Pharmacokinetics is the study of drug movement through the body, and describes the absorption, distribution, metabolism, and excretion of a substance (1,2). Pharmacokinetics determines how a specific dose of a drug will eventually reach target tissues to exert a clinical response (pharmacodynamics). Both patient- and drug-related factors may influence dosage adjustment in individual patients (Table 45.1). Physiologic processes and certain diseases may modify specific pharmacokinetic parameters, such as volume of distribution and clearance. Although patient-related factors are responsible for most of the variability in the relationship between drug doses and serum concentrations, differences in drug formulation can substantially influence the time course of drug action. The physical properties of a drug (ie, molecular size, ionization constant [pKa], lipid and aqueous solubility, dissolution rate) determine how the drug will interact with physiologic processes in the body to achieve necessary concentrations at target sites of drug action in the nervous system (3). A basic understanding of principles and factors that influence a drug’s pharmacokinetic profile can improve patient care by optimizing anticonvulsant dosing and monitoring, enhancing efficacy, and in some cases ameliorating dose-dependent adverse effects. This chapter reviews fundamental pharmacokinetic concepts, pharmacokinetics of antiepileptic drugs (AEDs), and the factors that can affect application of pharmacokinetics in the clinical setting. It also provides a brief overview of pharmacogenomics and its potential application to care of patients with epilepsy.
FUNDAMENTAL PHARMACOKINETIC CONCEPTS
Pharmacokinetic Models
Pharmacokinetic principles are based on mathematical models that are useful in predicting the time course to achieve drug concentration in fluids and tissues (2). When a model is selected for pharmacokinetic analysis of a specific drug, assumptions must be made to simplify complex physiologic processes. Models are named using the number of compartments (eg, one-compartment, two-compartment, or multicompartmental) and according to the type of pharmacokinetics that are applied (ie, linear versus nonlinear). Compartments do not represent a specific tissue or fluid, but represent a group of similar tissues or fluids. For example, highly perfused organs (eg, lungs, heart, liver, kidneys) often have similar distribution patterns, and along with blood/plasma are referred to as the central compartment. The peripheral compartment is less well perfused and includes fat tissue, muscle, and cerebrospinal fluid (CSF).
Although two-compartment models are preferable to one-compartment models, one-compartment models have been used most frequently in clinical practice and in pharmacokinetic studies. The one-compartment model assumes that the body is a single compartment and that all tissues and fluids belong to that compartment (2). This model also assumes that a drug distributes instantaneously to all body areas following administration of a single dose. Drugs that do not fit this model generally follow a two-compartment model, which assumes that a drug distributes into the central (vascular) and peripheral (tissue) compartments at different rates. For example, a drug may rapidly distribute into the bloodstream and to highly perfused organs and then distribute more slowly into other body tissues. Eventually the drug achieves equilibrium between the compartments by moving back and forth (Figure 45.1). Older literature suggested that phenobarbital’s biphasic distribution was responsible for a slower onset of action; however, more recent information has shown that phenobarbital distributes rapidly (within minutes) after intravenous administration to highly perfused organs, including the brain.
TABLE 45.1
FACTORS THAT DETERMINE THE PHARMACOKINETICS OF A DRUG | |
Drug-Related Factors |
Patient-Related Factors |
Molecular weight Ionization constant Lipid solubility Aqueous solubility Formulation |
Pharmacogenetics Age Diet and nutritional state Disease Comedication Route of administration |
Volume of Distribution
Volume of distribution (Vd) measures the apparent space in the body available to contain the drug and describes the extent to which a drug transfers into various parts of the body (1, 2). It relates the amount of drug in the body to the concentration of drug (Cp) in the blood or plasma. Vd refers to a hypothetical volume for which a given drug distributes (usually reported in milligrams per liter). Because it is the apparent volume necessary to contain the amount of drug at the concentration found in the serum or plasma, the values can exceed any physical volume in the body. Stated another way, it relates the amount of dose (D) of drug that would be required to produce a given plasma concentration at the end of the dose (Cp) and is defined by the following equation:
Vd = FD/Cp (F = 1 for IV administration)
When Vd is reported, it is typically normalized by weight (eg, liters per kilogram) or body surface area (liters per square meter).
The extent of a drug’s distribution is determined by its lipid–water solubility and affinity for plasma proteins (eg, albumin or α1-acid glycoprotein) or tissues. The term Vd is used to characterize or quantify these properties. Drugs with smaller Vds tend to be more protein bound or hydrophobic. Drugs with a large Vd exhibit low protein binding or have a higher degree of lipophilicity, which results in higher concentrations in extravascular tissue than in the vascular compartment. Many factors can influence Vd (eg, age, gender, pregnancy, renal or hepatic failure, changes in protein binding). Alterations in protein binding may result in changes in drug distribution and are particularly important for drugs with binding of 90% or higher (eg, phenytoin, valproate). Valproate’s protein binding is unique in that it is saturable at therapeutic concentrations. This results in nonlinear increases in free (active) valproate concentrations compared to the total concentration as drug doses are increased.
FIGURE 45.1 One-compartment and two-compartment models for drug distribution.
Another important concept for the clinical application of Vd relates to its use in determining a loading dose. A loading dose quickly increases a patient’s plasma concentrations to a desired amount. If a patient’s Vd is unknown, it is assumed to fall within the population normal for a specific drug. For example, the population normal Vd of phenobarbital (Table 45.2) is 1 L/kg. If a patient has not received any phenobarbital and one desires an immediate concentration of 20 mg/L, the patient should be given 20 mg/kg (dose = Cpdesired/Vd). Once a postloading peak serum concentration is obtained, an individual’s Vd can be calculated and used in subsequent dosing. For example, if the patient was given 20 mg/kg of phenobarbital and had a resulting postloading dose concentration of 22 mg/L, the patient’s apparent Vd would be 0.9 L/kg. If one desires to further increase the serum concentration to 30 mg/L, this could be done using the following equation:
Dose = (Cpdesired – Cpachieved)/Vd
For this example, the resulting dose would be 8.8 mg/kg [(30 − 22 mg/L)/0.9 L/kg]. By examining the equation, one can see that the plasma concentration of a drug is independent of its metabolism or elimination and only depends on the dose given, its bioavalibility, and the drug’s Vd. Stated another way, things that alter drug clearance (eg, induction or inhibition) do not affect a patient’s loading dose requirement.
Clearance
Clearance (Cl) summarizes all of the factors that act to reduce the drug concentration and is the measure of the body’s ability to eliminate the drug (1,2). Clearance is defined as the volume of distribution that is completely rid of drug per unit of time; hence, it has the dimensions of volume per time. The relative clearance is the best parameter for comparing drug dose requirements among different groups of patients. It also provides the most reliable basis for determining an initial maintenance dose, because clearance accommodates variations in body water and fat content. Unless a patient is receiving a continuous infusion of drug, clearance is rarely used to adjust dose, because the population normal values are more difficult to remember than doses based on weight. Operationally, clearance is the product of the Vd times the elimination rate constant.
Cl = Vd × Ke
Elimination Rate Constant
The elimination rate constant (Ke) is the fraction of drug that is eliminated from the body per unit of time. In first-order kinetics, it is independent of drug concentration in the body. It is easiest to evaluate drug elimination after intravenous dosing, when drug absorption is not an issue. With first-order elimination, the amount of drug eliminated is directly proportional to the serum drug concentration. A log scale plot of these data on the y-axis yields a straight line. The slope of this straight line correlates to Ke (Figure 45.2A). Mathematically, this relationship may be represented by the following equation:
Ke = [ln(Cp1/Cp2) / T2 − T1]
In a two-compartment model, the elimination rate constant is more complex, as drug distributes into the central and peripheral compartments at different rates. At first, the drug concentration declines very rapidly, but later the rate of decline becomes slower (Figure 45.2B). The initial rapid decline (the greek symbol phase = A) is due to instantaneous distribution of the drug from the central (vascular) compartment into peripheral (tissue) compartments. The the greek symbol phase = B, also referred to as the postdistribution or terminal phase, depicts a slower decline that is determined predominantly by drug elimination. The terminal portion of the concentration–time curve (the greek symbol phase = B) is used to determine the elimination rate constant. For a two-compartment model, the concentration–time curve after an intravenous dose is described by the following equation:
Cpt = A · e–αt + B · e–βt
Half-Life
A drug’s half-life (t1/2) is determined by the rate of metabolism and excretion of the drug and is defined as the time required for the amount of drug in the body (ie, serum concentration) to decline by 50%. Like the elimination rate constant, this too is calculated from the terminal portion of the concentration–time. While the elimination rate constant has the dimensions of a fraction per unit time, half-life has the dimension of time only. Half-life is described by the following equation:
t1/2 = 0.693/Ke
Both Ke and t1/2 are dependent upon clearance and volume of distribution (Cl = Vd × Ke). Clearance is inversely related to half-life. A long half-life reflects a low clearance [Cl = Vd (0.693/t1/2)]. The relative clearance is the clearance divided by weight.
TABLE 45.2
FIGURE 45.2 Semilogarithmic plot of intravenous concentration versus time curve based for a one-compartment model (A) and a two-compartment model (B). Curve A is obtained by subtracting the extrapolated terminal portions of curve A+B.
Half-life of a drug is important for several reasons. First, it indicates how much time is required for drug concentrations to stabilize after the dose is changed. Steady state refers to that time when drug input equals the rate of drug output. Assessments of serum concentrations before steady state can underestimate or overestimate the overall efficacy of a dose and cause one to make an unnecessary dose adjustment. Second, under steady-state conditions, the drug concentration is directly proportional to the half-life. The half-lives of curves A, B, and C are 4, 8, and 12 hours, respectively (Figure 45.3). If the half-life increases, the time to reach steady state is delayed and the resulting serum concentrations are higher. Third, the half-life ordinarily dictates how frequently doses should be administered. In most cases, doses should be administered at intervals equal to or less than the half-life, to limit the fluctuation in drug serum concentration between doses. An AED with a half-life of 1 to 8 hours usually requires dose administration every 1 to 3 half-lives. An AED with a half-life greater than 8 to 24 hours typically is given every half-life, and those with half-life greater than 24 hours can be given once daily for convenience. This principle explains why a medication like carbamazepine (immediate-release tablet or liquid) must be given several times per day, whereas phenobarbital can be given once daily. For drugs that have a short half-life, delaying absorption by the administration of slow-release formulations (Table 45.3) also limits the fluctuation in serum concentrations between doses.
FIGURE 45.3 Effect of varying half-life on time to reach steady state and on the eventual concentration at steady state. The half-lives of curves A, B, and C are 4, 8, and 12 hours, respectively. Increasing the half-life delays steady state and results in higher concentrations.
Steady State
In order to obtain clinically useful information, blood samples for serum drug concentrations are generally collected at steady state. When drug administration is initiated at a constant or fixed dose rate, the drug progressively accumulates in the body until the clearance equals the dosing rate (ie, steady state). For a first-order, one-compartmental drug for which the dose is unchanged, five half-lives must elapse before steady state is reached and drug concentration stabilizes (1,2). The time to reach steady state is independent of the dose, the number of doses administered, and the dosing interval, but it is directly proportional to the half-life. For drugs that exhibit nonlinear, or extensive first-pass, metabolism in the liver, the time to steady state may differ from the conventional five half-lives.
Administration of a loading dose causes drug serum concentration to increase rapidly and may enable one to achieve a target serum concentration more quickly. That said, a loading dose does not necessarily produce the same concentration that will eventually occur at steady state. Steady-state concentrations depend on the administration of a maintenance dose, which must be administered repeatedly (Figure 45.4). In the figure, note that although the same loading dose was given, the half-life for curve D is 8 hours, and that for curve E is 20 hours.
Under steady-state conditions, the average drug concentration in serum (Cpss) is constant and is equal to the dosing rate divided by the clearance:
After clearance is replaced with Vd and t1/2, it can be seen that the average drug concentration at steady state is directly related to the dosing rate and half-life and inversely related to the volume of distribution:
TABLE 45.3
EXTENDED-RELEASE FORMULATIONS* | ||
Product |
Dosing Interval |
Formulation |
Phenytoin sodium (Dilantin) |
qd |
30, 100 mg capsule |
Carbamazepine ER (Tegretol XR) |
bid |
100, 200, 400 mg tablet |
Carbamazepine ER (Carbatrol) |
bid |
100, 200, 300 mg capsule |
Divalproex sodium ER (Depakote ER) |
qd |
250, 500 mg tablet |
Gabapentin Enacarbil ER (Horizant ER and Gralise) |
qd |
300, 600 mg tablet |
Lamotrigine ER (Lamictal XR) |
qd |
25, 50, 100, 200, 250, 300 mg tablet |
Topiramate ER capsule | ||
(Trokendi XR) |
qd |
25, 50, 100, 200 mg capsule |
(Qudexy XR) |
qd |
25, 50, 100, 150, 200 mg capsule |
Levetiracetam ER (Keppra XR) |
qd |
500, 750 mg tablet |
Oxcarbazepine ER (Oxtellar XR) |
qd |
150, 300, 600 mg tablet |
*Available in the United States as of July, 2015.
Linear and Nonlinear Pharmacokinetics
Linear Pharmacokinetics
Most AEDs exhibit linear, first-order pharmacokinetics, which means the dose and resulting total and free serum concentrations increase in direct proportion to one another (Figure 45.5). When a drug exhibits linear pharmacokinetics, a constant fraction is absorbed, transported, and eliminated per unit of time. These elimination mechanisms dispose of a constant fractional amount of the drug independent of the drug concentration and are capable of removing much more drug than is usually present. For example, if the dose rate is increased or decreased twofold, the drug serum concentration will also increase or decrease twofold. Because the relationship between the drug dose and serum concentration is linear, it is intuitively predictable; hence, a dose can be individualized by simply cross-multiplying and solving for the new dose. For example, if the phenobarbital concentration is 20 mg/L on a dose of 300 mg/day and the desired steady-state concentration is 30 mg/L, one would give 450 mg/day.
FIGURE 45.4 Effect of varying half-life after loading doses. Note that independent of the administration of a loading dose, five half-lives are required for levels to stabilize. The half-life for curve D is 8 hrs, and that for curve E is 20 hours.
FIGURE 45.5 Dose versus serum concentration for drugs with linear (A) versus nonlinear (B) elimination.
Nonlinear Pharmacokinetics
Some drugs do not follow a linear model, but rather display nonlinear pharmacokinetic patterns that are saturable, concentration dependent, or dose dependent (Figure 45.5). Dosage adjustment for these drugs should be approached cautiously, as small changes in dose may result in disproportionately large changes in the serum concentration.
When a drug is eliminated by processes that employ nonlinear pharmacokinetics, the fractional rate of drug disposition varies with the dose or drug concentration. As a drug concentration increases, elimination becomes progressively saturated, resulting in a prolonged half-life. In this situation, the half-life that is observed should be designated as t50% to differentiate it from the usual t1/2.
The apparent half-life is directly proportional to the initial concentration that is present when the half-life is measured (4). Thus, increasing the dose leads to disproportionate increases in the serum concentration. When drug doses are reduced, the opposite occurs; small reductions in dose can cause precipitous declines in drug serum concentration. Finally, the amount of drug in the body and the concentration of the drug in plasma progressively increase if the dosing rate exceeds the capacity for drug elimination.
While most AEDs exhibit linear pharmacokinetics, gabapentin, carbamazepine, phenytoin, and valproate exhibit various forms of nonlinear pharmacokinetics (Figure 45.6). Gabapentin absorption is regulated by the L-amino acid transporter system located in the proximal small bowel. Because this system exhibits saturable capacity limited activity, gabapentin undergoes dose-dependent absorption (5,6). As the dose of gabapentin is increased, the bioavailability disproportionately decreases. The carrier-mediated mechanism that transports gabapentin across the blood–brain barrier is likely saturable at higher plasma concentrations (7). Carbamazepine (α1-acid glycoprotein), phenytoin (albumin), and valproate (albumin) exhibit saturable protein binding at plasma concentrations within their therapeutic serum concentration ranges; therefore, as the serum concentration increases, the percent of unbound drug disproportionately increases.
Carbamazepine also induces its own metabolism (ie, autoinduction or time-dependent metabolism) in a concentration- and time-dependent manner and affects concentrations of both carbamazepine and the carbamazepine-10,11-epoxide (8). Autoinduction occurs during the first month of therapy, often at the point of dose titration (9). Because dosage increases may not result in linear increases in carbamazepine serum concentrations, low concentrations may be misconstrued as medication nonadherence. The elimination mechanisms for phenytoin have a low capacity relative to the phenytoin serum concentrations that are usually present, so these mechanisms are partially saturated (4,10). This nonlinear elimination causes the relationship between the phenytoin dose and serum concentration to be extremely unpredictable. As the dose is increased, clearance decreases, resulting in a disproportionate increase in serum concentration (Figure 45.6A).
Michaelis–Menten Equations
The Michaelis–Menten equations describe the kinetics of enzyme reactions and can be used to define nonlinear drug elimination (1). This is done by measuring two or more steady-state drug concentrations and then solving for the nonlinear pharmacokinetic parameters: the maximal reaction velocity (Vmax) and the drug concentration at which the reaction rate is one-half of the maximal elimination velocity (KM). The critical assumption that underlies these calculations is that at steady state the rate of drug dosing equals the rate of drug elimination; hence, at steady state the dosing rate equals the reaction velocity. Similarly, the drug concentration is analogous to a substrate concentration. In order to calculate the nonlinear kinetic parameters, Vmax and KM, it is necessary to know two or more pairs of doses and concentrations. Several methods have been described for calculating Vmax and KM (11). These are discussed further in Chapter 60.
FIGURE 45.6 Example of nonlinear and linear characteristics on serum drug concentration.
For drugs with nonlinear kinetics, varying the absorption rate changes the apparent bioavailability. Martis and Levy described the effects of bioavailability (F), absorption rate (ka), KM, and Vmax on the rate of phenytoin concentration change (12).
In this equation, ka is the first-order absorption rate; Cp0 is the drug concentration at time zero; Cp is the concentration at any time t; F is the bioavailability or fraction of the drug that is ultimately absorbed; and K1 is the overall apparent first-order rate constant. This equation has been used to evaluate the relationship between absorption rate and apparent bioavailability. Based on computer simulations using average values of KM and Vmax, the apparent bioavailability declines by 25% as the time for absorption increases from 0 (intravenous administration) to 1.2 hours (13).
DRUG ABSORPTION
AEDs are given by the oral, intravenous, intramuscular (IM), subcutaneous, intranasal, rectal, or percutaneous routes. Regardless of administration technique, drugs given by an extravascular route must overcome chemical, physical, and biological barriers in order to be absorbed.
Absorption describes the entrance of a drug into the intravascular space, typically from the gastrointestinal (GI) tract, skin, or muscle (Figure 45.7). Drug absorption, or bioavailability, is defined in terms of both rate and extent. The rate of absorption is described by the absorption rate constant (ka, units 1/unit time) or the time to maximal plasma concentration (Tmax). The rate of absorption determines when the peak serum concentration will occur and is associated with the drug’s onset of action. Slowing the rate of absorption will attenuate the range of fluctuations in drug concentrations between doses by postponing the Tmax and reducing the peak serum concentration (Cpmax).
FIGURE 45.7 Various mechanisms of absorption, distribution, elimination, and metabolism.
The absorptive phase is minimal for intravenously administered AEDs; the Tmax is rapid and coincides with the end of the bolus. Typically the rate of absorption is slower for IM, rectal, sustained-release capsules/tablets, and subcutaneous administrations than for immediate-release oral capsules/tablets and solutions. For most currently available AEDs, the ka is a first-order process, meaning that a constant percentage of drug is absorbed per unit of time. Gabapentin is the exception, in that its intestinal absorption is saturable (ka becomes zero order at doses >1200 mg), making the ka dependent on dose. Regardless of route of administration, the more rapid the rate of absorption (shorter Tmax) the higher the Cpmax.
Both the rate at which a concentration increases and the actual peak concentration affect the probability of acute central nervous system (CNS) side effects. Extended-release dosage formulations may blunt these acute side effects by slowing the rate of drug absorption and thereby maintaining the Cpmax below the minimally toxic concentration (MTC) (Figure 45.8) while maintaining plasma concentrations above the minimally effective concentration (MEC) for 12 to 24 hours. It is hoped that manipulating the bioavailability through these dosage formulations will not only reduce toxicity, but also enhance medication adherence by allowing once-daily dosing.
The route of drug administration influences the absorption rate and thereby affects the onset of drug action. IV administration increases drug levels fastest, making the drug available for entry into tissues nearly instantaneously. IM administration of some drugs results in slower absorption than oral administration. In the case of lipophilic drugs with relatively poor aqueous solubility, such as phenytoin, absorption after IM administration is slow and erratic, although eventually complete. Among the AEDs, phenobarbital, fosphenytoin, and midazolam may be rapidly and reliably absorbed after IM administration. Phenobarbital and phenytoin (after fosphenytoin) concentrations usually reach peak values in less than 90 minutes and 30 minutes after an IM dose, respectively (14,15).
Oral administration of AEDs is the cornerstone of the treatment of epilepsy. By and large, drugs with erratic or low bioavailability are not suitable for chronic therapy. Drugs with rapid absorption and short half-lives are manufactured in delayed or slowly absorbed formulations to help sustain drug concentrations between doses. As a general principle, slowly absorbed formulations are preferable for chronic therapy because they attenuate the fluctuations in drug concentrations between doses. Examples include valproate (Depakote® ER Sprinkles), carbamazepine (Tegretol XR® and Carbatrol), and others (Table 45.3).
FIGURE 45.8 Immediate-versus sustained-release pharmacokinetic profile. In the figure, three different dosage forms of the same medication are shown. The immediate release (IR) dosage form results in large variations in peak–trough concentrations. Concentrations above the MTC can result in peak-related side effects (nausea, vomiting, dizziness), whereas concentrations below the MEC may result in subtherapeutic anticonvulsant concentration and seizures. In clinical practice, we try to maintain drugs within the therapeutic range (below the MTC and above the MEC) to maximize the potential benefits of the anticonvulsant. To minimize the larger variations that occur with anticonvulsants with short half-lives, two approaches may be used. The first is to give the IR dosage form dose more frequently (lower Cpmax, higher Cpmin). The second is to give a sustained-release dosage form that releases the drug slowly, such that the peak–trough fluctuations are small. This further has the advantage of less frequent dosing, which may improve patient adherence.
Rectal administration is important for patients who take drugs that lack parenteral formulations and/or for emergency situations when drugs cannot be administered either intravenously or orally. Rectal administration is also helpful when epileptic patients with epilepsy undergo surgery and cannot take their medications by mouth (16). Lipophilic agents, such as diazepam, are rapidly and well absorbed after rectal administration (17). Typically, drugs in solution are absorbed faster and more reliably than drugs in suppositories.
Many AEDs have been given rectally (eg, diazepam, clonazepam, lorazepam, valproic acid, carbamazepine, paraldehyde, and phenytoin) (17,18,19). Diazepam has an extensive history of rectal administration and has been used to interrupt serial seizures and status epilepticus. After rectal administration of diazepam solutions, peak concentrations usually occur in less than 10 minutes (20,21). The absorption of carbamazepine suspension following rectal administration is similar to that seen after oral administration; however, rectal administration of carbamazepine produces a strong urge to defecate (22).
Bioavailability
Bioavailability is the quotient of the areas under the concentration–time curves for a nonintravenous dose divided by that for an IV dose. For example, the area under the curve after an oral dose divided by the area after the same dose IV is the bioavailability of the orally administered formulation. Bioavailability is expressed as either a fraction or a percentage.
Two terms that are commonly mistaken for one another are bioavailability and bioequivalence. Absolute bioavailability (F) is a measure of the extent of drug absorption. It is independent of time and refers to the proportion of active drug that reaches the systemic circulation following nonparenteral administration; it is used to determine the effective dose (1,2). The values of F range from 0 to 1, with a value of 0.0 de noting no drug absorption and a value of 1.0 de noting complete absorption. All of an intravenously administered drug reaches the systemic circulation; hence, its value for F is 1.0. The absolute bioavailability generally decreases when a medication is given via an extravascular route. This decrease may be due to incomplete dissolution–absorption of a dosage form or because of extensive first-pass hepatic metabolism following oral administration.
Relative bioavailability describes the proportion of drug that reaches the systemic circulation when two different products of the same drug (tablet A and tablet B) or formulations (liquid, tablet, capsule) are administered. An understanding of bioavailability can be important when converting a patient from differing dosage forms (eg, an IV to tablet and vice versa) or products (eg, from brand to a generic). Nonequivalent conversion of a dose and interval can lead to a decrease in efficacy or an increase in toxicity.
Several factors reduce the bioavailability of an orally administered drug. Obvious reasons for failure to absorb a drug are GI disease, lack of tablet dissolution, and drug insolubility. Bioavailability is also reduced when drugs are metabolized in the GI tract or in the liver before they reach the systemic circulation (so-called first-pass metabolism). Other medications (prokinetics-metoclopramide, cation-containing antacids, sequestrants-cholestyramine) and food can affect the bioavailability of some AEDs. For example, the bioavailability of gabapentin and phenytoin is decreased by concurrent administration of antacids and G-tube feedings, respectively (23,24,25). Clinically these factors are important: if absorption is decreased, this increases the risk of subtherapeutic plasma concentrations, resulting in an increased likelihood of seizures. For most AEDs, food does not affect bioavailability, although it may delay the absorption (eg, increased Tmax).
Bioequivalence and Therapeutic Equivalence
Bioequivalence compares both the rate and the extent of absorption without the requirement for an IV dosage formulation. It can be thought of as the interchangeability of two dosage forms (eg, immediate versus sustained release) or products (eg, brand versus generic). The bioequivalence of various oral formulations is determined before generic formulations are authorized for marketing. Operationally, the absorption rate, time to peak concentration, and area under the concentration–time curves (AUC) for different formulations are compared.
According to the U.S. Food and Drug Administration, two drugs are bioequivalent if 90% of the confidence interval of the pharmacokinetics parameters fall within 80% to 125% of the brand name drug (Figure 45.9). While these parameters may be acceptable for most drugs, these tolerance limits may be too broad for AEDs that have a narrow therapeutic range or for some patients with difficult-to-control epilepsy.
Therapeutic equivalence is another term associated with the use of generic drugs. In order to be therapeutically equivalent, two different formulations of the same medication must be expected to exert the same clinical effect when given to the same treatment population. This definition includes two products or dosage forms being bioequivalent.
Generic AEDs
Most generics differ by 10% or less in AUC0−t compared to the reference brand drug, but some drugs with a narrow therapeutic range may exhibit differences that could be clinically significant. Generic substitution has the potential to reduce the cost of anticonvulsant therapy (26,27). Generic formulations are produced by several manufacturers and are distributed by numerous intermediate suppliers. While this may facilitate lower cost through open market competition, recent studies suggest a critical examination of this cost savings, in light of increased use of medical resources and emergency services occurring after a change from a brand to generic formulation (27,28). At times, it is difficult to determine which generic product a patient has been receiving, as chain pharmacies often align with the strongest and best-positioned suppliers, which may result in inventory disruption and product switches. Despite the need for low-cost AEDs, the overriding goal of treating epilepsy is to administer enough drug to prevent seizures while maintaining drug concentration below those that cause dose-related toxicity in the individual patient (26, 27).
FIGURE 45.9 Testing for bioequivalence.
As discussed previously, the magnitude of expected fluctuation in serum concentrations between doses depends on the drug elimination rate and the absorption rate (27). Generally, generic AEDs have faster absorption rates than brand name products; hence, substitution may increase concentration fluctuation between doses and increase the likelihood of both subtherapeutic and supratherapeutic values. When a formulation is absorbed rapidly, it produces a higher peak concentration (Figure 45.10). While this may impact the half-life determined from the alpha phase (Figure 45.2B), it does not affect the apparent half-life. This is not true for a drug that undergoes nonlinear kinetics (eg, phenytoin). Switching among formulations of phenytoin, which have different absorption rates and apparent bioavailability, may pose clinical problems. As a result, steady-state concentrations can change significantly when phenytoin formulations are switched. For this reason, generic substitution of phenytoin should be prohibited (27,29–32).
For carbamazepine, which has an intermediate to short half-life (18 hours or less, depending on the patient), the issue of generic substitution is complex. Changing the formulation can modify the extent of fluctuation between doses, but not alter the average concentration at steady state unless there is also a problem with tablet dissolution and bioavailability. For some patients who require only low carbamazepine levels to hold seizures in abeyance, generic substitution works well. For others who have a narrow therapeutic index for carbamazepine, generic substitution is highly problematic (27,30,31,32). For certain drugs such as phenobarbital, which has a very long half-life, this is a trivial issue and generic substitution is indicated. These types of patients are at risk of experiencing both toxicity and recurrent seizures if they are switched to a formulation that is more rapidly absorbed. Thus, the patient and the treating physician should be consulted when generic carbamazepine substitution is contemplated. Generic substitution is best avoided in seizure-free patients (33–35,36).
FIGURE 45.10 Effect of rapid versus slow absorption rate on fluctuations in drug levels between doses.
MEC, minimum effective concentration; MTC, minimum toxic concentration.
Source: Reproduced with permission from Ref. (72). Dodson WE. Aspects of antiepileptic drug treatment in children. Epilepsia. 1988;29(Suppl 3):S10–S14. John Wiley & Sons.
Age-Related Effects on Absorption
The impact of normal growth and development on the processes of drug absorption, distribution, metabolism, and elimination has been well characterized and can be used to predict age-specific changes in systemic drug exposure. Developmental pharmacokinetic information can also be used to develop dosing guidelines that achieve a target level of exposure and, theoretically, response in a pediatric patient.
Age-related changes in GI physiology (eg, gastric pH, stomach and intestinal emptying times) have been noted in the pediatric population and may affect the rate and extent of drug absorption (37). Intragastric pH at birth ranges between 6 and 8, but rapidly decreases to 1 to 3 within 24 to 48 hours (38). The pH returns to neutral by around 8 days of life and subsequently declines to achieve adult values around 2 years of age (39,40). The higher pH in neonates and young infants is attributed to a decrease in basal acid output that is accompanied by a total decrease in the volume of gastric secretions (39). This phenonomen may have a protective effect on acid-labile drugs with a low pKa (eg, penicillins), which likely contributes to higher bioavailability of these agents in neonates and infants. The bioavailability of orally administered weak acids, such as phenytoin and phenobarbital, may be reduced in infants and young children owing to increased ionization under achlorhydric conditions (41,42). Bioavailability of oral phenytoin is only 75% in neonates and infants up to 4 months of age; hence, larger oral doses may be required in order to achieve desired serum concentrations. Switching from intravenous to oral drug administration leads to slower drug absorption, reducing and delaying peak concentrations. In the case of phenytoin, slowed absorption causes a reduction in the apparent bioavailability of 20% to 30% (13). The time to peak serum concentration is also significantly delayed in neonates after a single oral dose of phenobarbital (43). Carbamazepine also has poor oral absorption, especially in the first 2 years of life, limiting its use in this age group.
Gastric emptying time and intestinal motility both contribute to the rate of drug absorption in the small intestine. Generally, the rate of absorption in neonates is slow owing to irregular and unpredictable peristaltic activity; hence, the time required to achieve peak serum concentration may be prolonged (44). Gastric emptying time approaches adult values within the first 6 to 8 months of life. Prolonged gastric emptying time during the neonatal period may account for delayed absorption of orally administered phenobarbital (45) and erratic absorption of some sustained-release products in infants and young children. Immaturity of the intestinal mucosa contributes to lower motility and proteolytic enzyme activity as well as greater intestinal permeability. Other factors, such as reduced intestinal absorption surface area and shorter gut transit time, may also be responsible for the delayed absorption. Active and passive transport are generally complete by 4 months of age (45).
Age-dependent changes in biliary function and activity of pancreatic lipase enzymes can affect the body’s ability to solubilize and subsequently absorb lipophilic drugs. The GI tract is rapidly colonized with bacteria after birth. Level of microflora related to bile acid deconjugation activity and B-glucuronidase activity suggest that activity of both is higher in neonates. Adult patterns are generally not reached until 9 years of age (46).
Age-related changes in the activity of drug-metabolizing enzymes and transport systems located in the intestines might also affect drug bioavailability. While duodenal and jejunal epoxide hydroxylase and glutathione peroxidase are not influenced by age (47), intestinal CYP1A1 may increase with age (47) and glutathione-S-transferase may decrease with increasing age (48). The marked decrease in oral clearance of midazolam in preterm infants may be attributed to immature intestinal CYP3A4, which results in decreased presystemic intestinal metabolism and increased bioavailability (49). Gabapentin absorption is dependent on the L-amino acid transporter system in the intestinal membrane. Oral clearance of gabapentin is 33% higher in children under 5 years of age compared to either children aged 5 to 12 years or adults (50). Immature activity of the L-amino acid transporter system is believed to be responsible for this effect (51). P-glycoprotein (P-gp or MDR1), an efflux transporter, may also play a role in intestinal absorption of select drugs. Analysis of MDR1 expression in neonatal intestinal tissue shows relatively low levels. The expression increases with age to reach maximum levels in young adults (15–38 years of age) (52).
AEDs are also given via the rectal (21), intranasal (53), and buccal (54) routes and have been used successfully in controlling acute seizures in the pediatric patient. While rectal absorption of diazepam and valproate is rapid, systemic concentration may vary depending on which dosage form is given (55). Some of the variability may relate to location of administration. For example, drugs administered into the superior portion of the rectum will enter the portal circulation and be subjected to first-pass metabolism by the liver. Drugs given in the lower rectum are absorbed via the hemorrhoidal vein, which is part of systemic circulation; hence, drug given here would not be subject to first-pass effect. Although buccal and sublingual routes also bypass gastric and hepatic first-pass metabolism, bioavailability can be incomplete, as the drug is often swallowed. Intranasal midazolam administration results in higher serum concentrations, faster onset of action, and more effective seizure control than that noted with rectal administration (56–59).
The absorption of intramuscularly administered drugs is influenced by patient characteristics (eg, blood flow to the site, muscle mass and activity, and quantity of adipose tissue). Properties of the drug that influence absorption include solubility at extracellular pH, ability to diffuse across capillary membranes, and surface area over which the injection spreads. Presence of hypoperfusion, dehydration, and vasomotor instability may also impede absorption. Absorption may be delayed in neonates owing to a reduction in blood flow to skeletal muscles and ineffective muscle contractions (37). Hydrophobic medications, such as diazepam and phenytoin, have minimal systemic absorption when given by this method. Phenytoin also may form insoluble crystals at the injection site that may be associated with local hemorrhage and muscle necrosis. Absorption of select hydrophilic medications (eg, aminoglycosides) is greater in infants than children.
Percutaneous absorption of drugs through skin may be greater in newborns and infants, as the stratum corneum is thinner (60). Other influential factors include better hydration of the epidermis, greater perfusion of the subcutaneous layer, and a larger ratio of total body surface area to body mass compared to adults (61). Collectively, these contribute to permeability rates that may be 100- to 1,000-fold greater before 30 weeks gestation and to rates that are threefold to fourfold greater in full-term neonates compared to adults (62). Thus, topically applied agents (such as corticosteroids, isopropyl alcohol, and hexachlorophene) can have unanticipated systemic absorption, which has resulted in toxicity (62,63,64). Conversely, enhanced absorption has also been used in a beneficial manner (eg, povidone-iodine, caffeine) (65).
DRUG DISTRIBUTION
Distribution involves the movement of drugs from the systemic circulation into various body compartments and tissues. It is influenced by body compartment size and composition, protein binding, and hemodynamic factors (eg, cardiac output, regional blood flow, membrane permeability).
Although it is the unbound drug concentration in plasma that is in equilibrium with the unbound drug concentration in the brain, total (bound plus unbound) anticonvulsant serum concentrations are generally measured in clinical practice. While the unbound value correlates better with drug action than does the total concentration (66,67), measuring the total drug concentration usually works well because total concentrations correlate highly with the unbound concentrations in most patients. High concentrations of valproate exceed the binding capacity of plasma constituents, resulting in a nonlinear relationship between the total valproate level and the unbound valproate level (68). When total valproate concentrations are high, the unbound valproate level increases disproportionately (69).
Various AEDs differ in the extent to which they are bound to serum constituents (70,71) (Table 45.2). The binding of ethosuximide, phenobarbital, and primidone is so low as to be clinically irrelevant. However, the binding of carbamazepine, phenytoin, and valproate is sufficiently high that significant changes in unbound drug levels occur when binding is altered (72). Conditions that cause hypoalbuminemia, such as renal disease and hepatic disease, and certain drug interactions with acidic compounds, such as fatty acids, aspirin, and valproate, increase the unbound drug concentrations of phenytoin and carbamazepine. Newborns also have reduced drug–protein binding because of fetal albumin and hypoalbuminemia (37,73). When drug–protein binding is disturbed, therapeutic ranges based on total levels of phenytoin and of carbamazepine no longer apply.
Age-Related Effects on Distribution
As noted earlier, distribution involves the movement of drugs from the systemic circulation into various body compartments and tissues and is influenced by body compartment size and composition, protein binding, hemodynamic factors, and membrane permeability. Physiochemical properties of the drug (molecular size, ionization constant, and aqueous/lipid solubility) also affect distribution.
Body Compartments
Volume of distribution can be influenced by alterations in the body water compartment size, which involves the relative proportion of total body water, total extracellular water, and total body fat. Total body water is inversely related to the amount of fat tissues in the body and constantly fluctuates during the first year of life. In the term infant, total body water accounts for about 75% of body weight (74). By 6 months of age, body fat has increased to 30% and total body water has decreased to 60%. By puberty, total body water approaches adult values of about 50%. These differences may impact the volume of distribution depending on the physiochemical characteristics of the drug. Highly water-soluble compounds, such as gentamicin, phenobarbital, or propofol, have larger volumes of distribution in neonates compared to adults. Conversely, body fat increases with age, from 1% to 2% in a preterm neonate to 10% to 15% in a term neonate. Lipophilic drugs, such as diazepam, tend to have smaller volumes of distribution in infants than in older children and adults (75). By puberty, girls have about twice the amount of body fat as males.
Protein Binding
The second important age-related difference concerns changes in protein binding. Although it is the unbound drug concentration in plasma that is in equilibrium with the unbound drug concentration in the brain, total (bound plus unbound) anticonvulsant serum concentrations are generally measured in clinical practice. While the unbound value correlates better with drug action than does the total concentration (66,67,76), measuring the total drug concentration usually works well because total concentrations correlate highly with the unbound concentrations in most patients.
Plasma–protein binding is dependent on the available binding proteins, the number of binding sites, the affinity constant of the drug for a specific protein, and the presence of endogenous compounds (eg, bilirubin, fatty acids) or diseases (eg, acid–base status) that may alter the drug–protein interaction. In general, acidic drugs (eg, phenytoin) bind to albumin and basic drugs (eg, carbamazepine) bind to alpha1-acid glycoprotein (AAG) and lipoproteins (77).
Plasma–protein binding tends to be reduced in neonates and infants (75,77,78,79) and does not reach adult values until about 1 year of age (80). This is due to a reduction in the total amount of plasma proteins, a qualitatively different albumin that has diminished binding affinity (ie, fetal albumin), and presence of endogenous competing substrates (eg, increased bilirubin and free fatty acids). The concentration of AAG is low at birth, but increases over the first year to reach adult values. These changes may cause a decrease in the protein binding of phenobarbital and an increased volume of distribution in neonates (75). Likewise, interpretation of the total serum concentration for drugs that are highly protein bound and have a narrow therapeutic range (eg, phenytoin) can be difficult (81). Highly bound acid drugs (eg, phenytoin) can compete for bilirubin-binding sites on albumin to displace bilirubin and thereby theoretically increase blood levels of unconjugated bilirubin; this may increase the risk of kernicterus in neonates (82).
High concentrations of valproate exceed the binding capacity of plasma constituents, resulting in a nonlinear relationship between the total valproate level and the unbound valproate level (83). When total valproate levels are high, the unbound valproate level increases disproportionately (69).
Various AEDs differ in the extent to which they are bound to serum constituents (70,71) (Table 45.2). The binding of ethosuximide, phenobarbital, and primidone is so low as to be clinically irrelevant. However, the binding of carbamazepine, phenytoin, and valproate is sufficiently high that significant changes in unbound drug levels occur when binding is altered (72). Conditions that cause hypoalbuminemia, such as renal disease and hepatic disease, and certain drug interactions with acidic compounds, such as fatty acids, aspirin, and valproate, increase the unbound drug concentrations of phenytoin.
Membrane Permeability
At birth, the blood–brain barrier (BBB) is not fully developed; hence, this allows drugs with lower penetration capacity to easily gain access to the CNS to increase the likelihood of toxicity (83). The volume of the CNS is large in younger children and reaches 80% to 90% of adult values by 4 to 6 years of age. It does not correlate well with body surface area, which reaches adult values by 16 years of age. These data suggest that cerebrospinal fluid concentration in young children compared to adults would be lower following intrathecal administration (84,85).
DRUG METABOLISM (86)
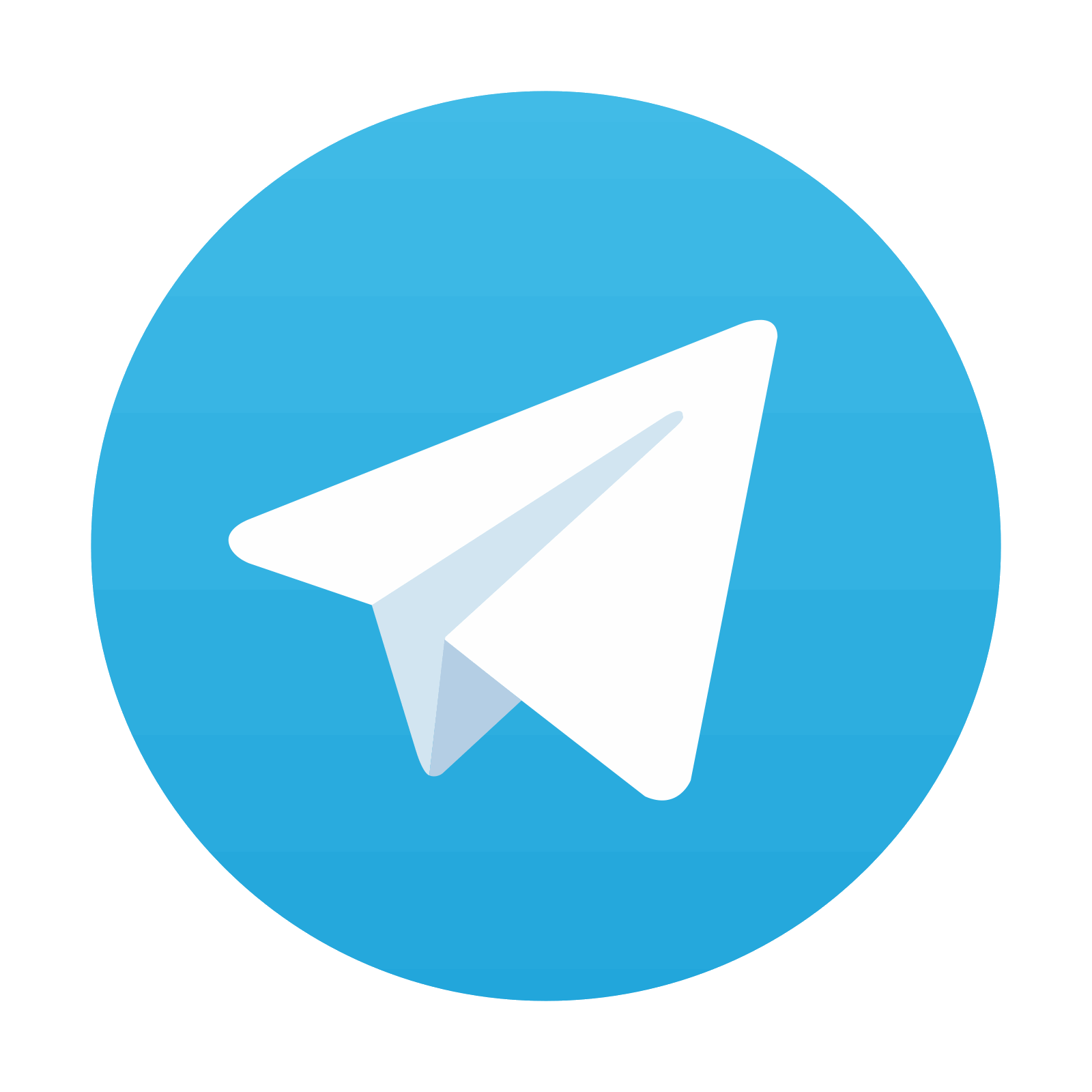
Stay updated, free articles. Join our Telegram channel
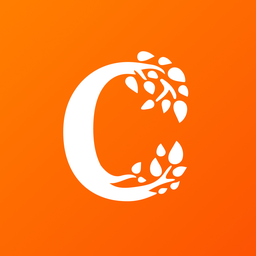
Full access? Get Clinical Tree
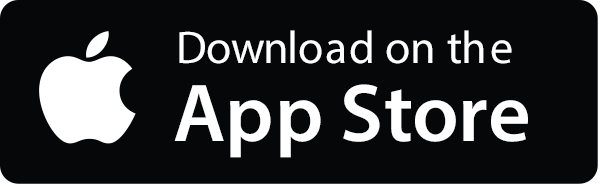
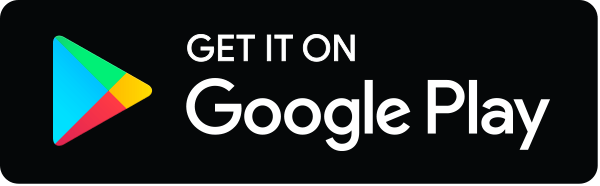