Principles of Management of Respiratory Problems
William E. Truog
Sergio G. Golombek
Although the principles of management of respiratory disorders are constant, the techniques of treatment have become more complex recently because of increasing and increasingly sophisticated technology. This complexity results from the incorporation into routine care of highly technical equipment and the necessity for skilled personnel—dedicated to the smooth functioning of life-sustaining devices. Knowledge of the pathophysiology of lung disorders and the maturational status of the lung is essential to applying the imperfect evidence derived from clinical trials and from experience to everyday use of assisted ventilation.
The goal of respiratory treatment is to provide tissue oxygenation and carbon dioxide (CO2) removal in a safe and effective manner. Excessive oxygen delivered to the airways or to organs can be harmful. Excessive lung distention can result in stretching and tearing of the lung. Excessive alveolar ventilation with resultant respiratory alkalosis and hypocarbia can in a detrimental manner alter blood flow distribution and oxygen unloading. In contrast, respiratory and/or metabolic acidosis may constrict pulmonary blood vessels, thus affecting pulmonary blood flow and oxygen uptake. Attention to organ system dysfunction other than the lung must occur in parallel with respiratory management or the efforts directed to optimizing delivery of assisted ventilation will be useless. One of the most challenging aspects of ventilatory treatment is the dynamic way in which the ventilatory needs can change because of either the treatment applied or the progression of the underlying disease. This chapter reviews the indications, methods, and complications of respiratory management and available methods of assessment.
OXYGEN THERAPY
Optimal Level Of Oxygenation
Considerable debate has occurred about what constitutes an acceptable level of oxygen tension or hemoglobin saturation, especially in extremely preterm infants (1). The debate has focused on the contribution of excessive or of insufficient supplemental oxygen levels and hence levels of arterial pO2 in the etiology of multiple disorders of prematurity. These disorders include central nervous system injuries, especially leukomalacia or hemorrhagic infarction; development or progression of retinopathy of prematurity (ROP); chronic lung disease of prematurity; and perhaps other disorders associated with excess level of reactive oxygen species (ROS).
Because of the direct relationship between elevated fraction of inspired oxygen (FiO2) and arterial oxygen partial pressure (PaO2) and the generation of ROS, it is reasonable that the arterial partial pressure of oxygen (PO2) and/or oxyhemoglobin saturation be minimized to a level sufficient to allow adequate oxygen tissue delivery with satisfactory reserves. There are several practical limitations to this seemingly innocent idea. Cardiopulmonary status of extremely preterm babies is inherently unstable. There is intrinsic cyclicity to such physiologic events as cardiac output and to spontaneous respiration rate and depth, and the variable contribution of spontaneously generated respirations in addition to those associated with assisted respiration.
Hemoglobin concentration is subject to significant changes and the relative quantity of the various forms of hemoglobin can change rapidly over the first days and weeks (e.g., decreasing fetal hemoglobin and increasing adult hemoglobin). As this occurs, the relationship between partial pressure of oxygen tension and oxygen saturation can change in somewhat unpredictable ways in short periods of time. Relying on only one measurement exclusively (e.g., PaO2 or pulse oximeter saturation [SpO2]) either may mask or may exaggerate changes in the tissue oxygen delivery. Hemoglobin concentration is measured per mL or 100 mL of blood, but the actual blood volume, and hence total body hemoglobin concentration, can vary in sometimes unpredictable ways also.
The overall limitation in assessing tissue gas exchange is that all the elements of tissue oxygen delivery are not routinely assessed at bedside. For example, arterial blood pressure measurements, either by sphygmometry or by direct arterial measurement, do not measure cardiac output or oxygen tissue delivery. Clinicians have available only summary information, at best, of total body oxygen delivery and consumption. In fact the body might be considered as interdependent organs with individualized oxygen consumption. Satisfactory levels of oxygen delivery in one or most of these may not be satisfactory for a particularly high-consuming area, and interorgan changes and distribution of blood flow can have a marked impact on local oxygen tissue delivery and consumption and hence on the risk of organ-specific ischemic injury.
For babies treated with assisted ventilation, it is possible to manipulate arterial oxygen tension, perhaps at the cost of other kinds of injury, especially to the lung. The tradeoff between mean airway pressure and FiO2 in the treatment of conditions associated with low end expiratory volume (e.g., respiratory distress syndrome or RDS) is one example of a difficult balancing act in clinical medicine. Central venous sampling or selective sampling of organ specific venous drainage would be crucial to optimizing the pressure FiO2-dichotomy, but obtaining that information is impractical.
Two randomized studies (2,3) and one case series (4) demonstrated that maintaining pulse Doppler oximetry levels in a higher end of normal range in a group of babies with progressive ROP did not result in reduced progression of ROP, but was associated with borderline statistically and clinically significant increases in pulmonary problems. However, Schultz and associates (5) demonstrated that with oxygen saturation and FiO2 maintained in a lower range of “normal,” there appeared to be an elevated pulmonary vascular resistance (PVR) and subsequent VA/Q mismatch (Fig. 31-1). Should episodes of lower SpO2 and higher PVR be exacerbated, then altered gas exchange with acute elevations in CO2 and subsequent ill effects on cerebral circulation could develop. The interdependency of these variables has meant that at present it is difficult to recommend, based on clinically relevant outcomes, a proper oxygen level.
![]() Figure 31-1 Relationship between venous admixture and PaO2. From Schulze A, Whyte RK, Way RC, Sinclair JC. Effect of the arterial oxygenation level on cardiac output, oxygen extraction, and oxygen consumption in low birth weight infants receiving mechanical ventilation. J Pediatr 1995;126 (5):777, with permission. |
An arterial PaO2 of 45 mm Hg results in a saturation of fetal hemoglobin (HbF) of approximately 90%. Maintaining the PaO2 above 50 mm Hg should be sufficient for tissue oxygen needs but excessive levels are quickly achieved. Mitochondrial PO2 is about 2 mm Hg.
The gap between the alveolar partial pressure of oxygen (PaO2) and the PaO2 indicates the magnitude of the arterial O2 gradient across the lungs and provides an indication of the magnitude of right-to-left shunting of blood. A simplification of the alveolar air equation provides an estimate of PaO2 i.e., PaO2 = PiO2-PaCO2.
Because at sea level the barometric pressure, minus water vapor pressure, is approximately 700 mm Hg, the percent of inspired oxygen multiplied by 7 equals PiO2 in mm Hg (e.g., 21% [approximate, equals] 147 mm Hg, 50% [approximate, equals] 350 mm Hg). Because PaCO2 approximates PaCO2 because of a usually small arterial-alveolar CO2 gradient (aADCO2), PaCO2 can be substituted for PACO2, and PAO2 can be derived. For example, if an infant is breathing gas with FIO2 of 0.6, with the measured PaO2 of 70 mm Hg and the PaCO2 of 40 mm Hg, the PAO2 = 420 – 40 = 380 mm Hg, and the alveolar-arterial gradient for O2 (AaDO2) approximates 310 mm Hg. In an infant without either lung disease or a significant right-to-left cardiac shunt, the AaDO2 should not exceed 25 mm Hg while breathing ambient air. Infants with severe RDS may have an AaDO2 in excess of 500 mm Hg while breathing 100% oxygen.
Oxygen Delivery
Each gram of HbF binds 1.37 dL of oxygen. The full-term newborn with a hemoglobin (Hb) of 17 g/dL binds and transports 23 dL of oxygen per 100 dL of blood. Less than 2% of transported O2 is carried as oxygen dissolved in plasma. Normal tissue oxygen consumption extracts 4 mL O2/100 mL if oxygen consumption and cardiac output are normal. HbF binds oxygen with a greater affinity than adult HbA. The oxyhemoglobin saturation curve is nonlinear, and the P50, the PaO2 at which Hb is 50% saturated, increases with gestational age (Fig. 31-2). The higher the P50, the greater the driving pressure for oxygen unloading. The curve gradually shifts to the right as hemoglobin A increases after birth. Several factors can adversely affect tissue oxygen delivery, including decreased cardiac output, maldistribution of cardiac output, arterial vasoconstriction, and shifts in the O2 dissociation curve. Oxygen unloading in the tissues is increased with a shift to the right of the O2 dissociation curve (i.e., decreased O2 affinity of Hb) facilitated by a local decrease in pH, increase in PaCO2, and increase in temperature. A shift to the right of the O2 dissociation curve can result from transfusion of adult red blood cells. Oxygen uptake depends on adequate alveolar ventilation (VA), an appropriate ventilation-perfusion match in the lungs, and absence of right-to-left shunting. Oxygen uptake or increased O2 affinity of Hb (associated with a shift of the curve to the left) is enhanced by alkalosis, decreased temperature, decreased 2,3-diphosphoglycerate, and increased HbF.
Oxygen Administration
There are two methods by which to deliver supplemental oxygen to neonates: an oxygen hood with sufficient gas flow to prevent CO2 retention and a nasal cannula or prongs. The concentration and the rate of flow are varied, and the precise amount of oxygen delivered to the lungs by nasal prongs is difficult to determine. This is because there may be dilution of inspired air through ill-fitting prongs or through an open mouth. Estimates of the effective FiO2 have been based on patient’s weight, gas flow rate, and concentration of oxygen blended in the blender (6). The O2-air mixture should be warmed to the same temperature as the incubator air, which should be in the range of thermal neutrality (see Chapter 24).
ASSESSMENT OF GAS EXCHANGE
Clinical Assessment
The infant with respiratory problems may present with a wide spectrum of clinical findings. The infant’s response depends on the degree of prematurity, lung and chest wall development, and maturation of respiratory control. The full-term infant may be able to increase the work of breathing to accomplish adequate gas exchange without treatment, including oxygen administration. The extremely premature infant will have a much weaker respiratory drive and inadequate muscular development and, thus, is less able to compensate for lung abnormalities. The classic clinical signs of respiratory distress are helpful in the assessment of the mature newborn infant. Nasal flaring, grunting respirations, and tachypnea are almost always present. With progression of lung disease and decreased lung compliance, chest wall retractions become more marked. With increased work of breathing, retractions progress from sternal to subcostal, to intercostal, and then to a seesaw pattern of chest and abdominal wall movement. The full-term infant may increase respiratory rate above 100 per minute, with shallow respirations. This pattern is the most efficient way to increase gas exchange, with the least costly work of breathing. Expiratory grunting represents an effort to retard expiratory flow to increase end-expiratory pressure and maintain alveolar patency. It is unsafe to rely on color changes as an indication of oxygenation, as abnormalities in peripheral perfusion as a result of poor cardiac output, hypotension, or hypovolemia may be misleading. Similarly, infants with recurrent apnea will have intermittent deficiency in gas exchange. Auscultation assists in determining the quality of air entry in various parts of the lung and the presence of airway secretions or obstructions.
Measurement and Techniques
The measurement of blood gases and pH helps to confirm clinical impressions, and verifies the values obtained by pulse oximetry, transcutaneous electrodes, and end-tidal CO2 monitoring. Most importantly, it helps to minimize the risks of hypoxia, hyperoxia, hypocapnia, hypercapnia, and metabolic acidosis.
Blood Gas Tension Measurements
Blood may be obtained by puncture of a peripheral artery, cannulation of a peripheral or umbilical artery, venous blood sampling, or capillary puncture. Venous and/or capillary blood values provide an approximation of arterial partial pressure of CO2 (PCO2) and pH but limited information about arterial oxygenation. The PCO2 in venous blood is approximately 6 mm Hg higher and the pH is 0.03 lower than in arterial blood.
Peripheral arterial blood most often is sampled from the radial or posterior tibial arteries using a 23- or 25-gauge needle. Peripheral puncture is indicated when the anticipated number of samples will be small, or if arterial cannulation is unsuccessful. The painful nature of the procedure may result in altered breathing and a spurious result.
Cannulation
The choice of peripheral artery or umbilical artery catheterization usually is determined by the size of the infant, and the anticipated duration of the cannulation. The major advantage of a peripheral arterial catheter is the avoidance of umbilical cannulation and risk of thrombosis. A right radial arterial catheter has the unique advantage of sampling preductal PaO2, which more accurately reflects retinal artery PO2.
The need to insert a catheter into the umbilical artery should be based on the infant’s maturation, postnatal age, and the type, severity, and expected duration of the illness. The high incidence of thrombus formation on the catheter tip, with its attendant risk of mural thrombus or emboli, must be weighed against the potential benefits to the infant. The tip of the umbilical catheter should rest in a low or high position to avoid the risk of occlusion, thrombosis, or direct infusion into a major branching artery. The distance to various levels within the aorta is estimated from the infant’s shoulder to umbilicus distance and the chart of Dunn (Fig. 31-3) (7). The high location range is below the ductus arteriosus and above the takeoff of the celiac artery, with the tip resting between T5 and T10. At the lower site, the tip should be above the bifurcation of the aorta (L3 to L5) and below the takeoff of the renal and inferior mesenteric arteries. There appears to be a higher complication rate with catheters in the low position. The higher position allows greater leeway for catheter migration, although there is a greater risk of downstream embolization. A thoracoabdominal radiograph should be obtained immediately after catheter placement and before medications are infused. Once the catheter is in place, there is a risk of intraluminal clotting. Therefore, a continuous infusion is required, as is flushing of the line following blood sampling. Heparinization of fluid infusions is performed in many special care nurseries routinely. The umbilical arterial catheter fluids serve as a site of low grade hemolysis. Amino acid containing fluids can reduce this risk and supply some necessary protein constituents (8). A pressure strain gauge should be connected to the catheter, with alarm limits to indicate changes in blood pressure. Dampening of the pressure waveform indicates intraluminal or catheter tip narrowing or obstruction. The catheter should be removed once the infant has improved, the frequency of blood gas determinations has decreased to once or twice per day, and systemic arterial pressure monitoring no longer is essential.
The complications of umbilical artery catheterization are listed in (Table 31-1). The most frequent problem is peripheral vasospasm associated with blanching or patchy cyanosis (See Color Plate) of the distal leg, foot, or toes. If this does not improve with reflex vasodilation by warming of the contralateral leg, or worsens over the next 15 to 20 minutes, the catheter should be removed. Some thrombosis at the catheter tip is inevitable. Fortunately, the rate of complications from thrombus formation is relatively low.
Transcutaneous PO2 and PCO2
Transcutaneous PO2 monitoring can be used selectively in conjunction with pulse oximetry. Most devices contain oxygen (O2) and CO2 electrodes. Noninvasive PO2 measurements continue to be helpful in certain situations, particularly if transcutaneous PCO2 also is obtained. Calibration before use and correlation with an arterial sample are necessary, but the need for subsequent blood samples should be reduced.
Arterial PO2 and transcutaneous PO2 are not identical. Differences can arise from local O2 consumption by the skin or by the electrode itself, heating of the skin, O2 diffusion time, and response time of the electrode (9). Skin blood flow may be affected by vasopressor medications, hypotension, and shock (10). Use of transcutaneous PO2 can reduce the number of blood sampling procedures, particularly during a period when rapid changes in O2 administration or mechanical ventilatory settings are taking place. Continuous monitoring for several hours also allows assessment of changes as a result of position, handling, suctioning, and feeding and for comparison with arterial oxygen saturation (SaO2) monitoring. The risk of partial thickness burns precludes its use for longer than 5 hours at a single site on the body.
TABLE 31-1 COMPLICATIONS OF UMBILICAL CATHETERIZATION | |||||||||||||||||||||
---|---|---|---|---|---|---|---|---|---|---|---|---|---|---|---|---|---|---|---|---|---|
|
Pulse Oximetry
Pulse oximetry provides a safe, accurate, and noninvasive adjunct to the assessment of tissue oxygenation 11,12). Oxygen saturation is determined by infrared spectrometry, utilizing two electrodes and a small cuff that can be placed around a hand, foot, or toe without requiring heating or calibration. One electrode contains two diodes that emit light at two wavelengths: red at 660 nm and infrared at 940 nm. The other electrode senses the light from both of these diodes that has not been absorbed by blood or tissue. The relative concentration of hemoglobin-oxygen (HbO2) and deoxyhemoglobin determines the amount of transmitted light, because different forms of Hb have markedly different absorption characteristics. The ratio of the amount of light absorbed at each wavelength is used to calculate a SaO2 value. The pulsed element of the apparatus allows the instrument to differentiate added arterial blood oxygenation and absorption from tissue, and it subtracts the amount contributed by nonpulsatile venous blood flow. With PO2 values greater than 40 mm Hg, the saturation accurately reflects measurements of PO2 obtained by catheter sample or by transcutaneous PO2 (12).
A PaO2 of 60 to 90 mm Hg results in a saturation value of 94% to 98% (see Fig. 31-2), and changes of 1% to 2% usually reflect a PaO2 change of 6 to 12 mm Hg. The point of inflection at which the HbO2 dissociation curve grows steep has considerable variability and depends on proportions of hemoglobin A, HbF, PCO2, pH, and temperature. Generally, these variables are not so critical to the interpretation of the percent SaO2 in arterial blood as they are to PaO2. Below 40 mm Hg, the SaO2 falls below 90%. Poor correlation with PaO2 exists when the SaO2 is above 96%, in which case the PaO2 may be well above 100 mm Hg. In very low birth weight (VLBW) infants who require chronic oxygen administration and who are at risk for developing ROP, the upper limit of saturation should be reduced to 95% or less depending on local nursery policies. Inaccuracies may reflect improper placement, movement, or peripheral ischemia. Motion artifacts also produce invalid readings. However, these problems are less significant with newer pulse-Doppler devices.
Near-Infrared Spectroscopy
Utilization of the unique light-absorbing properties of Hb and HbO2, as used in pulsed oximetry, has led to a sophisticated method of appraising tissue oxygenation by means of near-infrared spectroscopy. Near-infrared light penetrates the skin, bone, and various tissues and can be detected by electrodes placed on opposite sides of an infant’s skull. This permits assessment of cerebral tissue O2 and alterations in cerebral blood volume. Hb and cyto-chrome a and a3 (cyt a, a3) change their absorption characteristics according to the degree of oxygenation. The wavelength at which maximal absorption occurs is different for HbO2, deoxygenated Hb, total Hb, and reduced and oxygenated cyt a, a3. The small cranial size of the infant weighing less than 1500 g makes cross-temple spectroscopy feasible. However, the technique has not gained widespread use in clinical neonatology.
End-Tidal CO2 Monitoring
The concentration of CO2 at the mouth or nose rises to reach a plateau at the end of each breath. This plateau reflects the alveolar CO2 concentration under normal conditions, but may be inaccurate if there is marked ventilation-perfusion inequality or inhomogeneity of lung disease. Recent refinements to end-tidal CO2 detection equipment permit in-line or “mainstream” infrared monitoring just proximal to the endotracheal tube (capnography), with a continuous display of the PCO2 waveform. There is minimal dead space of the apparatus, and sampling accuracy has improved to compensate for the low expiratory flow rates characteristic of small premature infants. End-tidal CO2 is less reliable in extremely small premature infants. Capnography is as accurate as capillary PCO2 but is less precise than transcutaneous monitoring (1314,15).
Hazards of High or Low Arterial CO2
Identifying a safe range of PCO2 has proven as difficult as for PaO2. There are substantial risks to hypocarbia (Fig. 31-4) (16) (Table 31-2). Limiting the exposure time to hypocarbia may be important in presenting the development of cystic periventricular leukomalacia or decreasing the risk of CLD (17,18 and 19). There has been an increase in use of permissive hypercarbia, by which PaCO2 levels of 50 to 60 mm Hg are sought and maintained. The rationale is to avoid high peak inspiratory pressure or to delay the onset of or to avoid assisted ventilation (20,21).
![]() Figure 31-4 Effects of Hypocapnia on the Brain in Premature Infants. Hypocapnia has been implicated in the pathogenesis of neonatal white-matter injuries, including periventricular leukomalacia, resulting in intraventricular hemorrhage. At normal carbon dioxide levels (left-hand side of figure), cerebral blood flow is determined by local metabolic demand. Prolonged or severe hypocapnia includes severe cerebral vasoconstriction, resulting in brain ischemia, particularly in poorly perfused areas of the brain such as watershed areas (right-hand side of figure). This ischemia may initiate white-matter destruction in the brain of premature infants. Additionally, antioxidant depletion (caused by excitatory amino acids), lipopolysaccharide (LPS), and cytokines produced in response to sepsis, such as interleukin-1β and tumor necrosis factor α (TNF-α), potentiate the process. Finally, restoration of the normal partial pressure of arterial carbon dioxide can result in cerebral vasodilation, which may precipitate or contribute to intraventricular hemorrhage. From Laffey JG, Kavanagh BP. Hypocapnia. N Engl J Med 2002;347 (1):43, with permission. |
STRATEGIES FOR RESPIRATORY SUPPORT
Continuous Positive Airway Pressure
The application of end-expiratory pressure is intended to prevent alveoli and/or terminal airways from collapsing to airlessness. Continuous positive airway pressure (CPAP) may be applied during spontaneous breathing or as positive end-expiratory pressure (PEEP) during mechanical ventilation. This usually requires pressures between 4 to 6 cm H2O for CPAP and 3 to 8 cm H2O for PEEP.
TABLE 31-2 RISKS OF HYPOCARBIA AND HYPERCARBIA | |
---|---|
|
The physiologic effects of CPAP/PEEP may vary depending on the underlying pulmonary pathology, although the primary goal is to prevent alveolar collapse. Grunting respirations in infants with respiratory distress suggest laryngeal narrowing and increased resistance to expiratory flow to increase end-expiratory alveolar pressure. In the surfactant-deficient state, alveoli will collapse at end-expiration unless a minimum distending pressure is maintained. CPAP of 3 to 4 cm H2O will prevent alveolar collapse but will not recruit atelectatic alveoli. Opening pressures of 12 to 15 cm H2O are required to inflate collapsed alveoli. The infant will need to create a large distending airway pressure in the absence of CPAP. The shear forces from opening and closing of small airways may contribute to alveolar epithelial damage. Additionally, resultant abnormal distending forces on terminal or respiratory bronchioles will contribute to small airway injury. CPAP above physiologic levels of 3 to 4 cm H2O may cause overinflation of some alveoli. Therefore, inflation and deflation may occur on the flatter portion of the pressure-volume curve and increase the work of breathing. CPAP theoretically could stimulate surfactant secretion. Maintenance of alveolar volume will reduce right-to-left shunting of blood through atelectatic alveoli, hence reducing oxygen needs.
Indications
The clinical indications for CPAP are varied. Initial use was directed at infants with RDS with the goal of avoiding or at least delaying intubation and mechanical ventilation. The gestational age, birth weight, and stage and severity of respiratory disease should be factored into the decision to initiate CPAP. If infants have severe lung disease, e.g., meconium aspiration, severe respiratory distress syndrome with FiO2 needs <6, or idiopathic pulmonary hypertension, more appropriate therapy would include assisted ventilation.
Infants <1,000 g birth weight are at considerable risk for developing chronic lung disease (CLD) and recurrent apneic episodes; therefore, they commonly are intubated and supported with mechanical ventilation as a result of their inability to sustain an adequate respiratory effort. They often develop an increasing oxygen need during the second week of life, associated with radiographic signs of CLD and attributed, in part, to assisted ventilation. CPAP may be beneficial in maintaining patency of extremely small terminal airways and prealveolar gas exchange units in these very immature infants. CPAP appears to be well tolerated by such infants over a period of many days. The increased enthusiasm for the use of CPAP is stimulated by the desire to minimize or prevent CLD. Because assisted ventilation is one putative factor in the etiology of CLD, the use of CPAP shortly after birth to avoid or minimize barotrauma has much appeal. Avoidance of endotracheal intubation should decrease the chances for tracheal injury, airway infection, abnormal mucociliary function, and over- inflation from excess ventilator pressure or volume.
Brief intubation and the administration of a single dose of surfactant followed by nasal CPAP has been advocated as another method of reducing the need for mechanical ventilation in infants with moderate RDS (22). Improve-ment in gas exchange has been demonstrated; however, more evidence of its efficacy in reducing the incidence or severity of CLD is required.
Recurrent Apnea
CPAP helps some infants with recurrent apnea of prematurity to sustain a more regular respiratory rate. The mechanism of its action is not well understood, although an increase in functional residual capacity (FRC) may alter the Hering-Breuer reflex or stabilize the thoracic cage, minimizing chest wall distortion and possibly altering inhibitory spinal cord reflexes (23). CPAP also helps to overcome obstructive apnea and decreases total respiratory system resistance (24,25).
Earlier methods of applying CPAP utilized an enclosed head box, face masks, and nasopharyngeal tubes. More recently, nasal prongs have been adapted to fit most infants. One device (the ALADDIN Infant Flow System, Hamilton Medical Inc., Reno, NV) appears to be well tolerated by both large and small infants. This apparatus maintains a constant flow of air by incorporating a double fluidic jet system within the apparatus. During inspiration, one jet maintains the flow to match the infant’s inspiratory effort; during expiration, gas flow is reversed by a second jet to assist outflow while maintaining a constant minimum pressure. This system presumably does not add to the work of breathing and reduces the need to use high flow rates to compensate for air leak around the nasal prongs. A second system utilizes a variable depth water seal for the expiratory circuit to sustain continuous airway pressure (26). CPAP can also be applied utilizing constant flow ventilators.
Complications
CPAP may have adverse effects. Overinflation can result in increased work of breathing and decreased efficiency of gas exchange. Pneumothorax and pneumomediastinum may result from lung overdistension. Carbon dioxide retention may occur as a result of either increased dead space or ineffective ventilation of some alveoli. If the mean thoracic pressure is elevated with high levels of PEEP or CPAP, e.g., above 7 to 8 cm H20 in the absence of lung disease, cardiac output may be decreased because of impaired systemic and pulmonary venous return. The nasal prongs may cause irritation if the fit is not appropriate or the infant is active. Gastric distention may occur, making gastric feedings difficult, and often an indwelling or gastric tube is required for decompression.
Effectiveness
Does the use of CPAP decrease the need for assisted ventilation and does it prevent or ameliorate CLD in VLBW infants? Clinical trials conducted before the era of routine surfactant use and contemporary neonatal ventilation devices are now of limited relevance. Recent studies comparing the efficacy of conventional mechanical ventilation (CMV) to CPAP following the administration of surfactant found some differences in need for ventilation as an outcome although the number of infants studied was small (22,28).
CPAP is used to facilitate weaning from mechanical ventilation. Some infants, experiencing recurrent apneic episodes, appear to benefit (29), whereas infants evaluated in other studies have shown no benefit (30). Additional information is needed to confirm whether CPAP is an effective adjunct to successful extubation. The technique of nasal intermittent positive pressure ventilation (NIPPV) may gain widespread use if it can be shown to improve long-term outcome (31).
Assisted Mechanical Ventilation
Treatment with assisted ventilation is applied commonly to newborns across the neonatal birth weight spectrum (Fig. 31-5) (32). Illustrated is the rate of use of assisted ventilation, adjusted by birth weight, and the percent of all infants treated for more than or equal to 24 hours. Thirty-eight percent of treated infants were over 2,500 g birth weight in this survey, covering all California and New York State births for 1994-1995. Neonatologists must be expert in providing assisted ventilation across a 10-fold range of patient weight (0.5 kg-5.0 kg) and providing safe and effective assisted ventilation across a range of lung development from premature airways with preacinar gas exchange spaces to a virtually completely alveolarized organ. Thus, both size (patient and lung volume) and range of development are immense.
The Immature Lung
The immature lung presents a special hazard for the application of assisted ventilation. The application of positive pressure for the purpose of increasing ventilation and optimizing ventilation-perfusion (V/Q) matching may injure epithelial and endothelial tissues. In the incompletely developed lungs, structures are less elastic and more vulnerable to barotrauma. Injury to the mesenchymal and epithelial tissues that later give rise to alveolar septation and vascular formation may be irreversible. Studies in adult animals have demonstrated that otherwise healthy lungs can suffer injury, which is reflected by increased airway fluid and deterioration of gas exchange, if inappropriate distending lung pressures are applied (33). The particular problems of providing assisted ventilation are illustrated in Fig. 31-6 (34). Relative immaturity of distal bronchioles and respiratory ducts, coupled with fluid-filled and collapsed alveoli, create a set of conditions leading to overdistention of some areas and underventilation of other areas, with resultant ineffective gas exchange. This uneven ventilation, coupled with injury produced by reactive oxygen species, contributes to the common problem of chronic lung disease of prematurity. The risk of its development is inversely correlated with birth weight.
Great strides have been made in understanding how the immature lung differs from a mature lung in phospholipid and surfactant-associated protein biosynthesis. However, there are factors other than surfactant biosynthesis that are unique to the immature lung and that increase the susceptibility to injury. These factors include, but are not limited to, incomplete development of the supportive net of collagen and elastin (35,36), incomplete development of the capillary bed in the gas exchange areas (37), relative instability of the chest wall with reduced capacity to maintain expiratory lung volume at FRC, immaturity of the neural control producing sustained spontaneous respiratory effort, and probable immaturity of the metabolic functions of the pulmonary endothelium.
Respiratory failure ensues when spontaneous breathing efforts fail to produce adequate alveolar ventilation. In newborn infants, this may occur because of failure of adequate output from central nervous system respiratory centers, an overly compliant chest wall that increases the work of breathing, metabolic problems as a result of limited energy stores, or profoundly noncompliant lungs requiring more work and depleting available energy stores. Each of these may be an indication for assisted ventilation. In most neonatal respiratory disorders, these problems occur in combination, and the diagnosis of respiratory failure cannot be ascribed to any single cause.
Establishment of an Artificial Airway
PHYSIOLOGIC AND ANATOMIC AIRWAY PECULIARITIES
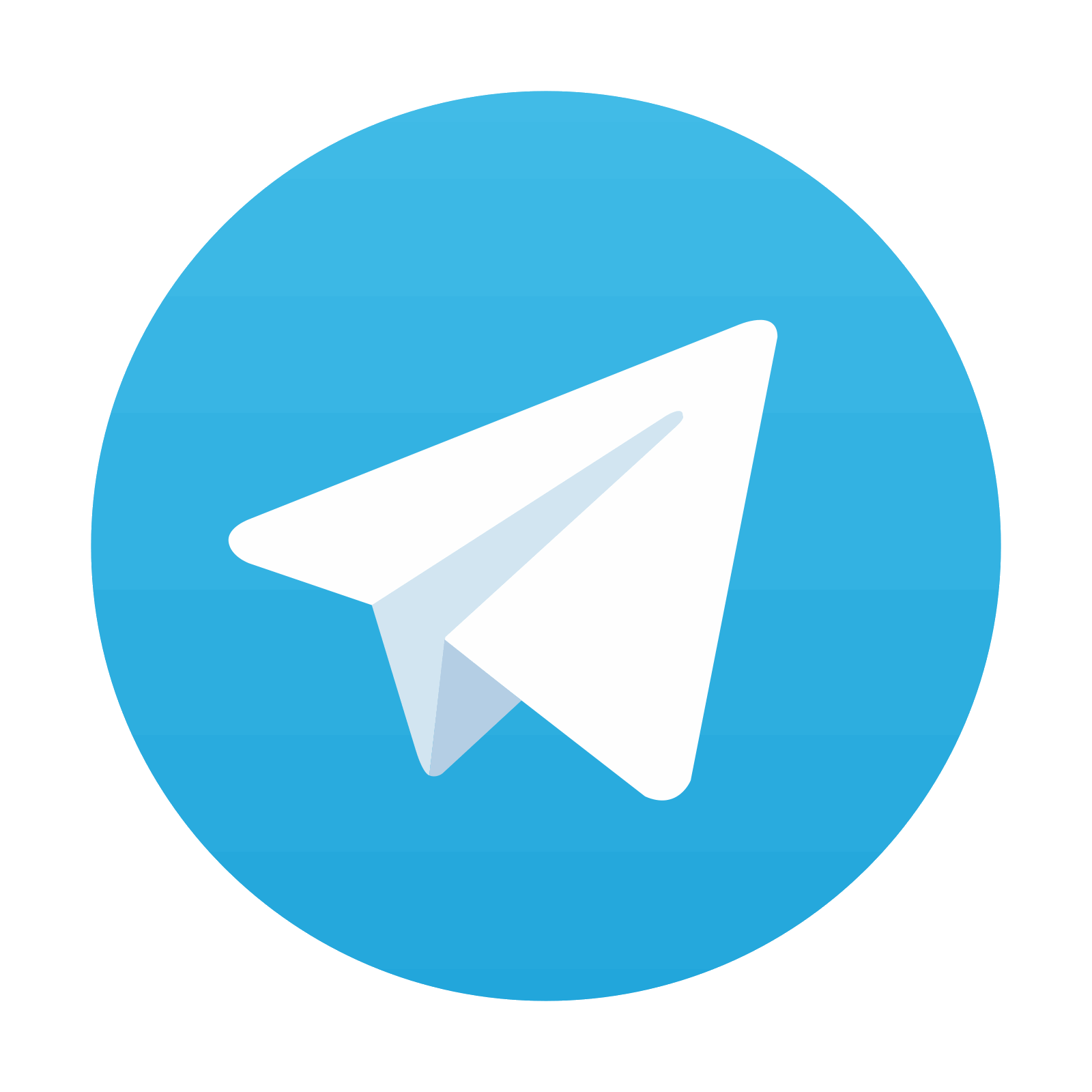
Stay updated, free articles. Join our Telegram channel
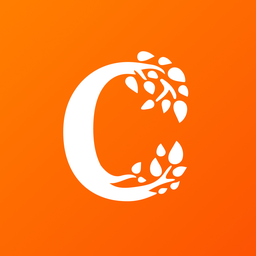
Full access? Get Clinical Tree
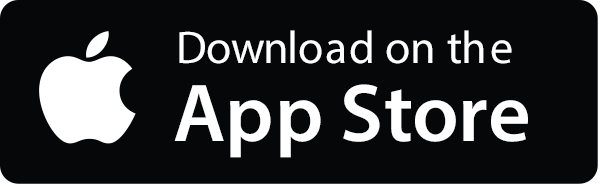
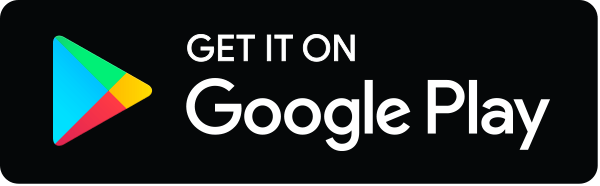