The word artifact is derived from the Latin words arte (“by skill”) and factum (“thing made”) and originally referred to things made by skill. The sense of the word artifact in biology and pathology is regressive and refers to features that are not true characteristics of the object of study. Perinatal neuropathology is bedeviled by artifacts because of the inherently soft nature of the immature brain and the susceptibility to autolytic processes. It is critical that the pathologist understand the nature of these artifacts. Note that these changes are especially obvious in brains of stillborn infants (see Chapter 28). In addition to macroscopic and microscopic structures, autolysis can also adversely affect immunoreactivity and the quality of RNA and DNA (1, 2).
Artifacts must be interpreted in the context of when they are introduced. Prefixation artifacts include those that occur between the time of death (or tissue devitalization) and fixation, including those due to handling. Certain artifacts almost always occur during fixation, while some may occur during microtomy and handling. This chapter will not cover general artifacts of fixation and microscopy (3–6).
Maceration
Maceration is defined as the softening of a solid by the action of a liquid. In the context of perinatal pathology, this usually refers to the uptake of amniotic fluids and sloughing of skin from stillborn fetuses. In myelomeningocele, rachischisis, and anencephaly, central nervous tissue is potentially exposed to amniotic fluid (7). In this context, the maceration is not an artifact but is actually a contributor to the degenerative process. It is not clear if the subpial and ventricular surfaces of the brain are subject to maceration from cerebrospinal fluid after death. Although this is probably a minor issue, occasionally external surfaces of the spinal cord can have a vacuolated appearance on microscopic examination. Organs removed at autopsy and immersed in water for excessive periods prior to fixation can also become macerated (8).
Autolysis
Autolysis is the breakdown of cells or tissues through the release and/or deregulated activation of endogenous proteolytic enzymes. Postmortem degradation of the brain is predominantly due to autolysis. Mature brain tissue contains cathepsins and other endopeptidases, but few lipolytic enzymes; these can contribute to autolysis (9–11). Immature brains are subject to more rapid and severe autolytic degradation, which can extend to the point of complete liquefaction. This can be explained on the grounds of endogenous enzymes that are involved in cell migration and vasculogenesis during the period of rapid brain growth (12–16). Because enzyme kinetics are temperature dependent (17), autolysis at body temperature (e.g., in a fetus following intrauterine death) occurs more quickly than autolysis in a refrigerated body (e.g., in a delayed autopsy situation). However, cooling to below 0˚C can be problematic. A body that is frozen will develop ice crystal artifacts (see Figure 27.1). If frozen for a long period, tissues may degenerate by freeze-drying (e.g., mummification).
Figure 27.1 Photomicrograph showing linear freeze crystal artifact in the putamen of a 1-week infant whose body was frozen after death. Hematoxylin and eosin (H&E) stain; original magnification 40x.
The gross features of autolysis include tan to dark brownish discoloration of brain and softening, with fragmentation and eventual liquefaction (see Figure 27.2). Gross morphology should be assessed and the brain photographed in situ before handling, because some aspects of the gyral details may be lost (see also the section on “Artifactual Pseudodeformities of the Brain”). There are no reliable markers for estimating the time of in utero death prior to delivery based upon the severity of autolysis.
Figure 27.2 Gross features of brain autolysis. A – Photograph showing a lateral view of a stillbirth brain, approximately 38 weeks gestation. The gyral pattern is intact and the brain is of normal size, but the entire brain has a tan discoloration. B – Upon slicing, the brain fragments easily, but normal gross anatomical features are retained. C – Photograph showing lateral surface of a stillbirth brain, approximately 21 weeks gestation. It is more severely autolytic with dark brown discoloration and generalized friability. D – Photograph showing fragments of a 24-week gestation stillbirth brain with moderately advanced autolysis and partial liquefaction.
Microscopic changes occur in autolytic brain tissue. Cracking along the external surfaces and irregularities in periventricular germinal tissue density are signs of autolysis (see Figure 27.3). Postmortem brain tissue often has a spongy appearance. This may be due to watery expansion of the cytoplasm (which could reflect antemortem edema) or clearing of the pericellular spaces due to shrinkage of neurons away from the surrounding neuropil (see the section “Fixation and Overfixation”). As a consequence of autolysis, nuclear chromatolysis begins at ~24 hours (18) and is pronounced with degradation of the nucleolus by 3 days (19). The neuropil may be discontinuous as synaptic terminals break up (20). In myelinated tissue, a spongy appearance occurs when myelin delaminates because myelin basic protein is susceptible to acid proteases in brain tissue (21–25). Autolytic dissolution of the internal granular layer of the cerebellum seems to depend more on the pH of the tissue than the postmortem interval, and therefore might be in part reflective of hypoxic-ischemic stress (26, 27). In spite of fairly advanced autolytic fragmentation, the microscopic detail of cells can be remarkably intact, allowing for evaluation of normal developmental features and absence of inflammatory foci.
A – Cracks at the surface of a 21-week stillbirth brain are easily recognized as artifact; cortical lamination is normal. H&E stain; original magnification 200x.
B – Irregular pale areas (arrows) in the ganglionic eminence of a 26-week stillborn fetus are due to autolytic degradation of the germinal cells. H&E stain; original magnification 40x.
C – Autolytic changes in the nucleus of a cortical neuron from a 25-week stillbirth brain; the chromatin is slightly condensed. H&E stain; original magnification 1000x.
D – Autolytic changes in the nucleus of a cortical neuron from a 33-week stillbirth brain; the chromatin is more fragmented and the surrounding neuropil vacuolated. H&E stain; original magnification 1000x.
E – Autolytic changes in the cerebellar internal granule layer of a 6-month infant who died suddenly in bed and whose autopsy was delayed. H&E stain; original magnification 600x.
Putrefaction
Putrefaction is the consequence of bacterial growth in tissue. The usual culprits are anaerobic bacteria from the intestine, which cross the blood-gut barrier after death and grow in the vascular system, often entering tissues including the brain. Putrefied brains are soft with a paste-like texture (in contrast to the liquid nature of advanced autolysis). Anaerobic bacteria can produce nitrogen, CO2, hydrogen, and small amounts of methane and hydrogen sulfide (28), thereby forming pockets of malodorous gas in affected tissue (“Swiss cheese artifact”; see Figure 27.4). Another subtler change related to putrefaction is the digestion of DNA and RNA in brain tissue by bacterial nucleases (1). Associated nuclear fragmentation and eventual loss of hematoxyphilia in the nuclei and Nissl substance should not be mistaken for antemortem damage (e.g., hypoxia-ischemia). The fetal intestine is sterile, so putrefaction in fetal stillbirth tissue is rare and most likely associated with amniotic infection. Unless deliberately hidden, neonatal and infant deaths are usually discovered within hours. Therefore, putrefaction is typically not a major confounder in these cases unless there is antemortem sepsis or the body is not properly stored prior to autopsy (29). If putrefaction is anticipated, some authors advocate cooling the head to facilitate removal of the brain in a more intact state (30). Putrefaction may obscure postmortem microbiologic tests (31).
A – Large vacuoles (“Swiss cheese” artifact) due to gas production by bacteria in the putamen. H&E stain; original magnification 12.5x.
B – Intravascular bacilli (Gram-negative) in the putamen. H&E stain; original magnification 600x.
C – Putrefaction-association nuclear degradation in the putamen. Chromatin fragmentation (arrow) resembles that seen in apoptosis; eventually, only ghosts of nuclei will remain. Note bacteria infiltrating tissue. H&E stain; original magnification 1000x.
Fixation and Overfixation
Hardening of brains, to facilitate dissection and processing for histology, is usually achieved by immersion in 10% neutral buffered formalin, which is a solution of 3.7%–4.0% formaldehyde in phosphate-buffered saline. Formaldehyde (methanal) is a gas at room temperature; a saturated aqueous solution is 40% formaldehyde by volume or 37% by mass (hence, the 10% formalin solution refers to 10% of the saturated formaldehyde solution). Formaldehyde reacts to form covalent methylene bridges between exposed amino groups on arginine, cysteine, histidine, glutamine, tryptophan, and lysine as well as amino groups on the nucleotides of DNA and RNA (32–34). The resultant cross-linking of proteins and nucleic acids makes the tissue firm and preserves the constituents. Formaldehyde penetration by diffusion occurs at approximately 1 mm per hour initially and slower thereafter. Covalent crosslinking is slower, likely requiring approximately 24–48 hours at room temperature (35, 36); both diffusion and chemical fixation are temperature dependent (37). Assuming an adequate supply of formaldehyde in solution, an average of 32 days is required before formaldehyde reaches the depths of adult brains immersed in fixative (38). Thus postmortem degradative changes are worse in tissue distant from an exposed surface. Tissue shrinkage occurs during formalin fixation (39), which can lead to artifactual retraction spaces around cells (especially between neurons and neuropil); apparent cell sizes are also reduced (40). Formaldehyde fixation can create a beading artifact along neuronal processes (41).
Prolonged fixation (“overfixation”) can lead to excessive cross linking of peptides, deamination of cytosine bases, or Eschweiler–Clarke methylation of amines on fatty acids and peptides (42). The potential consequences include masking of immunohistochemical stains (43) and interference with some DNA analyses (44). The latter reaction can also interfere with postmortem toxicology (45).
Fixed brains are typically rinsed prior to examination and slicing. Because the aldehyde bonds are reversible (46), this should be done in cold water for <18 hours (i.e., no longer than overnight). A prolonged alcohol transition stage during embedding might contribute to tissue vacuolation (47).
Embalming
Occasionally one is required to examine a brain from a previously embalmed body. The goal of embalming is temporary preservation of a body by intravascular perfusion of fixatives and antimicrobials. Embalming solutions are various combinations of formaldehyde, glutaraldehyde, alcohols, phenol, and other chemicals (48). The texture of the brain may differ from that fixed in formalin and the immunodetection of some antigens might be reduced.
Artifactual Pseudomalformations of the Brain
Prior to, during, and rarely after fixation, immature brains are predisposed to deformation, particularly those with some degree of autolysis. The most important artifacts to recognize are the distortions that mimic gyral abnormalities and migration disorders. The leptomeninges have greater tensile strength than the underlying brain tissue, and therefore mechanical disruptions of the brain surface might not be obvious. Brains wrapped in gauze during fixation can develop fine grooves reminiscent of polymicrogyria (see Figure 27.5). Rarely, shrinkage of the meninges can fold the underlying brain and create false sulci. Cracks that develop in brain tissue are usually obvious as artifacts. However, small convolutions and eruptions along the cortical surface can resemble migration abnormalities. These were recognized as common artifacts by embryologists in the early 1900s (49, 50). Regional misalignment of tissue blocks can resemble focal dysplasia, and dislodged tissue fragments can resemble leptomeningeal heterotopia. While not an artifact, glial sleeves along penetrating blood vessels can resemble leptomeningeal heterotopia, particularly if cut in a plane where the vessels are not obvious. During slicing of the fixed brain, individual cells can be dislodged. When this happens to Purkinje neurons, they can be mistaken for heterotopic cells in the leptomeningeal compartment (51).
Figure 27.5 Pseudomalformation artifacts. A – 22-week, slightly autolytic fetal brain that had been wrapped in gauze during fixation. A fine striate pattern from gauze fiber impressions mimics polymicrogyria on the lateral surface. B – Medial surface of the slightly autolytic brain from a 15-week fetus. Contraction of the leptomeninges compressed and folded the brain to create false sulci; the lateral surface was normal. C – Same case. H&E stain; original magnification 12.5x. D – False gyral irregularities on the lateral frontal surface of a 21-week fetal brain with mild autolysis. H&E stain; original magnification 40x. E – Fountain-like cortical irregularities on the lateral frontal surface of a different 21-week fetal brain with mild autolysis. H&E stain; original magnification 100x. F – Focal cortical misalignment in the brain of a 26-week stillbirth. Dislodged columns of neurons (arrows) resemble focal dysgenesis. H&E stain; original magnification 200x. G – Dislodged (arrow; likely during slicing) piece of soft cortex in the subarachnoid space of a 35-week stillborn brain; this must not be mistaken for a leptomeningeal heterotopia. H&E stain; original magnification 40x. H – Perivascular glial sleeve (arrow) in the choroidal fissure of a 4-month infant. Cut in a plane with the vessel hidden, these can be mistaken for glial heterotopia. H&E stain; original magnification 200x. I – Displaced Purkinje neuron on the surface of an infant’s vermis. H&E stain; original magnification 600x.
Hemorrhage and Pseudohemorrhage
Blood can contribute to problems with histopathologic evaluation in at least four ways: (1) A blood collection of substantial size adds to the total protein mass of the tissue and can exhaust the formaldehyde rapidly thereby preventing proper fixation of the brain unless fresh formalin is added. (2) If fixed too long, blood clots can become extremely hard and will distort or damage adjacent brain tissue during slicing. (3) Intravascular erythrocytes are potentially mobile after tissue sectioning, before the mounting medium has hardened. Pressure on the coverslip can displace blood; it is often in a different focal plane than the brain or straddling an intact blood vessel wall (see Figure 27.6). (4) Staining of brain surfaces by hemoglobin from lysed erythrocytes was recognized long ago (52). Formaldehyde reaction with heme (from degrading hemoglobin) creates acid hematin, which is sometimes called “formalin pigment”; it appears as dark brown to black irregular intra- or extracellular crystals (see Figure 27.6; 53, 54). Hematin or bilirubin may aggregate in tissues as orange crystals; these do not stain with the Perls’ Prussian blue method, which reacts with ferric iron (Fe3+) in hemosiderin.
A – Erythrocytes displaced from artery lumen (top left) across the vessel wall and into the subarachnoid compartment. If the overlap is not obvious, this can be mistaken for subarachnoid hemorrhage. H&E stain; original magnification 400x.
B – Acid hematin (“formalin pigment”; brown-black granules) in cells near the pial surface. H&E stain; original magnification 600x.
C – Bilirubin crystals in the pituitary of a 31-week stillborn infant. H&E stain; original magnification 1000x.
Extravasation of blood into skull sutures or onto the brain surface often occurs in the context of stillbirth. If the brain softens in situ, the skull may be distorted with overlapping sutures, often with hemorrhagic discoloration (see Figure 27.7). Autolytic blood vessels, which are often engorged, become weakened. Whether it occurs at the time of delivery or during removal of the brain is not clear. These passive collections are typically thin and patchy (see Figure 27.7). Intradural hemorrhage (i.e., blood cells amongst the collagenous layers of the dura, not in the subdural compartment) has been associated with hypoxia (55). In at least some of these cases, when the skull is opened and the dura mater is stripped from the inner surface, some of the intradural blood vessels may be damaged, leading to the spread of blood. Hematomas of significant thickness on or within the brain almost certainly occur during life. The importance of recognizing these pseudohemorrhagic artifacts is to avoid invoking antemortem trauma as an explanation for the death.
A – Vertex view of the skull of a 35-week stillborn. Softening of the underlying brain allows the frontal and parietal bones to separate across the coronal suture (arrow), and there is considerable subperiosteal blood.
B – Posterior exposure of the brain by removal of occipital bone from a 32-week stillborn showing subarachnoid hemorrhage.
C – Medial surface of the cerebral hemisphere from a 25-week stillborn showing small foci of subarachnoid hemorrhage in sulci.
Dark Neurons
Dark neurons are characterized by nuclear pyknosis, cytoplasmic hyperchromasia, and irregular dendrites (56). They may appear in clusters or scattered among histologically normal neurons. They are common and must be recognized to avoid confusion with hypoxic-ischemic neuronal damage (57–59). Unlike dead neurons, the nucleolus can often be distinguished within the pyknotic nucleus. One circumstance in which they arise is mechanical handling of brain tissue prior to complete fixation (60–62; see Figure 27.8), but they can also appear after seizure, hypoglycemia, electrical shock, and ischemia (63). The cellular mechanism appears to include glutamate stimulation and collapse of the cytoskeleton (64, 65). Notably, with respect to perinatal neuropathology, immature neurons in rodent cerebellum are less susceptible to the dark neuron artifact (66).
Figure 27.8 Dark neurons (arrows) among normal-appearing neurons near a laceration in the frontal cortex of an infant who died immediately after his head was driven over and crushed by a motor vehicle. H&E stain; original magnification 200x.
References
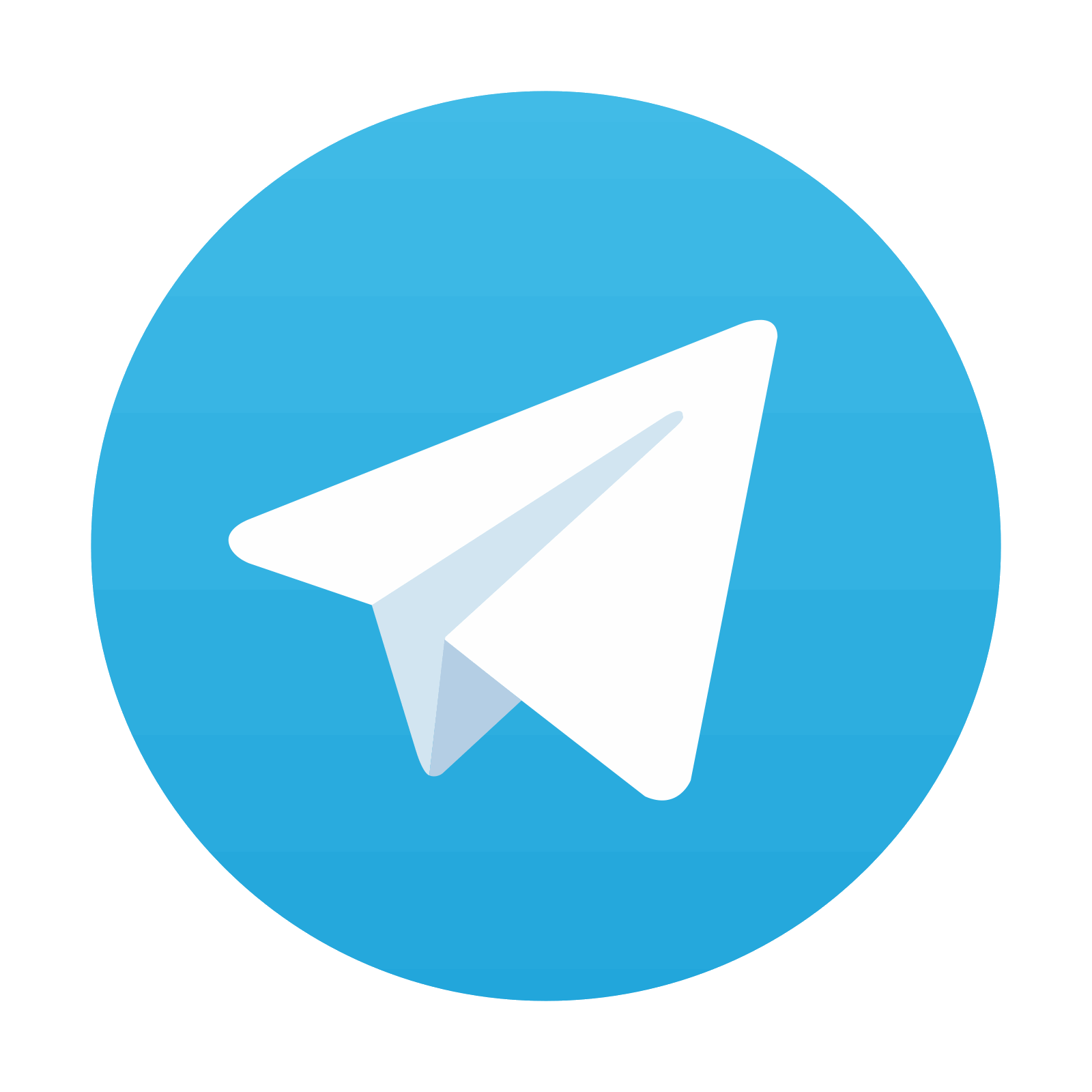
Stay updated, free articles. Join our Telegram channel
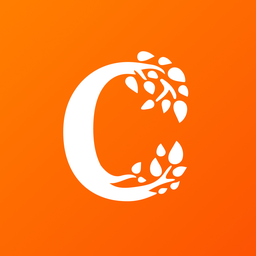
Full access? Get Clinical Tree
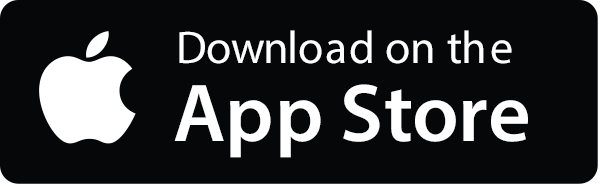
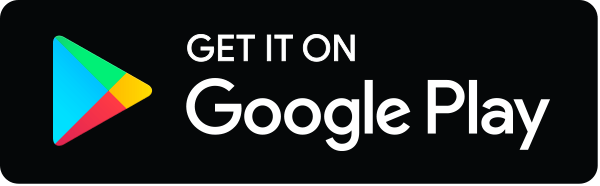