When one considers the complexity of the pulmonary and hemodynamic changes occurring after delivery, it is surprising that the majority of infants make the transition from intrauterine to extrauterine life so smoothly and uneventfully. Nonetheless, the staff working in the intensive care nursery spends a lion’s share of their time caring for neonates with respiratory problems that are responsible for much of the morbidity and mortality in this period.
Physiologic Considerations
Normal Developmental Changes
Before birth, the lung is a fluid-filled organ receiving 10% to 15% of the total cardiac output. Within the first minutes of life a large portion of the fluid is absorbed or expelled, the lung fills with air, and the blood flow through the lung increases eight- to tenfold. This considerable increase results from a decrease in pulmonary arterial tone and other physiologic changes that convert the circulation from a parallel arrangement to a series circuit.
The high vascular resistance in the fetal lung is caused by pulmonary arterial vasoconstriction. The pulmonary arterial vasodilation observed following delivery results, in part, from the large increase in oxygen tension, from the small decrease in CO 2 tension, and the corresponding increase in pH, biochemical changes, such as elevated prostaglandins, and from the mechanical effect of lung inflation.
At the same time, an adequate functional residual capacity (FRC = volume of air in the lungs at end expiration) is quickly attained. In healthy term infants, the first breaths are characterized by short deep inspirations and prolonged expirations through a partially closed glottis to ensure lung inflation. In preterm infants, continuous positive airway pressure (CPAP) enhances lung inflation. By 1 hour, the distribution of air with each breath in the newborn is already similar to that observed in later life. Specifically, lung compliance (change in lung volume expressed in mL of air/cm of H 2 O pressure change/mL of lung volume) and vital capacity increase briskly in the first hours of life, reaching values proportional to those in the adult.
Chemical control of respiration is, in general, similar in the newborn infant and the adult. As inspired (and arterial) P co 2 is increased, both infants and adults increase their ventilation, although the neonatal ventilatory response is smaller. The ventilation of the newborn is also transiently increased when inspired gas mixtures contain less than 21% oxygen; this response suggests that the carotid body chemoreceptors are active at birth. The infant, however, differs from the adult in that if hypoxic exposure continues beyond about 1 minute, respiration is depressed during the first weeks of life. Hypoxia thus appears to depress the respiratory center, negating the hypoxic stimulation via peripheral chemoreceptors. This hypoxic respiratory depression in the newborn appears to depend on the presence of suprapontine structures in the brain. Even though hypoxic respiratory depression may be useful to the fetus (who maintains a normal Pa o 2 of 20 to 25 mm Hg), persistence of this phenomenon into postnatal life may enhance vulnerability of neonatal respiratory control.
The effects of pulmonary stretch receptor activity on the timing of respiration (Hering-Breuer reflex) are more readily elicited in the newborn than in the adult. In infants, a sustained increase in FRC causes a marked slowing of respiratory rate by prolonging expiratory time. In the first days of life, a brisk lung inflation causes a deep gasp (Head’s paradoxical gasp reflex) followed by apnea, which is, again, a manifestation of the Hering-Breuer inflation reflex. The deep gasp observed in the first day of life with low inflation pressures may explain the clinical observation that very low pressures (10 to 15 cm H 2 O) are often effective in resuscitating the apneic newborn at birth by stimulating a gasp reflex.
The partial pressure of carbon dioxide (P co 2 ) reflects the ability of the lung to remove CO 2 . The HCO 3 concentration is controlled by the kidney. When the pH and CO 2 are determined, the HCO 3 can be calculated by using the Henderson-Hasselbalch equation:
pH = 6.1 + log HCO 3 Pco 2 × 0.03
If only the pH is measured, the cause of the acidosis or alkalosis cannot be determined. With metabolic acidosis, HCO 3 is decreased. To compensate for this, the infant hyperventilates, lowering arterial P co 2 . With respiratory acidosis caused by pulmonary disease, apnea, or hypoventilation, the arterial P co 2 increases. The kidney attempts compensation by retaining HCO 3 and excreting hydrogen ions. Only by measuring the P co 2 and pH and calculating HCO 3 can the cause of an abnormality in acid-base balance be determined. The normal newborn quickly regulates his or her pH to near adult values, although HCO 3 may be lower than normal adult values.
Oxygen Delivery
Oxygen is carried in the blood in chemical combination with hemoglobin and also in physical solution. The oxygen taken up by both processes depends on the partial pressure of oxygen (PO 2 ).
At ambient pressures, the amount of dissolved oxygen is only a small fraction of the total quantity carried in whole blood (0.3 mL O 2 /dL plasma/100 mm Hg at 37° C). Most of the oxygen in whole blood is bound to hemoglobin (1 g of hemoglobin can maximally bind to 1.34 mL of oxygen at 37° C). The quantity of oxygen bound to hemoglobin depends on the partial pressure and is described by the oxygen dissociation curve ( Fig. 10-1 ). The blood is almost completely saturated ∗
∗ The arterial oxygen saturation is the actual oxygen bound to hemoglobin divided by the capacity of hemoglobin for binding oxygen. <SPAN role=presentation tabIndex=0 id=MathJax-Element-2-Frame class=MathJax style="POSITION: relative" data-mathml='%sat.=mLO2boundtoHbHb(g)×1.34×100′>%sat.=mLO2boundtoHbHb(g)×1.34×100%sat.=mLO2boundtoHbHb(g)×1.34×100
% sat . = mL O 2 bound to Hb Hb ( g ) × 1.34 × 100

As an example, if the arterial PO 2 is 50 mm Hg, saturation is 90%, and hemoglobin (Hb) is 10 g/dL, then 9 g Hb is bound to oxygen. Thus, the oxygen content of this 100 mL sample is 12.06 mL O 2 bound to Hb (1.34 × Hb 9) + 0.15 mL O 2 (0.3 × Hb 50/100) dissolved in plasma for a total of 12.21 mL O 2 . Naturally, if the hemoglobin is doubled, then for the same saturation, the O 2 transported by hemoglobin is also doubled (1.34 × Hb 18 = 24.12 mL O 2 ) without changing the amount dissolved. The dissociation curve of fetal blood is shifted to the left and, at any Pa o 2 less than 100 mm Hg, fetal hemoglobin binds to more oxygen compared to adult hemoglobin. The shift is the result of the lower affinity of fetal Hb for diphosphoglycerate (DPG). In contrast, the dissociation curve is shifted to the right by increasing acidosis and temperature. The clinical significance of the shift to the left for fetal Hb is that fetal blood will take up more oxygen at a given O 2 tension (PO 2 ). However, tissue PO 2 will need to decrease to a lower level to unload adequate oxygen. Thus, oxygen delivery to the tissues is determined by a combination of cardiac output, total hemoglobin concentration, and hemoglobin oxygen affinity in addition to arterial PO 2 .
The shift in dissociation curve induced by fetal hemoglobin makes clinical recognition of hypoxia (insufficient amount of oxygen molecules in the tissues to cover the normal aerobic metabolism) more difficult, because cyanosis is observed at a lower oxygen tension. Cyanosis is first observed at saturations from 75% to 85%, which correspond to oxygen tensions of 32 to 42 mm Hg on the fetal dissociation curve. Cyanosis in the adult is observed at higher tensions. The flattening of the upper portion of the S -shaped dissociation curve makes it almost impossible to monitor oxygen tensions above 60 to 80 mm Hg by following arterial oxygen saturation. Although the shape of the oxygen dissociation curve limits the usefulness of pulse oximetry to detect high Pa o 2 values, keeping saturation measured via pulse oximeter between 92% and 95% is one of the most effective and practical ways of reducing the risk of hyperoxemia.
The partial pressure of oxygen in arterial blood not only depends on the ability of the lung to transfer oxygen, but also is modified by the shunting of venous blood into the systemic circulation through the heart or lungs. Breathing 100% oxygen for a prolonged time partially corrects desaturation resulting from alveolar hypoventilation, diffusion abnormalities, or ventilation/perfusion inequality. Measurements of Pa o 2 while breathing 100% oxygen are, therefore, useful diagnostically in determining whether arterial desaturation is caused by an anatomic right-to-left shunt, in which case oxygenation will fail to improve the condition (hyperoxia testing).
After birth, Pa o 2 increases to between 60 and 90 mm Hg. During the first days of life, 20% of the cardiac output is normally shunted from right to left, in either the heart or lungs. When the normal adult breathes 100% oxygen, Pa o 2 increases to 600 mm Hg as compared with approximately 300 to 500 mm Hg in healthy neonates, which results from the substantial shunting in infants.
At the end of the first hour of life, perfusion of the lung is distributed in proportion to the distribution of ventilation. In the healthy newborn baby, oxygen saturations rise slowly over the initial few minutes of life, however, they do not reach 90% in the first five minutes. The postductal oxygen saturation levels are usually lower than the preductal measurements for as long as 15 minutes, indicating a persistence in elevated pulmonary vascular resistance for a significant period of time after birth ( Fig. 10-2 ). The speed with which pulmonary ventilation and perfusion is uniformly distributed is an indication of the remarkable adaptive capacities of the newborn infant for the maintenance of homeostasis.
Dawson et al. defined the reference ranges for pulse oxygen saturation (Spo 2 ) values in the first 10 minutes after birth for 468 infants who received no medical intervention in the delivery room. For all 468 infants, the 3rd, 10th, 50th, 90th, and 97th percentile values at 1 minute were 29%, 39%, 66%, 87%, and 92%, respectively; those at 2 minutes were 34%, 46%, 73%, 91%, and 95%; and those at 5 minutes were 59%, 73%, 89%, 97%, and 98%. It took a median of 7.9 minutes (interquartile range: 5 to 10 minutes) to reach an Spo2 value of >90%. Spo 2 values for preterm infants increased more slowly than those for term infants (see also Chapter 3 ).

Practical Considerations
Oxygen Therapy
Oxygen supplementation is critical for the survival of many infants with respiratory problems. Previous restricted use resulted in an increase not only in mortality rate, but also in neurologic handicaps. Additionally, recognition of the toxic effects of excessive or prolonged oxygen therapy is imperative when treating newborn infants. This has resulted in curtailed use of supplemental oxygen during neonatal resuscitation of both term and preterm infants (see Chapter 3 ). Therefore, oxygen administration must be performed with great precision, while carefully monitoring arterial oxygen tension or assessing oxygenation via noninvasive techniques.
Oxygen Administration
For spontaneously breathing infants, a small hood to provide supplemental oxygen prevents fluctuations in inspired oxygen when opening the incubator. This has been largely replaced by low flow nasal cannulae to deliver gas mixtures. Because improper oxygen administration can be detrimental, the following practical considerations should be highlighted:
- 1.
Peripheral cyanosis may be present in a neonate with a normal or high arterial oxygen tension.
- 2.
Inspired oxygen concentration should be continuously monitored in all infants receiving supplementary oxygen or assisted ventilation.
- 3.
Oxygen therapy without concurrent assessments of arterial oxygen tension is dangerous. A noninvasive monitoring device to measure oxygen saturation by pulse oximetry or transcutaneous PO 2 should be used continuously in preterm infants receiving any supplemental oxygen. In the presence of an arterial line during the acute phase of illness, measure Pa o 2 at least every 4 hours if the infant is receiving oxygen.
- 4.
In preterm infants, arterial oxygen tension should be maintained between 50 and 80 mm Hg during the acute phase of respiratory failure. In the absence of available Pa o 2 monitoring, SaO 2 should be kept in the 90% to 95% range, although this remains a subject of intense investigation.
- 5.
The development of retinopathy of prematurity (ROP) is related to high arterial oxygen tension levels, and these may rise above the normal range even with relatively low inspired oxygen concentrations. Whereas initial hyperoxia stunts retinal vascular development, later hypoxia appears to stimulate damaging vascular proliferation.
- 6.
When infants receiving supplemental oxygen require mask-and-bag ventilation, both oxygen concentration and inflating pressures must be monitored closely.
- 7.
Use of a nasal cannula for prolonged oxygen therapy allows greater mobility for the infant and enables oral feeding without manipulating oxygen concentration. Both inspired oxygen concentration and flow rate are precisely adjusted and the infant’s oxygenation is closely monitored, typically via pulse oximetry. Administration of oxygen by nasal cannula requires close monitoring because in active infants, the cannula is easily displaced from the nose. Also, changes in respiratory pattern and oral breathing may entrain different amounts of room air around the prongs, changing the true inspired oxygen concentration. Finally, high gas flows via nasal prongs are under evaluation, although safety concerns remain.
- 8.
When the infant with respiratory distress syndrome (RDS) is improving, environmental oxygen should be lowered in small decrements while continuously monitoring oxygenation.
- 9.
Any inspired oxygen concentration above room air can be damaging to pulmonary tissue if maintained over several days. Oxygen therapy is continued only if necessary.
- 10.
All premature infants 30 weeks’ gestation or less, or older if they had an unstable course or need for significant supplemental oxygen, receiving additional oxygen for extended periods of time should be examined by an experienced ophthalmologist by the later of 31 weeks’ postmenstrual age (PMA) or four weeks after birth for treatable retinopathy of prematurity (ROP).
Carlo et al. performed a randomized trial with a 2-by-2 factorial design to compare target ranges of oxygen saturation of 85% to to 89% or 91% to to 95% among 1316 infants who were born between 24 weeks 0 days and 27 weeks 6 days of gestation. The primary outcome was a composite of severe retinopathy of prematurity (defined as the presence of threshold retinopathy, the need for surgical ophthalmologic intervention, or the use of bevacizumab), death before discharge from the hospital, or both. All infants were also randomly assigned to CPAP or intubation and surfactant. The rates of severe retinopathy or death did not differ significantly between the lower oxygen saturation group and the higher oxygen saturation group. However, death before discharge occurred more frequently in the lower oxygen saturation group (in 20% of infants versus 16%; relative risk, 1.27; 95% CI, 1.01 to 1.60; P = .04), whereas severe retinopathy among survivors occurred less often in this group (8.6% versus 17.9%; relative risk, 0.52; 95% CI, 0.37 to 0.73; P <.001). There were no significant differences in the rates of other adverse events. These results have been replicated in another trial that terminated prematurely. Hence, because of concerns regarding the increased mortality in the lower oxygen saturation group, despite the better retinopathy outcome, the recommendation has been to maintain the oxygen saturation between 90% and 95%.
Neonatal Problems
Diagnosis
The initial objective is to establish an etiologic diagnosis for any observed respiratory symptoms. A major error in care can easily be made if other organ systems are not considered initially. Not every rapidly breathing infant has respiratory distress syndrome or even respiratory disease. Hypovolemia, hyperviscosity (polycythemia), anemia, hypoglycemia, congenital heart disease, hypothermia, metabolic acidosis of any etiology, or even the effects of drugs or drug withdrawal may all mimic primary respiratory disorders. Appropriate care depends on the diagnosis. For example, rewarming should rapidly relieve respiratory symptoms in a mildly hypothermic infant; otherwise, sepsis must be strongly considered.
A working classification of some of these disorders is presented in Box 10-1 . Whenever faced with these respiratory symptoms, the next steps (following a history and physical examination) should be to obtain the following:
- •
Chest x-ray
- •
White blood cell count with differential and hematocrit (Peripheral hematocrits can be higher than intravascular hematocrits.)
- •
Blood sugar
- •
Assessment of blood gas status via an arterial stick or capillary blood gas. A capillary blood gas reliably estimates Pa co 2 and pH, but not Pa o 2 .
- •
Pulse oximetry to assess oxygenation
Pulmonary disorders
Respiratory distress syndrome
Transient tachypnea
Meconium aspiration syndrome
Pneumonia
Air leak syndromes
Pulmonary hypoplasia
Systemic disorders
Hypothermia
Metabolic acidosis
Anemia/polycythemia
Hypoglycemia
Pulmonary hypertension
Congenital heart disease
Anatomic problems of the respiratory system
Upper airway obstruction
Airway malformations
Space-occupying lesions
Rib cage anomalies
Phrenic nerve injury
Neuromuscular disease
The decision to catheterize the umbilical artery (see Appendix D ) depends on the infant’s condition. The umbilical artery and/or vein may need to be catheterized if significant metabolic acidosis or blood loss is suspected or if the infant remains severely distressed (as defined by continued hypoxemia and severe respiratory distress). On the other hand, if the infant has tachypnea and grunting with retractions but is active and pink, it is possible to withhold catheterization unless there is deterioration as manifested by marked respiratory distress and an oxygen requirement exceeding 40% to 50%.
Although the newborn has a relatively larger cardiac output and a lower peripheral resistance and blood pressure than the older child and adult, measurements of blood pressure must be routine. It has been shown that hypotension in sick preterm infants need not be associated with hypovolemia. Hypothermia or acidemia results in severe peripheral vasoconstriction and will confound blood volume estimates from measurement of blood pressure. In a hypovolemic infant, blood pressure often declines only after acidemia and hypoxemia are corrected. Blood pressure can be measured with a blood pressure cuff of correct size placed on one or all extremities (if coarctation of the aorta is suspected), employing either oscillometric or Doppler ultrasound techniques. Alternatively, direct arterial measurements may be made via indwelling catheters. Normal blood pressures and ranges may be found in Appendix C .
If the initial hematocrit is less than 30% without blood incompatibility or if the blood pressure is reduced, it is reasonable to assume blood loss (e.g., an acute fetomaternal hemorrhage) and consider immediate correction of blood volume. With acute blood loss, hypotension prevails over anemia, whereas after chronic blood loss, anemia dominates the clinical picture and perfusion is less compromised. Saline is initially used and blood is requested, starting with a push infusion of 10 mL/kg, observing blood pressure, heart rate, and the infant’s general condition. One must be extremely careful with rapid infusions of any solution in the critically ill premature infant because of the risk of increasing the incidence of intraventricular hemorrhage (IVH) by rapid volume expansion. Once a diagnosis has been made, it is necessary to determine whether the neonatal unit has all of the facilities that might be needed during the course of the illness. The following section discusses RDS in great depth because this has been the primary model for understanding pathophysiology and management of neonatal respiratory disease.
Respiratory Distress Syndrome
RDS is still probably the most common initial problem in the intensive care nursery among premature infants, weighing less than 1500 g. However, in preterm infants whose mothers have received antenatal steroids, and after postnatal intratracheal surfactant therapy, the characteristic clinical course for RDS may not be apparent. The following lists give the common symptoms, the physiologic abnormalities, and the pathologic findings.
Signs and Symptoms
- •
Difficulty in initiating normal respiration. The disease should be anticipated if the infant is premature, the mother has diabetes, or if the infant has suffered perinatal asphyxia.
- •
Expiratory grunting or whining (caused by closure of the glottis), is a most important sign that sometimes may be the only early indication of disease; this maintains air in the immature lungs during expiration, and a decrease in grunting may be the first sign of improvement.
- •
Sternal and intercostal retractions (secondary to decreased lung and increased rib cage compliance)
- •
Nasal flaring
- •
Cyanosis (if supplemental O 2 is inadequate)
- •
Respirations—rapid (or slow when seriously ill)
- •
Extremities edematous—after several hours (altered vascular permeability)
- •
X-ray film showing reticulogranular, ground-glass appearance with air bronchograms
Physiologic Abnormalities
- •
Lung compliance reduced to as much as one fifth to one tenth of normal ( Fig. 10-3 )
Figure 10-3
Air pressure volume curves of normal and abnormal lung. Volume is expressed as milliliters of air per gram of lung. Lung of infant with respiratory distress syndrome (RDS) accepts smaller volume of air at all pressures. Note that deflation pressure volume curve closely follows inflation curve for the RDS lung.
- •
Large areas of lung not ventilated (right-to-left shunting of blood)
- •
Large areas of lung not perfused
- •
Decreased alveolar ventilation and increased work of breathing
- •
Reduced lung volume
These changes result in hypoxemia, often hypercarbia, and, if hypoxemia is severe, metabolic acidosis.
Pathologic Findings (Anatomic, Biophysical, Biochemical)
- •
Gross—the lung is collapsed, firm, dark red, and liver-like.
- •
Microscopic—alveolar collapse, with overdistention of the dilated alveolar ducts, pink-staining membrane on alveolar ducts (composed of products of the infant’s blood and destroyed alveolar cells); hence the earlier term hyaline membrane disease.
- •
Electron microscopic—damage and loss of alveolar epithelial cells (especially type II cells); disappearance of lamellar inclusion bodies.
- •
Biophysical and biochemical—deficient, or absent pulmonary surfactant, especially phospholipid (surface-tension lowering) component; abnormal pressure volume curve, as shown in Figure 10-3 .
Etiology
The distal respiratory epithelium responsible for gas exchange features two distinct cell types in the mature infant lung. Type I pneumocytes cover most of the alveolus, in close proximity to capillary endothelial cells. Type II cells have been identified in the human fetus as early as 22 weeks’ gestation, but become prominent at 34 to 36 weeks of gestation. These highly metabolically active cells contain the cytoplasmic lamellar bodies that are the source of pulmonary surfactant. Surfactant synthesis is a complex process that requires an abundance of precursor substrates, such as glucose, fatty acid, and choline, and a series of key enzymatic steps that are regulated by various hormones, including corticosteroids.
Phosphatidylcholine is the dominant surface tension-lowering component of surfactant. In addition, surfactant-specific proteins have been characterized and their functions partially elucidated. Of particular interest is surfactant protein B (SP-B), which is critical for minimizing surface tension and whose absence results in the phenotypic expression of lethal RDS at term. Following secretion from lamellar bodies within the type II alveolar cells, the key phospholipid and protein components of surfactant are conserved by recycling and subsequent regeneration of surfactant. Exogenously administered surfactant appears to contribute to this recycling program by increasing surfactant pool size without inhibiting endogenous surfactant production.
It is widely accepted that RDS is the result of a primary absence or deficiency of this highly surface-active alveolar lining layer (pulmonary surfactant). Surfactant, a complex lipoprotein rich in saturated phosphatidylcholine molecules, binds to the internal surface of the lung and markedly lessens the forces of surface tension at the air-water interphase, thereby reducing the pressure tending to collapse the alveolus. By equalizing the forces of surface tension in alveolar units of varying size, it is a potent anti-atelectasis factor and is essential for normal respiration. Alteration or absence of the pulmonary surfactant would lead to the sequence of events shown in Figure 10-4 . This results in decreased lung compliance (stiff lung) and thus an increase in the work of breathing. The additional work would soon tire the infant, leading to a sequence of reduced alveolar ventilation, atelectasis, and alveolar hypoperfusion.
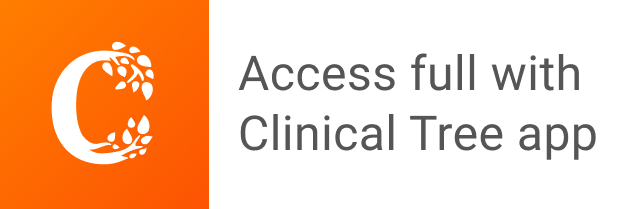