Neuron Death in the Developing Brain
The idea that loss of neurons could be associated with disease states and that certain microscopic appearances could be indicative of these arose in the mid to late 1800s. If neurons die, become dysfunctional, or are disconnected following a challenge, functionality of the affected neural circuit is compromised. Depending on the magnitude and localization, this may have relatively mild consequences (such as a learning deficit) or major consequences (such as developmental delay, epilepsy, cerebral palsy, or death). We are interested in selective or regional neuron death because they can help to explain the neurologic dysfunction experienced by an individual or give insight into the pathologic process that preceded death.
In resected tissue or in the brain of a deceased person, obviously all the cells are dead. The diagnostic pathologist, however, must recognize features of neurons that had been damaged prior to cessation of all physiologic processes. This relies on morphological or biochemical contrasts with “normal” appearances in control tissue or in neighboring cells. Note that mildly injured cells undergo morphologic changes such as swelling, which is not necessarily associated with a fatal outcome. These changes can be difficult to discriminate from post mortem artifacts.
Normal and Pathological Cell Death
The concept of “programmed cell death” within normal tissues appeared in the developmental biology literature in the 1960s. The term was meant as a descriptor of cells that were genetically predetermined to die once they had served their purpose during development (i.e., planned obsolescence; 1). In 1972, based upon morphologic features, Kerr et al. introduced the term “apoptosis” to describe a form of cell death involved in cell turnover in normal tissues (i.e., in programmed cell death), in neoplasms, and following exposure to certain “noxious agents” (2). Shortly after, Schweichel and Merker showed that not all cells undergoing programmed death during development had the same morphologic features. They identified apoptotic cells (type 1), autophagic cells (type 2), cells with swollen organelles and cytoplasmic spaces (type 3A), and cells with mitochondrial swelling, dilation of endoplasmic reticulum, and karyolysis (type 3B; 3, 4). To further blur the distinguishing features of programmed cell death, it is now apparent that some interneurons survive or die (by apoptosis) according to the local physiologic needs, which are dictated by activity during brain development (5).
As the biochemical mechanisms of apoptosis were elucidated, some authors erroneously began to equate the terms apoptosis and programmed cell death in the context of neurological aging and disease states (6–10). Some ignored the developmental aspect almost entirely and re-defined programmed cell death as “a series of stereotypical biochemical and morphological alterations leading to cell demise” (11). A more appropriate term for these orderly biochemical cascades is “regulated cell death” (12, 13).
The term “necrosis” was originally defined as the localized death of living tissue. Virchow introduced the idea of a “nekrobiotischen Prozesse” (necrobiotic process) that left a “Erweichung im Gefolge” (softening effect as the end result). He recognized that a “vorübergehenden Ernährungsstörun” (temporary nutritional disturbance) can damage the brain (14). The idea of “selective neuronal necrosis” (i.e., death of individual cells) began to appear in the medical literature in the late 1930s in the context of encephalitis (15) and by the 1950s was used in reference to kernicterus and hypoxic birth injury (16–19).
Pathology textbooks began to describe cell death as a duality of necrosis (“accidental death”) and apoptosis (“programmed death”). However, it is now apparent that there are multiple, partially overlapping biochemical mechanisms through which cells, including neurons, can die (20, 21). The intensity and exact type of insult might dictate the cell death pathway activated. Several forms of cell death may be triggered through the same cell surface receptors and mediated by increases in mitochondrial and/or lysosomal permeability (21). Multiple death pathways may be simultaneously activated in individual neurons (22). Note that the definitions used by authors are not entirely consistent with authorities’ suggestions. International consensus groups (e.g., the Nomenclature Committee on Cell Death, NCCD) offer some direction in this regard (12, 23–26).
It is important to note that the molecular mediators of cell death might differ between human neurons and experimental animal neurons in some situations (27). For example, DNA damage appears more quickly in the non-human primate brain than in the rat brain following focal ischemia (28).
Modes of Neuron/Cell Death
Apoptosis. Apoptosis is the archetypal mechanism of programmed cell death (29), but apoptosis is not a synonym of programmed cell death (24). It is active during normal development and in pathological processes. Apoptosis may be activated through two main pathways. The extrinsic death receptor pathway is triggered by the ligation of tumor necrosis factor (TNF) – family death receptors on the cell membrane. The intrinsic mitochondrial pathway is regulated by Bcl-2 family proteins (21). Energy failure (typically less severe than that which causes necrosis) leads to dysregulation of Ca++, excessive opening of mitochondrial permeability transition pores (30), release of cytochrome C, and activation of the caspase cascade. Caspase-3 is the major ‘executioner’ caspase. Activated caspase-3 can be detected by immunostaining in normal and damaged nervous tissue with relative specificity for apoptosis (31–36). Caspase-3 activates caspase-activated DNase (properly known as DNA fragmentation factor, DFF), a heterodimeric enzyme comprised of alpha and beta subunits. It then cleaves nuclear DNA at internucleosomal sites (i.e., between histone cores) into regular small fragments (multiples of 180-base-pairs; 37–40). Caspase-6 cleaves the nuclear envelope protein, lamin A; this step is necessary for chromosomal DNA to undergo complete condensation during apoptosis (41). Apoptotic cells shrink and are phagocytosed rapidly without causing significant inflammatory stimulus because they do not release their cellular constituents and the phagocytic cells do not produce pro-inflammatory cytokines (29).
The mRNA for CASP3 is expressed at moderate levels in the brain until approximately four months postnatal, after which it drops to low levels. The mRNA of DFFA and DFFB is expressed at low levels in the brain throughout life (Developmental Transcriptome database 42). Caspases and DFF may also be involved in non-apoptotic regulation of cell fate determination (40, 43).
Characteristic DNA fragments are detectable on electrophoresis gels as a banded pattern (DNA ‘laddering’) and can be demonstrated by PCR (44). Fragmented DNA can be detected by a variety of in situ methods. Terminal deoxynucleotidyl transferase dUTP nick end labeling (TUNEL), which detects 3′-hydroxyl terminals in double-strand DNA breaks, is often promoted as a means for demonstration of apoptotic cells. However, it is not actually specific as these breaks also occur in necrotic cells, including neurons (45–48). The in situ oligonucleotide ligation (ISOL) method, which detects 5′-phosphate terminals at double-strand blunt-ended DNA breaks, is more specific for apoptosis (49, 50).
Linker cell-type death. In many developing organisms, programmed cell death does not necessarily occur through apoptosis (51). This mechanism refers to linker cells in the gonads of C. elegans worm larvae. In contrast to apoptosis, which is characterized by cell condensation, linker cell-type death (LCD) in the embryonic mouse nervous system features swollen organelles, dispersed chromatin, and an irregular nuclear envelope (52, 53). LCD does not require caspases; it functions through the ubiquitin proteasome system, the key biochemical steps including induction of ubiquitin conjugating enzyme E2 D2 (UBE2D2) by heat shock transcription factor 1 (HSF1; 54). LCD may serve as a backup when caspases are unavailable. This mechanism has not been studied in human tissue, but the mRNAs for both major factors are present at moderate levels in the human brain throughout life (Developmental Transcriptome database; 42).
Oncosis. This form of accidental necrotic cell death is “virtually instantaneous and uncontrollable … corresponding to the physical disassembly of the plasma membrane caused by extreme physical, chemical, or mechanical cues” (12). In simplest terms, this is the passive death of cells that results when homeostatic mechanisms cease, for example as a consequence of anoxia (energy failure) or high dose radiation (ionizing damage to protein, lipid, and nucleic acids). Energy failure leads to loss of ion gradients and passive movement of water (55). Oncosis is morphologically characterized by increased cell volume, swelling of organelles, plasma membrane rupture, and subsequent loss of intracellular contents (24).
Necrosis. The terminology used to describe tissue necrosis patterns (coagulative, liquefactive, caseous, fibrinoid, etc.) has no relationship to the cellular processes. Acute deprivation of oxygen or glucose leads to decreased ATP formation, which is necessary for the maintenance of Na+/K+ exchange pump activity at the cell membrane. Loss of the Na+ gradient allows passive movement of water, which causes organelle and cell swelling, and allows influx of Ca++ into the cytosol from the outside and from endoplasmic reticulum. The Ca++ in turn indiscriminately activates calpain 1 (which degrades proteins) and phospholipases (which damage cell membranes; 21, 56). Calpain-mediated cleavage of the membrane protein, alpha II-spectrin, can be detected by immunostaining (57–59). The mRNA of calpain 1 (CAPN1) is highly expressed in the human brain from the embryonic period throughout life (Developmental Transcriptome database; 42). Damage to lysosome membranes releases other proteases (cathepsins B and D) that contribute to cellular and tissue degradation.
Damaged lysosomes also discharge enzymes that degrade nucleic acids. Deoxyribonuclease 2 (DNase 2) hydrolyses phosphodiester linkages of the DNA backbone thereby releasing random size, small DNA fragments (60, 61). Blunt-ended DNase2 cleavage breaks can be detected using a topoisomerase-based labeling method (62). DNASE2 mRNA is expressed in the human brain at moderate levels beginning at around 24 weeks gestation (Developmental Transcriptome database; 42). Ribonuclease 1 (also known as alkaline RNase or RNase A) and ribonuclease K are both present in human neurons (www.proteinatlas.org; 63, 64). Within six hours of experimental cerebral ischemia in gerbils, an “acid ribonuclease” – likely RNase K (65), but not alkaline ribonuclease – is released (66). This accounts for ribosome degradation seen in damaged neurons. At the mRNA level, ribonuclease 1 (RNASE1) and ribonuclease K (RNASEK) are the main RNases expressed in the human brain, the former beginning at about 25 weeks gestation and the latter throughout fetal and postnatal life (Developmental Transcriptome database; 42).
Necroptosis. Necroptosis is a form of regulated necrosis. Extrinsic inflammatory activation of death receptors (e.g., FAS, TNFR1, which are also involved in apoptosis) or direct activation routes (e.g., by some viruses) lead to phosphorylation of receptor-interacting protein kinase 1 (RIPK1) and subsequent oligomerization of RIPK3 (67). Phosphorylation and oligomerization of mixed lineage kinase-like protein (MLKL) then causes cell membrane damage (68). Experimental ischemic or traumatized neurons can die by necroptosis, the morphology of which is not distinguishable from other forms of necrosis (69–71). However, the mRNAs for RIPK1, RIPK3, and MLKL are only expressed at extremely low levels in the normal human brain at any age (Developmental Transcriptome database; 42). In situ demonstration of RIPK1 and RIPK3 activation has been considered to be problematic (72, 73). Nevertheless, there are reports of RIPK1 and MLKL immunoreactivity in the inflammatory lesions of multiple sclerosis (74), and in microglia of injured human spinal cord (75).
Parthanatos. Parthanatos is a form of necrosis that depends on the activation of poly(ADP-ribose) polymerases (PARP-1, which may be triggered by oxidative stress DNA damage), followed by the release of apoptosis-inducing factor (AIF) from mitochondria. AIF forms complexes with macrophage migration inhibitory factor (MIF), and is translocated to the nucleus where the latter degrades chromatin (21, 76). The mRNAs of PARP1 and AIFM1 are expressed at moderate levels and MIF at high levels in the human brain throughout life (Developmental Transcriptome database; 42). Increased PARP1 immunoreactivity has been demonstrated in ischemic human brain tissue (77).
Ferroptosis. Ferroptosis is a type of cell death that is characterized by the iron-dependent accumulation of lethal quantities of lipid hydroperoxides. It is implicated in the neuron damage associated with experimental ischemic and hemorrhagic brain lesions (78). Shrunken mitochondria are one of the morphologic features (79). Indirect indicators such as increased 15-lipoxygenase and decreased selenoperoxidase (GPX4) have been reported in experimental brain trauma (80). The mRNA for GPX4 is expressed at high levels in the human brain throughout life while ALOX15 mRNA is present at negligible levels (Developmental Transcriptome database; 42).
Pyroptosis. Pyroptosis is a type of necrosis mediated by caspase-1 following hypoxia/ischemia or inflammatory stimuli. Activated caspase-1 cleaves gasdermin D, which then polymerizes to form pores in cell membranes (21). Although pyroptosis has been shown in several experimental brain diseases, the mRNAs for CASP1 and GSDMD are present at negligible levels in the human brain at any age (Developmental Transcriptome database; 42).
Autophagy/autosis. Autophagy (“self-eating”) is a process whereby cell components are engulfed by lysosomes for digestion and recycling. It is generally considered to be a survival mechanism during extreme stress (21). Autophagosomes accumulate in experimental neonatal hypoxic-ischemic encephalopathy (81) and can reliably be detected using immunohistochemical markers for microtubule-associated light chain 3 protein (MAP1LC3A, also known as LC3) and beclin 1, among others (82). The mRNAs for MAP1LC3A and BECN1 are both present at moderate to high levels in the human brain throughout life (Developmental Transcriptome database; 42). The morphology of autophagic neurons differs from necrotic and apoptotic neurons. Although molecular overlaps present some uncertainty if autophagy is the cause of cell death or an ineffective attempt to survive, most authorities believe that excess autophagy can cause cell death (21, 83, 84). Some autophagic neurons might undergo apoptosis (85).
Other forms of cell death. In addition to those discussed above, other cell death mechanisms have been described, but not necessarily within brain cells in vivo. These include paraptosis, phagoptosis (entosis), mitochondrial permeability transition driven necrosis, immunogenic cell death, lysosome-dependent cell death (autolysis), oxieptosis, and alkaliptosis (12, 21, 86).
Instigators of Cell Death in the Central Nervous System
Beyond programmed cell death in the developing CNS, many factors can cause neurons (and other cell types) to die (21). Because of their high metabolic demands and their high density of ionotropic glutamate receptors (which function as ligand-gated cation channels), neurons are highly susceptible to anoxic depolarization and are relatively more sensitive to stressors than most other cell types in the CNS (87, 88). Immature (premyelinating) oligodendrocytes may be equally susceptible in the 23–32 week gestation period as a consequence of glutamate receptors on those cells (89, 90). In the context of perinatal neuropathology, hypoxia-ischemia, inflammation, reactive oxygen and reactive nitrogen species, and excitotoxicity are the most important factors to consider (60, 91). John Olney proposed the principle of excitotoxicity to explain how glutamate and its analogues could have both excitatory and toxic effects on neurons (92, 93). Protracted glutamate stimulation of neurons leads to Ca++ influx, which in turn can cause mitochondrial dysfunction and activate a range of proteolytic enzymes including calpains that cause cell death (94, 95). In culture, neurons exposed to low doses of the excitotoxin N-methyl-D-aspartate (NMDA) undergo apoptosis, while high doses of NMDA cause necrosis (96).
Other causes of neuron death include loss of neuron-to-neuron connections (transneuronal degeneration), axotomy, aberrant cell cycle reentry, and toxic protein aggregates with unfolded protein response (21).
Histological Features of Damaged and Dead Neurons in the Developing Brain
On hematoxylin and eosin-stained sections, normal neurons typically have a large round nucleus with prominent nucleolus and a fine stippled chromatin pattern (Figure 29.1 A). The cytoplasm is faintly basophilic due to ribosomes; the largest neurons have clumps of basophilic rough endoplasmic reticulum (“Nissl substance”) at the periphery. The morphology of apical and basal dendrites varies considerably depending on the age and location. In situations where the time to fixation has been delayed, post-mortem autolytic changes may manifest as fragmentation of the cytoplasm (due to the release of lysosomal enzymes; Figure 29.1 B; see Chapter 27). In cases with white matter damage, neurons with axonal injury may show central chromatolysis wherein the nucleus is pushed to the cell periphery (Figure 29.1 C). Following a hypoxic-ischemic insult, the earliest neuronal change (<1 hour) is swelling of organelles (which may appear as cytoplasmic vacuoles of various shapes and sizes; Figure 29.1 D-F; 97). Associated astroglial swelling imparts the appearance of empty spaces around neurons and capillaries. These changes, which are due to passive water shifts, are theoretically reversible. Within 1–6 hours, definitive features of irreversible damage appear in neurons. In experimental animals, necrotic neurons are first evident at 1 hour and abundant by 6–12 hours after cerebral ischemia (98, 99). In adult humans following cardiac arrest, early neuronal eosinophilia and nuclear pyknosis are evident in the hippocampus, arterial boundary zone cortex, and putamen at 1–2 hours (97). The appearance depends to some extent on the gravity of the injury. Complete loss of homeostasis caused by a severe insult is associated with swelling and fragmentation (oncosis); this may be indistinguishable from post-mortem autolysis. In the various regulated forms of necrosis, activation of calpains and cathepsins (which digest proteins) and ribonucleases (which digest ribosomes) renders the cytoplasm of necrotic neurons hypereosinophilic. Fluoro-Jade C, a fluorescent marker, stains all degenerating neurons, regardless of the initial insult type or mechanism of cell death. Although it shows essentially the same neurons that are hypereosinophilic, it has a much better signal to noise ratio (100). The release of deoxyribonuclease causes digestion of the nucleus with progressive loss of chromatin staining (Figure 29.1 G–I; 23). Neurons that undergo apoptosis show contraction of the cell body and digestion of the nucleus into a single or multiple, usually round and intensely hematoxyphilic, structures (karyorrhexis; Figure 29.1 J; 23, 101).
Figure 29.1 Photomicrographs of compromised and dead neurons in the immature central nervous system. A. Normal cortical neurons (surrounded by slightly swollen glial cells) in an infant who died suddenly. B. Cortical layer 5 in an infant who died suddenly and whose autopsy was delayed 3 days. Note the normal neuron surrounded by neurons with autolytic vacuolization of the cytoplasm (arrows). C. Neuron with mild central chromatolysis (arrow) in the putamen of a full-term infant with hypoxic encephalopathy who died 2 days after birth despite cooling of the head. D. Spinal motor neuron with (pseudo crystalline) expansion of the Golgi or endoplasmic reticulum (arrow) in an infant who died with swollen brain. E. Spinal motor neuron with engorgement of the endoplasmic reticulum (arrow) in the same case. F. Neuron with swollen endoplasmic reticulum (arrow) in a brain with severe hypoxic-ischemic changes. In theory, the changes shown in C–F would have been reversible if the child had lived. G. Dead cortical neuron in a full-term stillborn infant showing early nuclear pyknosis (shrinkage) and eosinophilic change in the cytoplasm. H. Dead pontine neurons with advanced cytoplasmic hypereosinophilia and nuclear karyolysis (arrows) in a full-term stillborn infant. I. Dead neuron (ghost neuron) with complete loss of nuclear staining (arrow) in the cortex 6 days after a severe hypoxic insult. J. Dead pontine neurons with karyorrhectic nuclear change (arrow) in a 38-week gestation stillborn infant. K. Early mineral encrustation (arrows) of a thalamic neuron in a term infant with kernicterus and severe hypoxic-ischemic change. L. Mineralized fossils of thalamic neurons in a 9-week infant who suffered severe perinatal hypoxic encephalopathy. All photomicrographs taken at 600x or 1000x original magnification. All hematoxylin and eosin stain.
Considering the variety of biochemical routes through which neurons can die, it would not be surprising to point out that not all eosinophilic neurons are the same. In experimental cerebral infarction, eosinophilic neurons with cytoplasmic shrinkage are present in the ischemic core, while those with nuclear disintegration and slightly atrophic cell bodies are present in the ischemic periphery (102). Dead neurons will gradually disappear with debris phagocytoses by neighboring microglia. In principle, necrotic cells that release their debris into the surrounding tissue can incite an inflammatory response. This may be largely microglial or can include neutrophils. The extent to which this happens will depend on the integrity of blood flow (necessary to deliver leukocytes) and the health of the tissue overall (i.e., selective neuronal death versus regional infarction). Experimental animal studies suggest that dead neurons disappear by 5–7 days after hypoxia-ischemia (103–105). With respect to interpretation of the insult timing, a complicating factor is the fact that neuron necrosis may in some situations be delayed (98, 106). In experimental animals, the onset of visible signs of hippocampal neuron necrosis can be delayed by 7 days or more, particularly with brief periods of cerebral ischemia (107). Likely the same delay is possible in humans.
Dying neurons in the immature nervous system may undergo mineralization in a scattered, apparently random pattern (Figure 29.1 K–L). These can occur anywhere but are easiest to find in the neocortex, thalamus, and brainstem following hypoxic-ischemic damage (108–112). Calcium and phosphorus salts are the main constituents of the basophilic particles, but they may also contain iron. Consequently, various descriptive terms include mineralized, calcified, ferruginated, and rarely ferrocalcinosis (which usually refers to vascular changes; 113, 114). There is some experimental evidence that excitotoxicity predisposes to calcification (115). Complete mineralization of neurons is seldom seen earlier than 6 days after an insult (116).
Prototypical morphologic features of dead neurons are shown diagrammatically in Figure 29.2 and features of dead neurons that can be practically used in diagnostic neuropathology are summarized in Table 29.1.
Figure 29.2 Diagrammatic representation of the prototypical changes in dead neurons that have died by unregulated oncosis (followed by fragmentation), regulated necrosis (with hypereosinophilic cytoplasm and eventual possible mineralization), apoptosis (with karyorrhectic nuclear fragmentation), and autophagy (with lysosomal vacuoles). It may be difficult in some situations to distinguish neurons that died prior to somatic death from postmortem artifactual changes including autolysis (which is primarily due to the release of lysosomal enzymes) and putrefaction (which is caused by bacterial enzyme digestion).
Mode of death | Morphology in brain tissue (12, 86) | In situ detection methods (21) |
---|---|---|
Apoptosis | Single or small clusters of cells; shrunken hypereosinophilic cell body; chromatin condensation or beading (karyorrhexis) | Activated caspase 3 / 8; DNA fragmentation (ISOL is more specific than TUNEL) |
Oncosis (unregulated) | Single or large areas of contiguous cells; swollen pale cell body ± vacuoles; fragmentation of chromatin (karyolysis); cellular debris; inflammation (24) | None |
Necrosis (regulated) | Loss of Nissl substance; pyknotic hypereosinophilic cell body; pyknotic nucleus; fragmentation of chromatin (karyolysis); ghost cells | Calpain cleavage products (e.g., spectrin); DNA fragmentation into small random fragments (TUNEL); Fluoro-Jade C (100; none are specific) |
Necroptosis | Similar to oncosis (?; 21) | RIP1 and/or RIP3 activation; MLKL phosphorylation |
Ferroptosis / oxytosis | Similar to necrosis (?; 21) | Lipid peroxidation and responses (e.g., increased 15-lipoxygenase) |
Parthanatos | Similar to necrosis (?; 21) | PAR accumulation + AIF translocation to nucleus |
Autophagy | Mild chromatin condensation; cytoplasmic accumulation of autophagosomes and small vacuoles; swelling of perinuclear space (82) | Lysosomal activation (MAP1LC3A / LC3; beclin 1) |
Neuronal Maturation Affects the Mode of Cell Death
The stage of neuronal maturation, as well as the type of neuron, the severity of the insult, and likely other factors determine whether a neuron dies by apoptosis or by one of the regulated or unregulated forms of necrosis. Experimentally it was shown that “brain maturity influences the morphologic phenotype of neurodegeneration and that excitotoxic neuronal death in the immature brain is not a uniform event but, rather, a continuum of apoptotic, necrotic, and overlapping morphologies” (117). Because apoptosis is a normal feature of CNS development, several components of the intrinsic apoptotic pathway (many in the Bcl2 family) remain upregulated in the immature brain and are downregulated with maturation (118–120). Conversely, the “apoptosis repressor with caspase recruitment domain” (ARC or Nol3), a potent inhibitor of apoptosis in neurons and other tissues (121), is upregulated at the mRNA level in the human brain beginning at about 35 weeks gestation (Developmental Transcriptome database; 42). In general, less mature neurons (usually before full-term gestation in the human brain) and milder insults will be associated with apoptosis while mature neurons are relatively resistant to intrinsic apoptosis (122). Because multiple physiologic and anatomical variables are involved, a mix of karyorrhectic and hypereosinophilic forms is not uncommon within single foci.
Overlap of Neuron Death and Axon Damage Processes
Axon damage is considered in detail in Chapter 34. Here we note only that some of the enzymatic processes responsible for killing neurons are also active in focal axon injury (123). Axons are not simply conduits for vesicular transport of synaptic molecules and propagation of electrical potentials. Because of their considerable extension from the neuron cell body, many metabolic processes take place locally within axons including mitochondrial production of ATP along with the synthesis of structural and transmembrane proteins. Axon homeostasis is dependent on local nutrient and oxygen delivery as well as neighboring astroglial and oligodendroglial cells (124). Increased permeability to Ca++ at sites of physical damage or hypoxia-ischemia (125) can initiate the destruction of the axoskeleton by calcium-dependent calpains, caspases, cysteine proteases, and phosphatases (123, 126). This is essentially the same as necrosis in the neuron cell body. The necroptosis pathway may be activated in axons and oligodendrocytes in some experimental situations (127–129). Retrograde axonal degeneration occurs through other molecular processes (130).
In situ detection of damaged axons typically relies on immunohistochemical demonstration of accumulating proteins that are normally transported to the axon terminal, in particular beta-amyloid precursor protein (131). Damaged axons in the white matter can be detected within 30 minutes of metabolic or physical compromise (132, 133). Immunostains can also be used to demonstrate calpain cleavage products including fragments of spectrin (134), which is a critical and abundant component of the axon membrane skeleton (135), and fodrin, a spectrin-like protein (136). Caspase-mediated cleavage of axonal actin and tubulin occurs after experimental and clinical cerebral ischemia (137). In the normal CNS, pruning of neurites during synaptic remodeling (plasticity) shares some of the caspase-regulated destruction programs with neuronal cell death (138).
References
Astroglia
Astrocytes are the cells most responsible for dynamic homeostasis of the central nervous system (CNS; 1, 2). During development, they arise from maturing radial glia as well as from symmetric division of existing astrocytes (3, 4). Their cell processes are in intimate contact with synapses, myelin internodes, and capillaries. They are involved in the flow of extracellular fluids through the glymphatic system (5). Gap junctions form an extensive interconnected network of astrocytes with their brethren and with oligodendrocytes. Signaling by calcium ion and other factors helps coordinate their activities (6). They are responsible for recycling many neurotransmitters, regulating blood flow in the microdomains, and maintaining the blood-brain barrier. With this plethora of duties, astrocytes must be able to react rapidly to a range of stresses and insults.
Astrocytes are recognizable by their ovoid, slightly irregular nuclei with fine stippled chromatin pattern. The cytoskeleton has glial fibrillary acidic protein (GFAP) and vimentin intermediate filaments that can be detected easily by immunostaining. Astrocytes exhibit considerable heterogeneity in shape and properties depending on the locale (7). In white matter, GFAP immunoreactive cells are widespread. In normal gray matter, GFAP is more restricted to perivascular and subpial astrocytes (Figure 30.1), while gray matter “protoplasmic” astrocytes have negligible GFAP. Astrocytes express high levels of glutamine synthetase, glutamate transporter 1 (GLT1), and aquaporin-4 in the cytoplasm or membranes. SOX9 has been used as a nuclear marker, although it is also expressed by ependymal cells and by immature germinal cells (8).
Figure 30.1 Astrocytes and astroglial reactions. A) Immunostain for GFAP (brown; with hematoxylin counterstain) showing normal stellate astrocytes with abundant processes and small cell bodies (arrow) in the white matter of a 7-month infant. B) Immunostain for vimentin (brown) showing normal perivascular astrocytes (arrow) in the gray matter of a 4-month infant. C) Immunostain for vimentin (brown) showing normal astrocytes in the white matter of a 4-month infant. To appreciate the morphology of astrocytes with anti-vimentin is often easier than with anti-GFAP because the former is less abundant. D) Swollen astrocytes with clear cytoplasm (arrow) within 1 hour of hypoxic-ischemic brain damage. Periodic acid-Schiff stain. E) Swollen astrocytes with expanded nucleus (arrow) within hours of hypoxic-ischemic brain damage. Hematoxylin and eosin stain. F) Swollen astrocytes with eosinophilic cytoplasm (arrows) as a consequence of plasma protein pinocytosis in the vicinity of a contusion that occurred 2 days prior to death. G) Early reactive astrocyte with enlarged, convoluted nucleus (arrow) near a contusion that occurred 3 days before death. H) Mitotic cell (presumed astrocyte, arrow) near a contusion that occurred 5 days before death. GFAP Immunostain. I) Hypertrophic astrocytes (arrow) in the damaged caudate nucleus of an infant with glutaric academia type 1. GFAP Immunostain. J) Residual chronic astroglial cytoskeletal change (astroglial “scar”) shown as blue fibrils with modified phosphotungstic acid hematoxylin stain (mPTAH; 18) in the atrophic cerebellum of a child. Normal tissue would show only scant blue staining at the subpial surface.
The most rapid response of astrocytes to neuronal stress in the context of hypoxia-ischemia is swelling. Active uptake of potassium ion and glutamate is accompanied by a passive influx of water, which causes expansion of the cytoplasm and some organelles within 15 minutes (9). These cells have diluted cytoplasm and/or nuclei (Figure 30.1). Through pinocytosis, astrocytes are capable of ingesting plasma proteins at sites of vascular injury. In this circumstance, the cells become swollen with a strongly eosinophilic cytoplasm (Figure 30.1; 10). Reactive astrogliosis is a more complex process that is evolutionarily directed at modulating acute stress, limiting tissue damage, and restoring homeostasis (11, 12). Prior to the upregulation of hundreds of proteins, the nuclei of astrocytes become enlarged and irregular. Within 3–5 days after an acute insult, some of the astrocytes may undergo mitosis (Figure 30.1). Others become engorged with proteins and an expanded cytoskeleton. These cells, with enlarged cell bodies and stubby processes, are typically referred to as gemistocytic or reactive astrocytes. They produce a host of trophic substances, including vascular endothelial growth factor (VEGF), which promotes endothelial proliferation and makes blood vessels more permeable (13). Some evidence indicates that the reaction of astrocytes differs depending on whether the CNS insult is primarily inflammatory or ischemic, in part because of the stimulation of astrocytes by cytokines (14, 15).
Astroglial proliferation at the sites of injury helps restore homeostasis. Months after the insult, the reactive cells atrophy but they do not completely return to normal. Their altered cytoskeletons can be demonstrated with the modified phosphotungstic acid-hematoxylin stain (PTAH; Figure 30.1; 18). In some respects, this represents the astroglial “scar” (15, 19).
Oligodendroglia
Oligodendrocytes are the myelin-producing cells of the central nervous system. In addition, they provide trophic support to axons (20). Progenitors arise largely from the subventricular zone (SVZ) during the fetal period (21). Oligodendrocyte precursor cells (OPC), which are capable of proliferating and myelinating, persist in mature human white matter (22) and are estimated to constitute 5% of the glial cell population (23).They can be identified by the expression of proteoglycan NG2 or platelet-derived growth factor receptor A (PDGFRA; 24). Mature oligodendrocytes can be identified tentatively by their characteristic round nuclei. More definitive identification is achieved by immunolabeling of Olig2 or SOX10 transcription factors in the nuclei (25), or of myelin-related proteins. The latter are satisfactory in the premyelination phase, but in well-myelinated regions may not be helpful for identifying cell bodies.
Oligodendrocytes have a limited repertoire of reactive states, which are not really noticeable in routine neuropathologic examinations. Post injury oligodendrogliogenesis occurs in experimental situations (26). In the traumatized adult human brain, oligodendrocyte death seems to be at least partially compensated for by an increase in OPCs (23, 27). It remains to be determined if OPC proliferation shown in experimental studies translates to a significant OPC reaction in human perinatal hypoxic-ischemic white matter injury (21).
Ependyma
The ependyma is a simple ciliated epithelium that lines the ventricular system including the central canal of the spinal cord (28, 29). The cells tend to be flat to cuboidal in the lateral ventricles and more columnar along the third and fourth ventricle wall and central canal. Terminal differentiation of the cells occurs as proliferative activity in the ventricular zone gradually decreases (30). During the differentiation phase, the ependymal lining may be pseudostratified (Figure 30.2). Differentiation occurs earliest in the central canal, fourth ventricle, and cerebral aqueduct, typically by mid-gestation. The last region to develop mature ependymal cells is the ganglionic eminence over the caudate nucleus, usually by about 30 weeks gestation. For many years, ependymal cells were purported to be capable of dedifferentiation into neural stem cells; however, recent work suggests that this is unlikely (31). Sparsely distributed, residual SVZ cells likely hold that role (32).
Figure 30.2 Ependyma and ependymal reactions. A) Ciliated ependymal cells over the dorsal ganglionic eminence of a normal 23-week gestation fetus. Note the pseudostratified organization (arrow). B) Cuboidal ependymal cells over the normal corpus callosum. C) Focal interruption of ependymal cells with an expansion of the subependymal astroglial layer (arrow). D) Buried ependymal rosettes over the caudate nucleus head of an infant who had suffered a small germinal matric hemorrhage. E) Normal GFAP immunoreactivity (brown) in the ependymal layer of the fourth ventricle in a 20-week gestation fetus. The long processes are likely radial glia (arrow). F) Ubiquitin immunoreactivity (brown) in buried and luminal ependymal cells around the cerebral aqueduct of a 4-month infant. G) Central canal of the cervical spinal cord of a normal 23-week gestation fetus. It is filled with amorphous material and a few cells (arrow). H) Subcommissural organ in the posterior third ventricle of a normal 22-week fetus. Amorphous material similar to that in G appears to arise from the specialized columnar ependymal cells (arrow). All images are hematoxylin and eosin stain unless specified otherwise.
Normal ependymal cells are variably immunoreactive for GFAP, vimentin, low molecular weight cytokeratins, CD99, epithelial membrane antigen, E cadherin, and S100 (33). Buried and luminal ependymal cells also often express ubiquitin, often in a cytoplasmic dot-like pattern (34). It may be involved in transcription factor turnover that is necessary to maintain the multiciliated state (35; Figure 30.2).
Although the ependymal lining starts as a continuous epithelium, focal breaks are common in the occipital horns and along the corpus callosum. An injurious process (e.g., infection, hemorrhage) may cause more widespread interruptions or buried rosettes, particularly where the ependyma lies on gray matter structures (Figure 30.2; 36, 37). Ependymal cells are not capable of proliferating sufficiently to replace the lost cells (38).
The central canal of the fetal spinal cord usually contains amorphous material, sometimes with a few cells that are CD68 or HLA-DR immunoreactive (i.e., macrophages). This resembles the Reissner’s fiber, which has been described in almost all mammals. It is formed by secretion from the subcommissural organ, which lies dorsal to the proximal cerebral aqueduct at the back of the third ventricle (Figure 30.2; 39–41; 42). In zebrafish, the Reissner’s fiber seems to be critical for maintaining a straight body axis during development (43). Another possible function is maintaining patency in the cerebral aqueduct and central canal (44). If both functions are true, it may help to explain why in teenage years the central canal disappears and is replaced by a jumble of ependymal cells (45).
References
Immune System Development
The development of the human immune system and responses have been reviewed in detail by others (1–3). Monocytes/macrophages, the earliest circulating immune cells, appear at the beginning of the fetal period. They are followed by neutrophils, lymphocyte precursors, and natural killer (NK) cells by gestational week 8–10, and then naïve T and B lymphocytes at week 12. The thymus (the source of T cells) grows rapidly during gestational weeks 7–14 and continues to grow, reaching its maximum size by the end of the first year of life, after which it involutes eventually replaced by fat at puberty (4).
During pregnancy, the maternal and fetal circulations exchange small numbers of cells (5). This condition, known as microchimerism, is associated with the persistence of fetal cells in the mother after delivery (6), and conversely persistence of maternal cells in the offspring (potentially including the brain). Immune tolerance to these non-inherited maternal antigens is critical during fetal development. Maternal non-tolerance to fetal cells can lead to erythrocyte Rhesus factor (Rh) disease, wherein a sensitized Rh-negative mother mounts a devastating immune response against the blood of a subsequent Rh-positive fetus (7). After birth, a hypoinflammatory state in the infant may be a necessary adaptation to tolerate microbial colonization of the gut and skin. Unfortunately, this adaptation renders infants susceptible to many infections (8). The ability to generate pro-inflammatory cytokines increases during the first year of life. In general, immune responses are low before 2 months of age. Although substantial by 1 year age, completely adult-like responses might not occur until after 10 years of age (1).
Microglia
Microglia are resident immune cells of the central nervous system (9). They are not derived from the neural tube. Progenitors of the monocyte/macrophage family invade from the circulating blood as the vasculature grows during the embryonic and early fetal period (from the 4th to at least the 24th gestational week; 10–12). Once the microglia enter the CNS, they establish a local ability to renew themselves (11). The density of microglial cells in the CNS is fairly uniform and remains stable over the human lifetime through constant self-renewal every ~95 days; the population is preserved by a balanced coupling of proliferation and apoptosis (13–15). Uniform distribution is facilitated by the motility of microglia (12). In human white matter (16), resting microglia have a small soma and delicate processes that radiate among other cells (“ramified” microglia; Figure 31.1 A). Microglial processes contribute to the human glia limitans around blood vessels (17). The ultrastructural features of normal and reactive microglia are well-characterized (18). Microglial nuclei are recognizable by their narrow diameter, pointed tips, and often irregular contour along with a generally dense chromatin pattern.
Although microglia are typically thought of in the context of inflammatory responses, they are firstly important in normal CNS development. They interact with endothelial cells during angiogenesis (19, 20). They are critical in the early development of axonal connections, synaptic refinement, and likely in the establishment of the entire neural network (21–25). Throughout life, they are critical in immune surveillance (26).
Figure 31.1 Demonstration of inflammatory cells in infant brains. A) Immunohistochemical demonstration of AIF1 (Iba1) in normal white matter. Perivascular cells and ramified microglia are labeled. However, not all presumptive microglia (based on nuclear morphology) are labeled (arrows). B) HLA-DR immunolabeling of microglia along the polymorphous layer of the dentate nucleus in an infant who died unexpectedly. C) Faint CD45 immunolabeling of ramified microglia in the polymorphous layer of the dentate nucleus in an infant who died unexpectedly. Note that not all cells with rod-shaped nuclei are labeled (arrows). D) RCA1 lectin labeling of all ramified microglia in the polymorphous layer of the dentate nucleus in an infant. E) CD68 immunolabeling of amoeboid microglia/macrophages in the CA1 sector of an infant who sustained severe hypoxic-ischemic brain damage and lived several days. F) CD3 immunolabeling of T lymphocytes in an infant with presumed viral encephalitis. Many lymphocytes are perivascular and confined by the glia limitans (arrows). Others are distributed throughout the cerebellar white matter. Figures A, B, C, E are 400x; D is 600x; and F is 200x original magnification. All counterstained with hematoxylin (blue).
Nonparenchymal Macrophages and Mast Cells in the Perivascular, Meningeal, and Choroid Plexus Compartments
Although distinct from microglia, these cells also originate from the circulating erythromyeloid precursors and enter the early fetal CNS (27). They are likely involved in immune surveillance, antigen presentation, and recruitment of circulating leukocytes during inflammation (28). Unlike microglia, which remain confined to the CNS, experimental evidence indicates that perivascular macrophages exchange regularly with circulating monocyte/macrophages (29). This cell population may be involved in the two-step entry of leukocytes cells into the CNS whereby migration across the vascular wall into the perivascular space and advancement across the glia limitans into the parenchyma are distinct processes (30). There is likely another distinct population of cells in the perivascular compartment, the pericyte, which is a smooth muscle-like contractile cell that serves a role in the maintenance of vascular integrity (31–34).
In parallel with the perivascular and meningeal macrophages, mast cells enter the developing brain (35). In the human brain, they persist and are concentrated in the infundibulum, pineal organ, area postrema, choroid plexus, and leptomeninges, while in the CNS parenchyma there are only very few (36). Understanding of the role of mast cells in the CNS remains limited (37–39).
Inflammation – Leukocytes from the Circulating Blood
In response to a variety of stimuli including bacterial infections (e.g., meningitis) and cerebral ischemia, neutrophil granulocytes are the first circulating cells to enter brain tissue or the leptomeningeal compartment. This typically begins at approximately 12 hours after an acute insult; concentrations peak at 2–3 days unless the stimulus persists. Glial chemokines activate endothelial cells to upregulate selectins and adhesion molecules on the luminal surface. Circulating neutrophils stick to E- and P-selectin. Contact with adhesion molecules (ICAM1 and VCAM1) leads to secure adhesion followed by diapedesis between endothelial cells to the site of inflammation. There, neutrophils release anti-microbial enzymes, chemokines, and reactive oxygen/nitrogen species that cause further attraction and activation of immune cells including macrophages. Within 1–2 days of extravasation, neutrophils undergo apoptosis and are phagocytosed by microglia or meningeal macrophages (40). Although important in the mature CNS (41–43), there is a minimal invasion of lymphocytes into the neonatal human brain following hypoxic-ischemic injury, perhaps because the progenitor cells are immature (44). However, in older infants invasion of CD3 immunoreactive T lymphocytes at sites of viral infection is robust (Figure 31.1 F).
Inflammation – Microglial Activation
Microglia are involved in basal immune surveillance in the CNS and respond in a subtle manner to normal neuronal activity (26). A variety of ion channels on microglial membranes make the cells sensitive to the extracellular environment and set the cell resting potential, which in turn influences the activation state (45). Microglial activation occurs rapidly (within hours of a stimulus) and persists for weeks. Activated microglia retract their processes and become more globoid (“amoeboid”) as they take on phagocytic properties. Macrophages can be recognized by their vacuolated cytoplasm. Microglial hyperplasia or hypertrophy into macrophage forms may be evident after a few days (46, 47). The extent to which human microglial proliferation increases beyond the normal turnover rate after injury or in disease is unclear (48).
Identification of Microglia in the Human Brain
Microglia can be detected and activation states shown using a variety of antibodies (Table 31.1; Figure 31.1). Among these are CD45, CD68, allograft inflammatory factor 1 (AIF1 / Iba1), and human leukocyte antigen major histocompatibility complex (MHC) class II (e.g., HLA-DR; 47, 49). At “rest,” microglia lack MHC-II expression, but when activated they are capable of MHC-II dependent antigen presentation (50). In general, anti-CD45 (at concentrations used for conventional detection of leukocytes) is the least sensitive. In this author’s experience there are several caveats: (1) these antigens cannot easily be used to demonstrate a hierarchy of activation states, particularly in the developing brain; (2) anti-HLA-DR may not be expressed in midgestation cases with overt damage; (3) anti-AIF1 does not always detect “resting” microglia (Figure 31.1A; 51). There is some evidence that microglial activation can undergo “polarization” into classic and alternative activation states, although it may be that this represents a continuum rather than alternate states (49). Irrespective of the above uncertainties, a positive immunostaining result can be used as a sensitive surrogate marker of irritated neurons or to localize sites of lost neurons when the neuron changes are not obvious histologically.
Table 31.1 Microglial antigens that can be detected by immunohistochemistry in routinely processed human brain tissue
Common /alternate name(s) | Comment | References |
---|---|---|
AIF1 (allograft inflammatory factor 1) / Iba1 (ionized calcium binding adaptor molecule 1) | Resting microglia in experimental animals but variable results in humans. Reduced expression in macrophages. | (72, 73) |
CD45 / LCA (leukocyte common antigen) / protein tyrosine phosphatase receptor type C | Activated microglia. | (74, 75) |
CD68 (cluster of differentiation 68) | Lysosome-associated membrane glycoprotein (LAMP) present in activated microglia and macrophages. | (76) |
CD163 (cluster of differentiation 163) | Perivascular macrophages and macrophage-like microglia. | (47) |
HLA-DR (human leukocyte antigen DR) / major histocompatibility complex, class II, DR | Antigen-presenting molecules on activated microglia. | (77) |
P2Y12 receptor (purinergic receptor P2Y12) | Expressed on resting parenchymal microglia from fetal to adult stages; absent from perivascular or meningeal macrophages. | (78) |
TMEM119 (transmembrane protein 119) | Labels activated microglia but not infiltrating macrophages (unlike most other markers). Use in fetal tissue not shown yet. | (79–82) |
Another method, less frequently available in clinical pathology laboratories, is the labeling of microglial surface carbohydrates with lectin histochemistry (52). Tomato lectin and Ricinus communis agglutinin (RCA) lectin can label resting microglia in fetal and infant human brains (16, 53). An even less common approach now is enzyme histochemistry on fixed-frozen brain tissue to detect, for example, nucleoside diphosphatase (NDPase) in microglia (54).
Inflammation as a Cause of Brain Damage
There is direct evidence in adult humans that systemic inflammation, in the absence of CNS infection, can activate microglia, particularly in the white matter (55). Considerable epidemiologic and indirect data suggest that systemic maternal, fetal, or infant inflammation is associated with adverse neurological outcomes (56–61). These situations are not associated with brain damage that is detectable on a routine neuropathological examination. A nuanced interpretation is that altered microglial activation interferes with normal synaptic development in the growing brain rather than damage per se (23, 62, 63). In the mature brain, uncoupling of neuron–microglia interactions might contribute to altered neuroplasticity and be associated with neuropsychiatric symptoms (64).
Inflammation is first considered as a protective and janitorial response to an insult. Phagocytosis by microglia and blood-derived macrophages is a necessary removal process of pathogens, apoptotic cells, and cellular debris (65). It is also possible that inflammation might potentiate brain damage. Perinatal inflammation associated with materno-placental infection or meningitis is a risk factor of neonatal arterial ischemic stroke because it can cause vasospasm, emboli, or focal arteritis and thrombosis (66). Perivascular mast cells, which are sparse normal residents in the central nervous system (36), have been implicated in the pathogenesis of neonatal hypoxic-ischemic brain injury; factors released from activated mast cells can contribute to blood-brain barrier damage, brain edema, and neutrophil recruitment (67). Microglia and macrophages serve the beneficial role of phagocytosing debris and microbial pathogens in the injured brain. Cytokines and complement components are among the many mediators of normal immune functions. However, pro-inflammatory cytokines such as tumor necrosis factor alpha and interleukins 1 beta, 4, and 6 can disrupt the blood-brain barrier and cause secondary damage to immature neurons directly (68). Abnormal expression of interferons (IFN) and TNF-alpha can lead to developmental damage in many organ systems, including the CNS (2). In genetic disorders that cause overproduction of type 1 interferon, the pattern of brain damage, including intracranial calcification and inflammation, resembles that of congenital infection. Thus Aicardi-Goutieres syndrome is sometimes referred to as pseudo-TORCH syndrome (69, 70). Despite the potentially deleterious effects, microglial responses have evolved to be beneficial in the grand scheme (71).
References
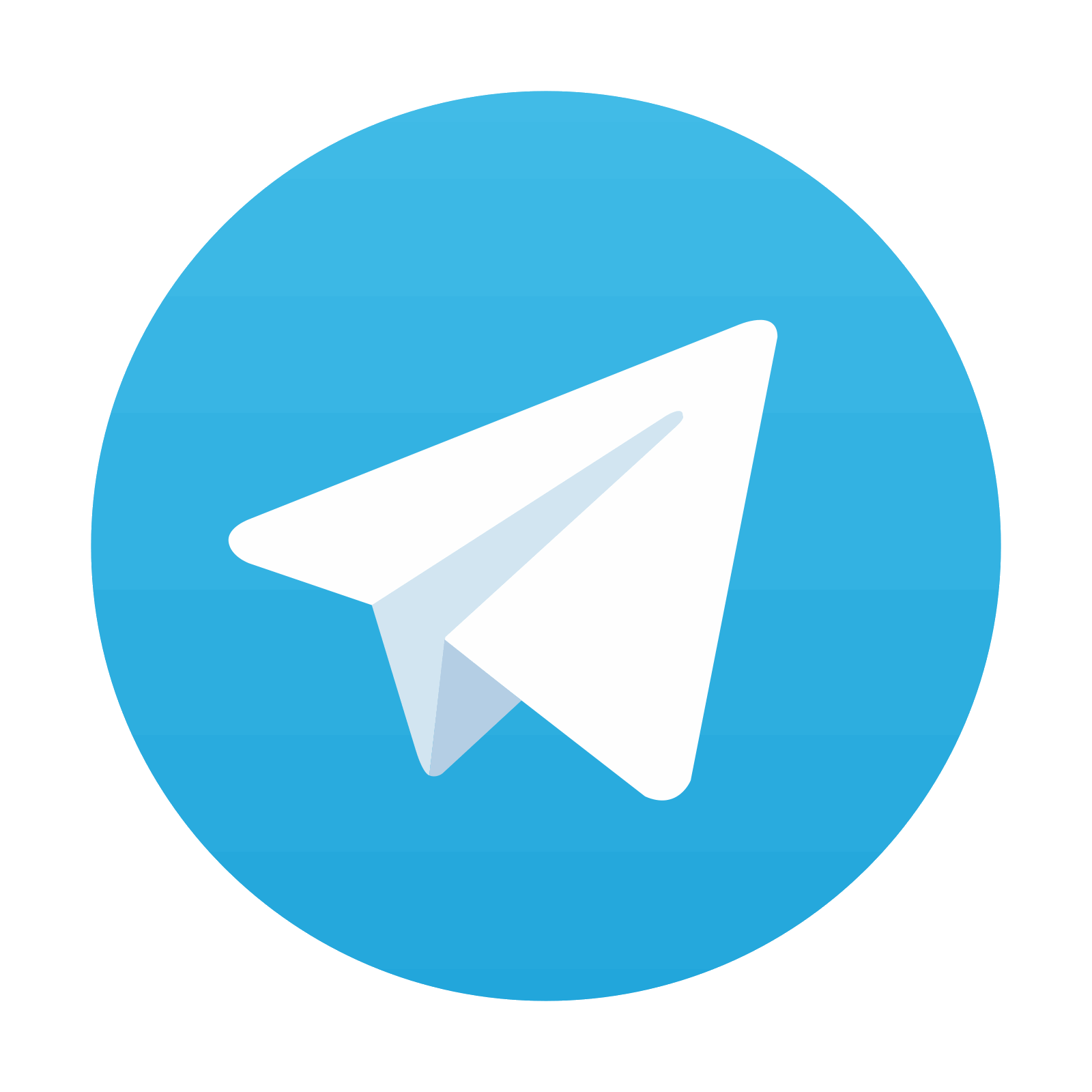
Stay updated, free articles. Join our Telegram channel
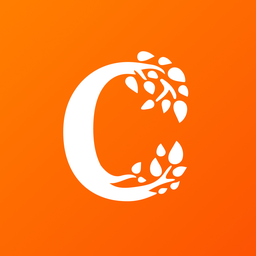
Full access? Get Clinical Tree
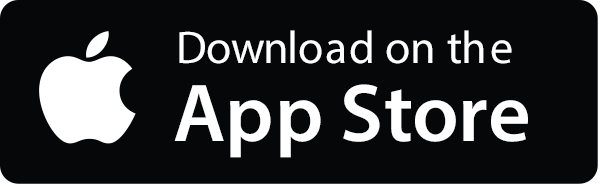
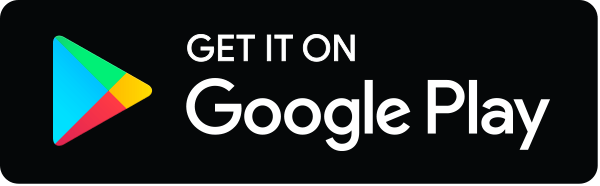