Abbreviations
aCGH
Array comparative genomic hybridization
AF
Amniotic fluid
CRISPR
Clustered regularly interspaced short palindromic repeat
CVS
Chorionic villus sampling
FISH
Fluorescence in situ hybridization
HDR
Homology directed repair
ICM
Inner cell mass
ICSI
Intracytoplasmic sperm injection
mtDNA
Mitochondrial DNA
nDNA
Nuclear DNA
NGS
Next-generation sequencing
NHEJ
Nonhomologous end joining
cfDNA
cell-free DNA
PCR
Polymerase chain reaction
PGD
Preimplantation genetic diagnosis
PGS
Preimplantation genetic screening
PGT
Preimplantation genetic testing
PGT-A
Preimplantation genetic testing for aneuploidies
PGT-M
Preimplantation genetic testing for monogenic/single-gene defects
PGT-SR
Preimplantation genetic testing for chromosomal structural rearrangements
qPCR
Quantitative polymerase chain reaction
SNP
Single nucleotide polymorphism
STR
Short tandem repeat
TE
Trophectoderm
UPD
Uniparental disomy
WGA
Whole-genome amplification
Introduction
Over the past two decades, in vitro fertilization has become routine clinical practice. Technological advances in genetics and DNA amplification now allow preimplantation diagnosis (PGD in the past, now known as preimplantation genetic testing for monogenic/single-gene defects [PGT-M]) to be performed by testing a few cells of a developing preimplantation (usually day 5 or day 6) blastocyst embryo. This technique was developed initially to benefit couples at high risk of producing an embryo with a known genetic disorder. Preimplantation testing allows for selection of unaffected embryos for implantation and avoids the emotional stress of termination of an affected pregnancy. Most of these diagnoses are for heritable Mendelian disorders.
Because first-trimester and earlier miscarriages are known to have aneuploidy rates in excess of 40%, screening preimplantation embryos for aneuploidies was proposed to potentially improve implantation rates and take-home pregnancy rates. Preimplantation aneuploidy testing (PGS in the past, now known as preimplantation genetic testing for aneuploidies [PGT-A]) was specifically applied to improve singleton embryo transfer pregnancy rates and therefore reduce twinning and higher-order pregnancy rates, to improve outcomes for patients with recurrent miscarriages, and to improve pregnancy rates for women of advanced maternal age. Randomized controlled trials to support many of these indications are lacking, although there is significant support for testing among experts.
Preimplantation genetic testing (PGT) is advancing rapidly and will continue to benefit from new genomic technologies. Coupled with preconception carrier screening of parents, whole-genome sequencing of embryo genomes can theoretically diagnose most heritable and de novo Mendelian disorders. Noninvasive approaches to embryo genotyping show promise and will likely make PGT more widespread. Genome editing technologies are raising tantalizing possibilities of correcting pathogenic variants in affected embryos and fears that such technologies will be used to “enhance” embryos for certain traits. Moreover, PGT raises many ethical questions before conception including whether to test for adult onset disorders; whether to use for sex selection outside of medical indications; and whether to transfer embryos with identified pathogenic variants because of family wishes. It is important to keep in mind that any form of genetic testing of the embryo or parents should involve genetic counseling and careful documentation of such counseling by the healthcare provider. Vigorous research in the area of preimplantation genetic testing needs to continue to further understand the fundamentals of embryo development and benefits/clinical utility of genomic testing.
What are the Rates of Aneuploidy at Different Stages of Embryo Development?
Incorrect number of chromosomes (aneuploidy) is the most common contributor to miscarriage and congenital birth defects in humans. Aneuploidy can occur in dividing cells as the result of chromosome missegregation during meiosis or mitosis, leading to gametes with an extra or missing chromosome. Chromosome nondisjunction during meiosis of the oocytes is the cause of most trisomic/monosomic embryos, and the risk of aneuploidy increases with maternal age. Chromosome gains and losses happen during spermatogenesis; however, male surveillance mechanisms are thought to eliminate aneuploid sperm at higher efficiency than eggs. After oocyte fertilization, the resulting zygote, a single diploid cell, undergoes rapid mitotic cell divisions to develop into an 8-cell blastomere at day 3 postfertilization and into a 100- to 200-cell blastocyst at days 5–6 ( Fig. 15.1A ). Human embryos at the early stages (days 1–3) of development are susceptible to unequal distributions of chromosomes or chromosome misallocation between the daughter cells ( Fig. 15.1B ). Therefore, an elevated level of chromosomal anomalies is commonly present at the day 3 embryo biopsy ( Fig. 15.2A ). A systematic review of data from a large cohort study showed that ∼35% of embryos in day 3 biopsies are euploid, whereas ∼65% of embryos are aneuploid or contain both normal and abnormal cells (mosaic aneuploid embryos). The rate of mitotic aneuploidy (aneuploidy that arises postfertilization, also termed postzygotic) is relatively similar among women of all age groups, suggesting that the occurrence of aneuploidy by postzygotic mitotic errors is not influenced by maternal age and equally affects maternal and paternal chromosomes. Furthermore, individual couples with compromised response to repair double-stranded DNA breaks that occur during meiosis and mitosis may experience extremely high rates of aneuploidy and chromosomal rearrangements.


Importantly, comparison between the chromosome status of embryos on day 3 and day 5 demonstrates that ∼20–30% of aneuploid embryos that developed to the blastocyst stage undergo self-correction. Thus, the rate of euploid embryos at day 5 is higher than the proportion of chromosomally normal embryos at day 3 ( Fig. 15.2B ). Postzygotic aneuploidy resulting from zygotic chromosome loss, chromosome gain, or mitotic nondisjunction is a common defect in human embryos, and the process of embryo self-correction via apoptosis and death of aneuploid cells or growth advantage for proliferation of chromosomally normal cells requires further investigation.
What Is the Difference Between Inner Cell Mass and Trophectoderm?
The fertilized egg is totipotential, capable of differentiation into any cell type. After asymmetric division, a few cells positioned inside the embryo remain pluripotent and will give rise to the inner cell mass (ICM), whereas the rest differentiates into trophectoderm (TE). The ICM will become the fetus, whereas trophectoderm (TE) will give rise to the placenta and umbilical cord ( Fig. 15.1A ). This differentiation into ICM versus TE occurs circa the 16-cell stage (day 4–5). The ICM can develop into any specialized cell in the human body. At the blastocyst stage, most of the aneuploidies appear to be meiotic in origin and present in both ICM and TE cells. Mitotic errors during the first cleavage divisions result in mosaicism for aneuploid cells and random distribution of abnormal cells within a developing embryo, which can cause discrepant findings between ICM and TE in ∼3.3% of blastocysts. The frequency of mitotic aneuploidies in human preimplantation embryos can be deduced from the frequency of mosaic embryos. Mosaic embryos may implant at a lower success rate, but more investigation is necessary to determine its clinical utility.
What Are the Different Embryonic Stages at Which Diagnostics Apply?
Testing can be performed on a biopsy obtained from either a polar body, blastomere at the cleavage stage (day 3 after fertilization), or trophectoderm at the blastocyst stage (day 5 or 6 after fertilization). The earliest approach was based on polar body removal, and the genetic status of the oocyte was inferred from the results of the polar body assay. In some countries, religious influences have led to sanctity of life laws that forbid embryo biopsy, and polar body biopsy remains the only option. Polar body biopsy disadvantages include that it only provides information about the maternal contribution to the embryo and autosomal recessive disorders can only partially be diagnosed. Polar body biopsy also carries increased risk of diagnostic error because of the degradation of the genetic material or effects of recombination. The second assay to be developed was blastomere biopsy performed on embryo day 3. Initially, one or two cells were biopsied and evaluated by a fluorescence in situ hybridization (FISH) technique for aneuploidy involving a limited set of chromosomes commonly associated with first-trimester miscarriages. The FISH approach was abandoned because of a high rate of chromosomal mosaicism on day 3, the limited number (one or two) of cells available for analysis, and a large randomized trial that showed no benefit to using FISH for selected chromosomes (18, 21, 13, X, Y, 1, 16, and 17). In this trial, testing using FISH significantly reduced the rates of ongoing pregnancies and live births after IVF in women of advanced maternal age. Misdiagnosis is also more common in blastomere biopsy because of the technical limitations of DNA amplification from a single cell by PCR. Erroneous amplification of sperm DNA from the zona pellucida, and/or allele dropout of one of the parental alleles during amplification, may lead to false-negative results. Current molecular technologies using single nucleotide polymorphisms and short tandem repeats to identify informative markers from both parents can eliminate the risk of misdiagnosis because of contamination with extraneous DNA or because of allele dropout (one of the two alleles is not amplified). Nonetheless, blastomere biopsy has lost its appeal mainly because of the low number of cells that are available for analysis. At the present time, testing at the blastocyst stage (days 5–6) is most popular because of a greater number of cells available for analysis (4–10), accurate and reliable evaluation for meiotic and mitotic nondisjunction errors, and little known negative impact on embryo viability.
What Is the Difference Between PGD and PGS?
In the past, PGD referred to a targeted analysis of variants in the embryo, at risk of inheriting a Mendelian disorder, such as cystic fibrosis, from the parents. PGS referred to screening for aneuploidies to improve implantation and take-home baby rates. Recently, the terms preimplantation genetic diagnosis (PGD) and preimplantation genetic screening (PGS) have been replaced by preimplantation genetic testing (PGT) and descriptive suffixes. PGT-A refers to analysis for aneuploidies and replaces the term PGS; PGT-M replaces the term PGD and refers to testing for monogenic/single gene defects, whereas PGT-SR refers to testing for chromosomal structural rearrangements. Couples undergoing in vitro fertilization (IVF) may select specific PGT options based on the primary referral reason(s) ( Table 15.1 ).
Primary referral reason | PGT-A Aneuploidy | PGT-M Monogenic Disease ∗ | PGT-SR Structural Rearrangements | Prenatal testing |
---|---|---|---|---|
Advanced maternal age, IVF for nongenetic reasons | + | cfDNA, CVS, amniocentesis | ||
Repeated pregnancy losses | + | cfDNA, CVS, amniocentesis | ||
Donor egg/sperm | + | cfDNA, CVS, amniocentesis | ||
Infertility, repeated IVF failure, embryonic arrest | + | cfDNA, CVS, amniocentesis | ||
Family history of X-linked disorder, male lethal condition of unknown etiology | + | cfDNA, CVS, amniocentesis | ||
Gender identification/selection | + | cfDNA, CVS, amniocentesis | ||
Known alteration in a single gene (carriers of autosomal dominant or autosomal recessive condition) | + | + | CVS, amniocentesis | |
Known alteration in a single X-linked gene | + | + | CVS, amniocentesis | |
Family history of a gene defect for a late-onset autosomal dominant disorder | + | + | CVS, amniocentesis | |
Mitochondrial disorder due to a known alteration in a gene encoded by nuclear genome | + | + | Amniocentesis | |
Mitochondrial disorder due to an alteration in mitochondrial genome ^ | + | Amniocentesis | ||
HLA-matched donor | + | + | CVS, amniocentesis | |
Parent with a chromosome rearrangement | ||||
|
+ | CVS, amniocentesis, UPD | ||
|
+ | + | CVS, amniocentesis | |
|
+ | + | CVS, amniocentesis | |
|
+ | + | CVS, amniocentesis | |
|
+ | + | CVS, amniocentesis | |
|
+ | + | CVS, amniocentesis |
∗ ICSI is preferred to minimize the risk of contamination from sperm DNA.
^ Nuclear transfer or mitochondria replacement therapy may prevent the transmission of mtDNA variants.
PGT-A is used to evaluate embryos for numerical chromosomal alterations such as trisomy and monosomy and to select chromosomally balanced embryos for transfer. The incidence of aneuploidy is high in IVF-produced embryos and significantly increases with advanced maternal age. Therefore, aneuploidy testing of blastocyst stage embryos for all 24 chromosomes has been advocated to improve implantation and delivery rates. At present, however, there is insufficient evidence to recommend the routine use of blastocyst biopsy with aneuploidy testing in all infertile patients.
PGT-M is a test performed on embryos to identify their status for known genetic defect(s), such as pathogenic variants in the CFTR (cystic fibrosis) gene, when these are carried by the parent(s). The aim of PGT-M is to provide a reproductive option to parents with a risk of offspring with inherited disorders to select embryo(s) for pregnancy that are free of a genetic disease.
PGT-SR is a test to identify genomic imbalances that arise from parental structural chromosomal rearrangements such as translocations, inversions, deletions, and duplications affecting a part of chromosome. Carriers of structural chromosome rearrangements are at high risk to produce gametes with extra and missing DNA segments of rearranged chromosomes (segmental aneuploidies). Several technologies used for aneuploidy analysis can also detect segmental aneuploidy. In some patients with structural chromosome rearrangements, gametes are expected to contain imbalances for large DNA segments such as whole-chromosome aneuploidy in carriers of Robertsonian translocations. Consultation with laboratory personnel is recommended before biopsy to determine techniques and the optimal PGT-SR protocol to identify products of patient-specific chromosomal rearrangement.
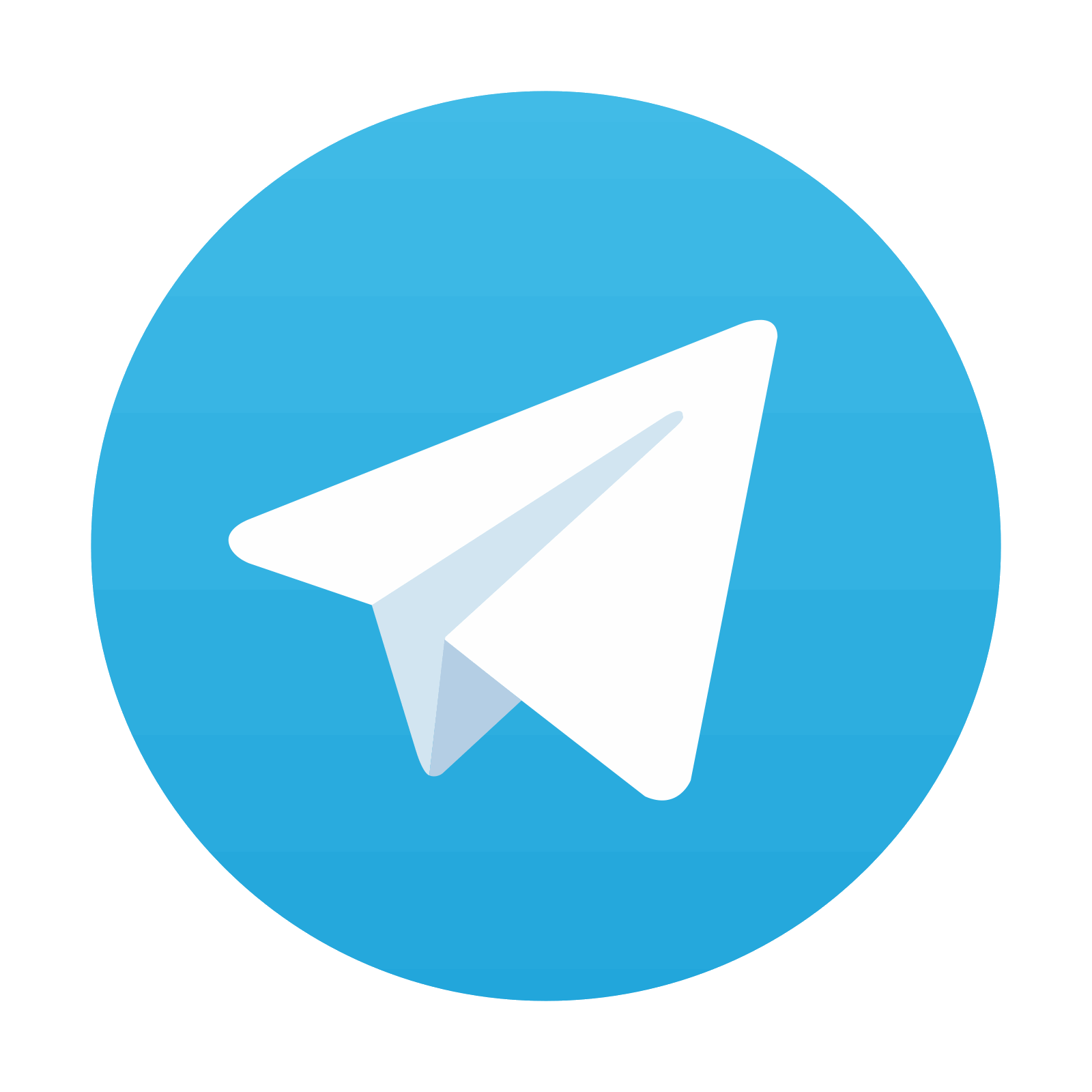
Stay updated, free articles. Join our Telegram channel
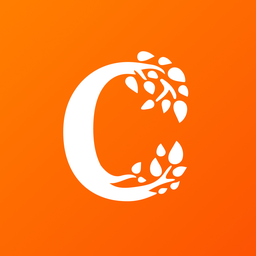
Full access? Get Clinical Tree
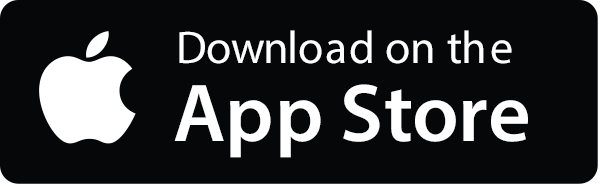
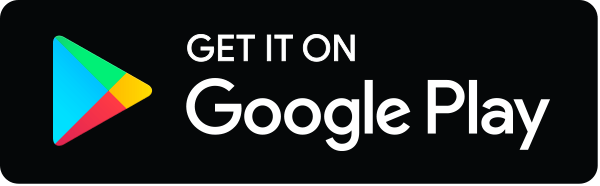