al. | Various, often unclear; cerebral aqueduct may be abnormal | |
Neoplasm | Diffuse midline glioma; ependymoma; medulloblastoma; pilocytic astrocytoma; pinealoma; et al. | Infiltration or compression of CSF pathway |
Cyst | Colloid cyst | Mechanical obstruction at interventricular foramen |
Venous pressure | Cardiac malformation; obstruction superior vena cava | Altered pressure dynamics at absorption sites |
Effects of Hydrocephalus on the Brain
In addition to brain damage associated with the primary disease process (e.g., meningitis, malformation, hemorrhage), enlargement of the cerebral ventricles can cause secondary damage to the surrounding brain tissue – in particular, axons in the periventricular white matter (Table 39.2; 99–105). Mechanical distortion can stretch axons and can compress periventricular blood vessels leading to impaired blood flow and secondary damage to axons (106). Retrograde and anterograde phenomena can alter neuron cell bodies and dendrites. Retarded movement of extracellular fluid and clearance of CSF might unfavorably alter the extracellular environment. The type of brain damage depends on the developmental state of the CNS.
Tissue location / cell type | Type of damage | Functional consequence |
---|---|---|
Ependyma | Patchy loss (especially over white matter) | Theoretical loss of barrier function, but likely not actually significant |
Germinal matrix in fetuses | Reduced cell proliferation (not proven in human brain) | Interference with production of neuron and glial precursors; possible contribution to epilepsy if inhibitory interneurons are faulty |
Axons (periventricular) | Mechanical and ischemic injury | Disconnection syndromes, cerebral palsy |
Myelin and oligodendrocytes | Delayed myelination secondary to chronic ischemia | Neuron dysfunction (potentially reversible by shunting) |
Hippocampal neurons | Deafferentation atrophy (secondary to axon damage) | Memory deficits |
1 | Examine the inner surface of the skull for evidence of chronic pressure-related changes, prior trauma, or structural abnormality of the posterior fossa including foramen magnum. |
2 | Examine the outer surface of the brain for evidence of leptomeningeal fibrosis or arachnoid cysts (which could be secondary to scarring). |
3 | Examine the brain in detail for evidence of malformation. In fetuses, carefully inspect the corpus callosum through the interhemispheric fissure (if there is autolysis, the callosum might not be obvious after coronal slicing). |
4 | After coronal slicing, evaluate ventricles for symmetry and possible sites of obstruction. |
5 | If acute ventriculomegaly is suspected, evaluate samples of corpus callosum and angles of frontal horns histologically to look for axonal damage. Petechial hemorrhage at the angles of the ventricles is a clue indicating this. |
6 | In cases with chronic severe hydrocephalus, examine the corpus callosum and fornix carefully for evidence of atrophy. Horizontal tissue blocks of the fornix immediately above the mammillary bodies are recommended. Anterograde and retrograde axonal changes may also be obvious in the alveus/fimbria over the hippocampus. Atrophy of the pyramidal tracts in the brainstem or spinal cord may reflect diffuse but subtle damage in the internal capsules or corona radiata. Myelin stains are very useful in postnatal cases. |
7 | Evaluate samples of ventricle wall including ganglionic eminence / caudate nucleus to look for evidence of prior hemorrhage. Widespread ependymal loss and/or periventricular calcifications may be suggestive of previous infection. |
8 | Thin slice and block the entire midbrain and pons to evaluate patency of the cerebral aqueduct and fourth ventricle. |
9 | If the child has received a ventricular shunt device, it is important to rule out an obstruction or infection. Try not to disturb the ventricular or peritoneal catheters until their tips can be examined in situ for evidence of obstruction. Perform microscopic examination on tissue surrounding or growing into the catheter tips. If there is nothing obvious, flush the catheter. |
In the developing CNS, most cell proliferation occurs in the VZ and SVZ, located in the walls of the cerebral ventricles (see Chapter 20). CSF circulation and composition might be important for normal function of the germinal matrix (107). In ferrets with kaolin-induced hydrocephalus, SVZ proliferation is inhibited by hydrocephalus (108). Confirmatory human fetal data are limited to preliminary studies (Del Bigio et al., unpublished). Many studies have shown variable loss of ependyma over white matter structures in hydrocephalic brains. This is assumed to be a destructive process caused by stretching of the ventricle wall and the inability of ependymal cells to regenerate (99). Although the ependyma serves a barrier function, the practical consequences in humans are not proven (12, 109). There is sparse evidence that choroid plexus cells might be discharged into the CSF of hydrocephalic infants (110).
Periventricular white matter is the main site of brain damage in hydrocephalus (99, 102, 103). Children who died of progressive hydrocephalus had atrophy of the corpus callosum and periventricular white matter (111). This is associated with motor and cognitive deficits (112). A magnetic resonance imaging study of hydrocephalic children showed changes suggestive of delayed myelination (113, 114). Although the cerebral mantle may be severely thinned, gray matter and neuron damage appear to be largely secondary to the axonal injury unless the cerebral white matter is entirely destroyed or the cerebrum ruptures (24, 115, 116).
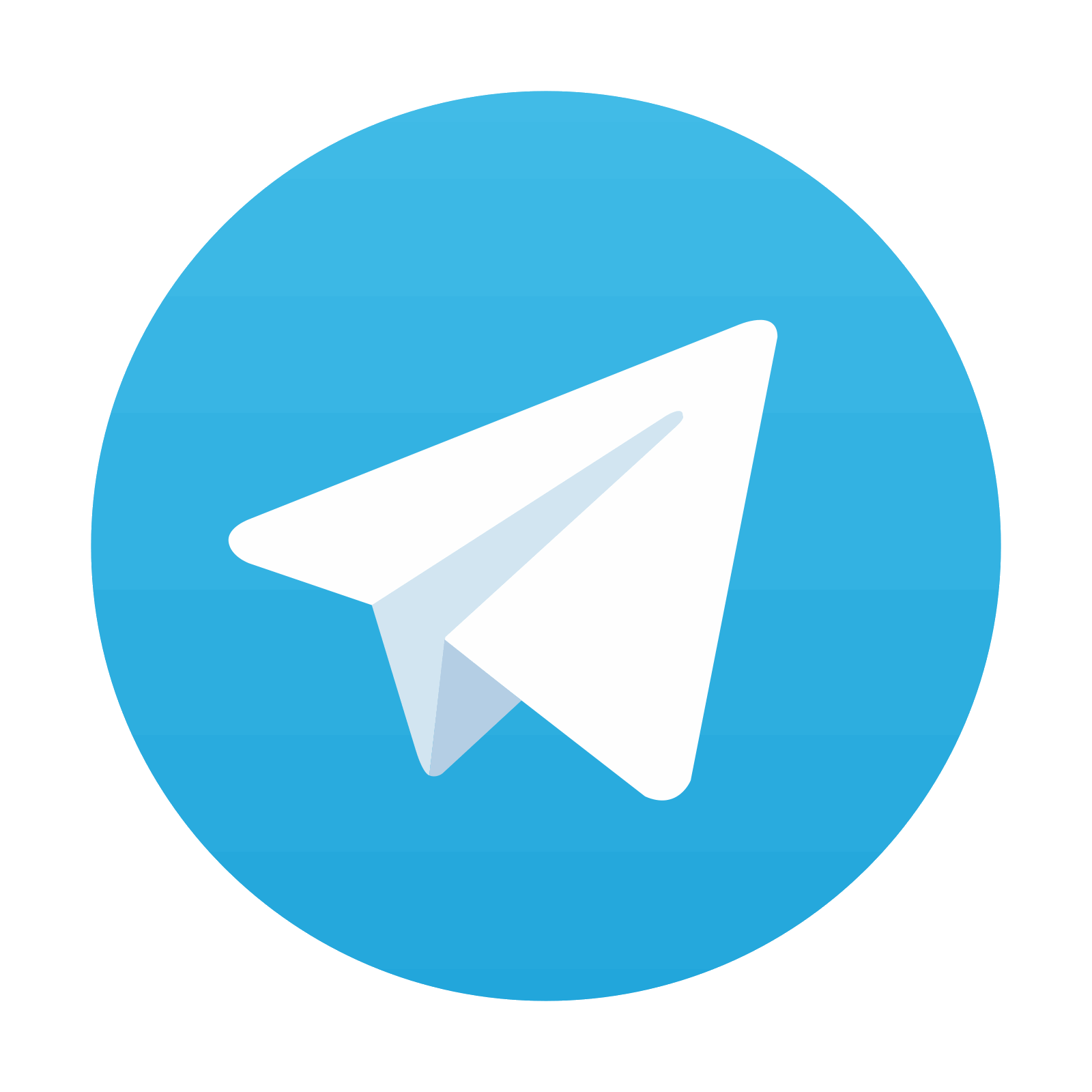
Stay updated, free articles. Join our Telegram channel
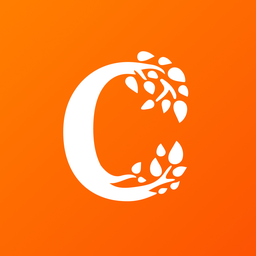
Full access? Get Clinical Tree
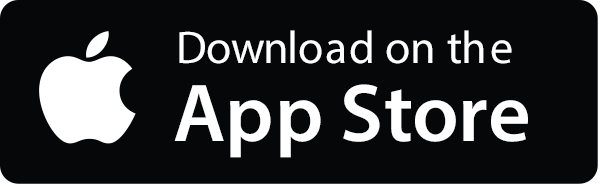
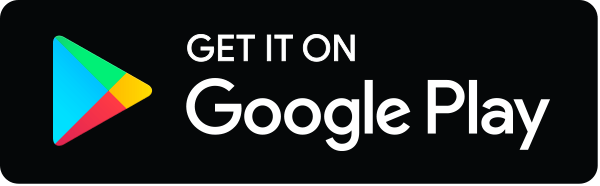