Objective
Pioglitazone (PIO), an antidiabetic drug of the thiazolidinedione family, improves glucose and lipid metabolism in muscle, adipose, and liver tissues via peroxisome proliferator-activated receptor gamma activation. We hypothesize that PIO therapy will improve the metabolic status of offspring exposed to maternal obesity in a mouse model developmentally programmed for metabolic syndrome.
Study Design
CD-1 female mice were fed a high-fat diet for 3 months prior to breeding and throughout pregnancy and lactation. The pups were weaned to a standard-fat diet. Offspring were randomly assigned to receive 40 mg/kg of PIO in 0.5% of methyl cellulose or 0.5% methyl cellulose by daily oral gavage for 2 weeks. The pre- and posttreatment total body weights of the pups were recorded. Visceral and subcutaneous adipose tissue were evaluated using microcomputed tomography. Serum analytes were measured. After treatment, minimally invasive microendoscopic fluorescence confocal imaging and intraperitoneal glucose tolerance tests were performed. The data were analyzed using appropriate statistical tests (significance, P < .05).
Results
PIO therapy resulted in lower total body weight and lower visceral adipose tissue gain and increased subcutaneous adipose tissue. PIO significantly lowered triglycerides, insulin levels, and homeostasis model assessment of insulin resistance in males and fasting glucose in females. There was a trend toward larger adipocyte size.
Conclusion
Short-term PIO therapy in the offspring of obese mothers attenuates metabolic changes associated with the developmental programming of metabolic syndrome. These novel data suggest a potential role for drugs that activate peroxisome proliferator-activated receptor gamma receptors to prevent metabolic syndrome in the adult offspring at risk to develop metabolic alterations.
According to the International Diabetes Federation, metabolic syndrome (MetS) is defined by the presence of central obesity and at least 2 of the following components: hypertension, glucose intolerance, and dyslipidemia. The MetS has reached epidemic proportions in many developed countries. Consequently, understanding the detrimental potential of obesity and its unfavorable consequences is vital to the development of preventative measures.
Traditionally, metabolic syndrome and obesity research has focused on lifestyle factors in childhood and adulthood. More recently the role of pregnancy in the obesity epidemic is beginning to be recognized. The rate of obesity in pregnant women worldwide is between 18.5% and 38.3%, and these rates continue to rise. Epidemiological and experimental studies demonstrate that offspring from obese mothers are at an increased risk of obesity as well as other features and complications of MetS. For example, the relative risk of childhood obesity associated with maternal obesity in the first trimester of pregnancy was found to be increased by 2-fold at age 2-3 years and 2.3-fold at 4 years of age.
MetS was found to be more common in adolescents who were born to obese mothers. Others have shown that the mother’s body mass index is one of the strongest predictors of overweight/obesity and percentage of body fat in her children. Adults born to obese mothers were more insulin resistant than those born to nonobese mothers. Moreover, maternal prenatal fat intake was one of the strongest predictors of fat mass in the offspring at 10 years of age. Thus, there is strong accumulating evidence that offspring born of pregnancies complicated by obesity are at increased risk of obesity and other features of MetS.
Visceral adipose tissue dysfunction is emerging as the primary defect leading to MetS. Pathological lipid accumulation and adipocyte hypertrophy, in addition to hypoxia and a variety of other processes, lead to adipose tissue dysfunction and the development of metabolic and/or cardiovascular disorders. Previous studies, including our own, have demonstrated that adipose tissue dysfunction is present in fetal programming models of MetS. The most notable examples of adipose dysfunction in fetal programming models include elevated serum leptin levels, increased adipose tissue lipid accumulation, augmented expression of angiotensin, hypoxia-inducible factor-1α and peroxisome proliferator-activated receptor gamma (PPARγ) receptor, amplified activities of lipoprotein lipase and glycerol-3-phosphate dehydrogenase, and decreased levels of antioxidant enzymes.
PPARγ is highly expressed in white and brown adipose tissue and plays a critical role in adipocyte differentiation and mature adipocyte maintenance. PPARγ regulates systemic insulin signaling via ligand-dependent transcriptional activation of target genes. In animal models, messenger ribonucleic acid expression of the PPARγ is significantly increased in the adipose tissue of the offspring of obese dams. When administered to pregnant animals, thiazolidinediones (TZDs), PPARγ agonists (TZDs) improve maternal and fetal metabolic markers. However, none of these studies followed up offspring development into adulthood, nor were there studies that investigated the effects of TZDs if administered postnatally to offspring at risk to develop MetS, such as those born to obese mothers.
In this study, we tested the hypothesis that postnatal administration of the pioglitazone, a TZD class drug, will improve the metabolic status of adult offspring at risk to develop MetS because of exposure to a maternal high-fat diet during the prenatal and the early postnatal periods.
Materials and Methods
All procedures were approved by the Animal Care and Use Committee of the University of Texas Medical Branch. The animals were housed separately in temperature- and humidity-controlled quarters with constant 12 hour light, 12 hour dark cycles and were provided with food and water ad libitum.
Study design
In our laboratory, we have established a mouse model of developmental MetS by feeding female mice a high-fat diet for 3 months before pregnancy, during pregnancy, and until weaning. At 6 months of age, offspring of these dams develop significant adiposity, hypertension, elevated fasting glycemia, and dyslipidemia when compared with pups born to mothers fed standard chow.
For the current study, female and male CD-1 mice were obtained from Charles Rivers Laboratories (Wilmington, MA). Female mice were approximately 4-5 weeks of age upon arrival. Females were fed high-fat rodent chow (34.9% fat; D12492 ; Research Diets Inc, New Brunswick, NJ) for 3 months prior to breeding, during pregnancy, and until weaning (3 weeks postpartum), when all animals (mothers and offspring) were placed on the standard chow diet (5.6% fat; Teklad 7012: Harlan Teklad LM-485 mouse/rat sterilizable diet; Harlan Teklad, Madison, WI). The fat source differed between diets: the high-fat diet used lard, and the standard-fat diet used soybean oil.
At 8 weeks of age, the offspring were subjected to fasting blood glucose measurements and blood collection for later analysis of triglyceride and insulin levels. Mice also underwent in vivo whole-body microcomputed tomography (CT) imaging. At 10 weeks of age, the pups were divided into control and treatment groups to include animals born to the same mother in both groups. The treatment group (PIO group; males, n = 6, females, n = 4) received 40 mg/kg pioglitazone (PIO; Sigma-Aldrich, St Louis, MO) dissolved in 0.5% of methyl cellulose by oral gavage (Fisher Scientific, Pittsburgh, PA). The control group (CTR group; males, n = 6, females, n = 4) received a similar amount of vehicle (0.5% methyl cellulose) only. Treatment was given daily (except on weekends) for 2 weeks, until 12 weeks of age.
The selection of dosage and timing of the treatment was based on evidence from literature, in which db/db mice, which are at risk to develop MetS (same as our animals), showed improvements in their metabolic parameters after 2-3 weeks of PIO treatment. Following the treatment, intraperitoneal glucose tolerance tests (IGTT), fasting glucose measurements, and in vivo micro-CT and confocal imaging were performed. One male per group died during posttreatment manipulations. Blood was collected for insulin and triglyceride analysis from the remaining animals, and then the offspring were euthanized. Visceral adipose tissue, including mesenteric, epididymal, and perirenal fat, was excised and weighed.
In vivo imaging
Previously validated imaging methods for the quantitative assessment of visceral (VAT) and subcutaneous (SAT) adipose tissue and liver fat infiltration in rodents were used. Experimental protocols for noninvasive CT and minimally invasive confocal imaging were optimized in our laboratory over several months prior to the study. After administering anesthesia intraperitoneally with ketamine/xylazine (80-100 mg/kg and 5-10 mg/kg, respectively), mice were imaged with a small animal micro-CT scanner (Inveon; Siemens Preclinical Solutions, Knoxville, TN). Imaging parameters were set as follows: voltage 70 kV, current 500 μA, resolution 0.107 mm, exposure time 1000 milliseconds, 520 steps and 360 degrees of rotations. Scan time was approximately 15 minutes per mouse. Mice were given supplemental oxygen via nose cone during scanning.
The transverse views of CT images (1 per animal) at the level of the fifth lumbar vertebra were selected for analysis of VAT, whereas transverse images at the level of sixth lumbar vertebra were used for analysis of SAT. The cross-sectional total body area and adipose tissue area were measured using Inveon Research Workplace software. The percentage of VAT (%VAT) and SAT (%SAT) was calculated from the cross-sectional total body area. The correlations between the weight of VAT determined after extraction and determined by CT were significant (Pearson r = 0.82, P = .003 for males and r = 0.82, P = .01 for females).
Liver and spleen radiodensity values in a transverse section between the 13th thoracic and first lumbar vertebrae were expressed as liver to spleen density ratio and compared between the groups. The radiodensity of the liver normalized to the radiodensity of the spleen (liver to spleen radiodensity ratio) indicated fat infiltration in the liver.
To quantitatively assess PIO treatment-induced changes in adipocyte size, offspring underwent high-resolution minimally invasive microendoscopic fluorescence confocal imaging using a Cell-Vizio-488 confocal microendoscope (Mauna Kea Technologies, Paris, France). A fluorescent 1% fluorescein sodium solution (0.2-0.3 mL per animal) was injected via the tail vein or intraperitoneally. A 1.8 mm probe was inserted through an abdominal incision. Confocal images of mesenteric adipocytes in VAT were exported into Image J software (National Institutes of Health, Bethesda, MD), and the cell area was measured by manually tracing the cell borders. Every cell within 5 imaging fields was measured, and the mean cell area was calculated for each animal. The mean area values were used to compare the 2 groups.
Individuals interpreting VAT, SAT liver/spleen radiodensity, and adipocyte size were blinded to the treatment/control group allocation.
Intraperitoneal glucose tolerance test
IGTTs were performed following 2 weeks of PIO or vehicle administration. Mice were fasted overnight (16-18 hours) prior to a 20 % glucose bolus (1 g/kg per mouse) by intraperitoneal injection. Blood glucose levels were recorded prior to glucose injection and at 15, 30, 60, 90, and 120 minutes after the injection. Overnight fasting and the glucose dose were selected based on our pilot studies in CD-1 mice. Sixty microliters of blood were collected via the tail vein prior to glucose injection for fasting insulin measurements. The blood was immediately centrifuged, and the serum was stored at −80°C until the insulin assay was performed.
Serologic analysis
Blood glucose levels were measured using an OneTouch Ultra glucometer (LifeScan, Milpitas, CA) after an overnight (16-18 hours) fast. Blood was collected from the offspring before and after treatment. Commercially available kits were used according to the manufacturer’s instructions to determine serum levels of triglycerides, cholesterol (BioAssay Systems, Hayward, CA), and insulin (CrystalChem, Downers Grove, IL).
Data analysis
Data were analyzed as the difference from baseline (posttreatment value minus pretreatment value) and expressed as the mean ± SEM. The area under the IGTT curve for glucose was calculated using GraphPad Prism software (GraphPad Software, Inc, La Jolla, CA). Insulin sensitivity was determined using the homeostasis model assessment of insulin resistance (HOMA-IR) and calculated by (fasting glucose level [milligrams per deciliter] × fasting insulin level [milliunits per liter])/405. Statistical significance was determined using an unpaired Student t test or a Mann-Whitney test if the data were not normally distributed. The IGTT results were analyzed using a 2-way repeated-measures analysis of variance with Bonferonni correction. A P ≤ .05 was considered statistically significant.
Results
There were no differences in total body weight, VAT and SAT area, or fasting blood glucose and insulin levels between the 2 groups of mice at the start of the study ( Tables 1 and 2 ). Triglycerides levels were significantly higher in male mice assigned to PIO group ( Table 2 , P = .01).
Variable | Nontreated | Pioglitazone treated | P value |
---|---|---|---|
Body weight, g | 38.4 ± 3.5 | 39.8 ± 4.7 | .6 |
VAT as determined by CT (% from total area) | 28.7 ± 5.7 | 34.5 ± 2.9 | .4 |
SAT as determined by CT (% from total area) | 8.3 ± 1.3 | 8.9 ± 1.8 | .7 |
Liver/spleen radiodensity, HU | 0.7 ± 0.04 | 0.6 ± 0.02 | .6 |
Triglyceride, mmol/L | 2.3 ± 0.2 | 3.1 ± 0.2 a | .01 |
Fasting glucose, mg/dL | 178 ± 11.5 | 164 ± 11.0 | .4 |
Fasting insulin, ng/mL | 0.8 ± .02 | 1.3 ± 0.2 | .1 |
HOMA-IR | 5.8 ± 2.0 | 12 ± 3.2 | .2 |
a Statistically significant difference between the groups ( P < .05).
Variable | Nontreated | Pioglitazone treated | P value |
---|---|---|---|
Body weight, g | 29.6 ± 2.4 | 32.2 ± 2.3 | .2 |
VAT as determined by CT (% from total area) | 32.8 ± 3.5 | 37.5 ± 5.5 | .7 |
SAT as determined by CT (% from total area) | 10.8 ± 2.5 | 10.9 ± 2.4 | 1.0 |
Liver/spleen radiodensity, HU | 0.7 ± 0.02 | 0.7 ± 0.03 | .9 |
Triglyceride, mmol/L | 1.9 ± 0.3 | 2.4 ± 0.4 | .5 |
Fasting glucose, mg/dL | 70 ± 5.7 | 79 ± 7.4 | .6 |
Fasting insulin, ng/mL | 0.4 ± 0.07 | 0.5 ± 0.1 | .7 |
HOMA-IR | 2.5 ± 0.5 | 2.2 ± 0.6 | .9 |
Effects of PIO treatment on outcome measures are presented in Tables 3 and 4 : the PIO-treated males and females group gained less total body weight. Treated males acquired less VAT than animals in the CTR group. Females in both groups lost VAT with the PIO group losing less than CTR mice. The area of SAT increased in PIO-treated animals. The liver to spleen radiodensity ratio increased in males and females treated with PIO, suggesting that fat infiltration in the liver decreased as a result of PIO exposure ( Tables 3 and 4 , respectively). None of these changes/parameters were statistically significant between the groups.
Variable | Nontreated | Pioglitazone treated | P value |
---|---|---|---|
Body weight, g | 4.2 ± 1.0 | 0.3 ± 2.0 | .3 |
VAT as determined by CT (% from total area) | 10.5 ± 5.0 | 6.2 ± 3.1 | .4 |
SAT as determined by CT (% from total area) | 1.2 ± 1.4 | 5.2 ± 1.8 | .2 |
Liver/spleen radiodensity, HU | 0.01 ± 0.04 | 0.08 ± 0.04 | .2 |
Triglyceride, mmol/L | 0.8 ± 0.2 | −0.9 ± 0.04 a | .02 |
Fasting glucose, mg/dL | −32.4 ± 11.9 | −55.8 ± 7.6 | .1 |
Fasting insulin, ng/mL | 0.58 ± 0.2 | −0.6 ± 0.3 a | .03 |
HOMA-IR | 3.7 ± 1.8 | −7.3 ± 2.6 a | .03 |
a Statistically significant difference between the groups ( P < .05.)
Variable | Nontreated | Pioglitazone treated | P value |
---|---|---|---|
Body weight, g | 2.3 ± 0.2 | 0.3 ± 0.9 | .34 |
VAT as determined by CT (% from total area) | −10.5 ± 5.1 | −4.8 ± 2.7 | .5 |
SAT as determined by CT (% from total area) | −0.7 ± 2.9 | 1.2 ± 1.5 | .8 |
Liver/spleen radiodensity, HU | 0.0006 ± 0.04 | 0.08 ± 0.02 | .3 |
Triglyceride, mmol/L | −0.3 ± 0.1 | −0.7 ± 0.6 | .7 |
Fasting glucose, mg/dL | 41.0 ± 8.1 | 5.5 ± 4.3 a | .04 |
Fasting insulin, ng/mL | −0.15 ± 0.07 | 0.2 ± 0.3 | .4 |
HOMA-IR | −0.7 ± 0.4 | 1.4 ± 1.3 | .4 |
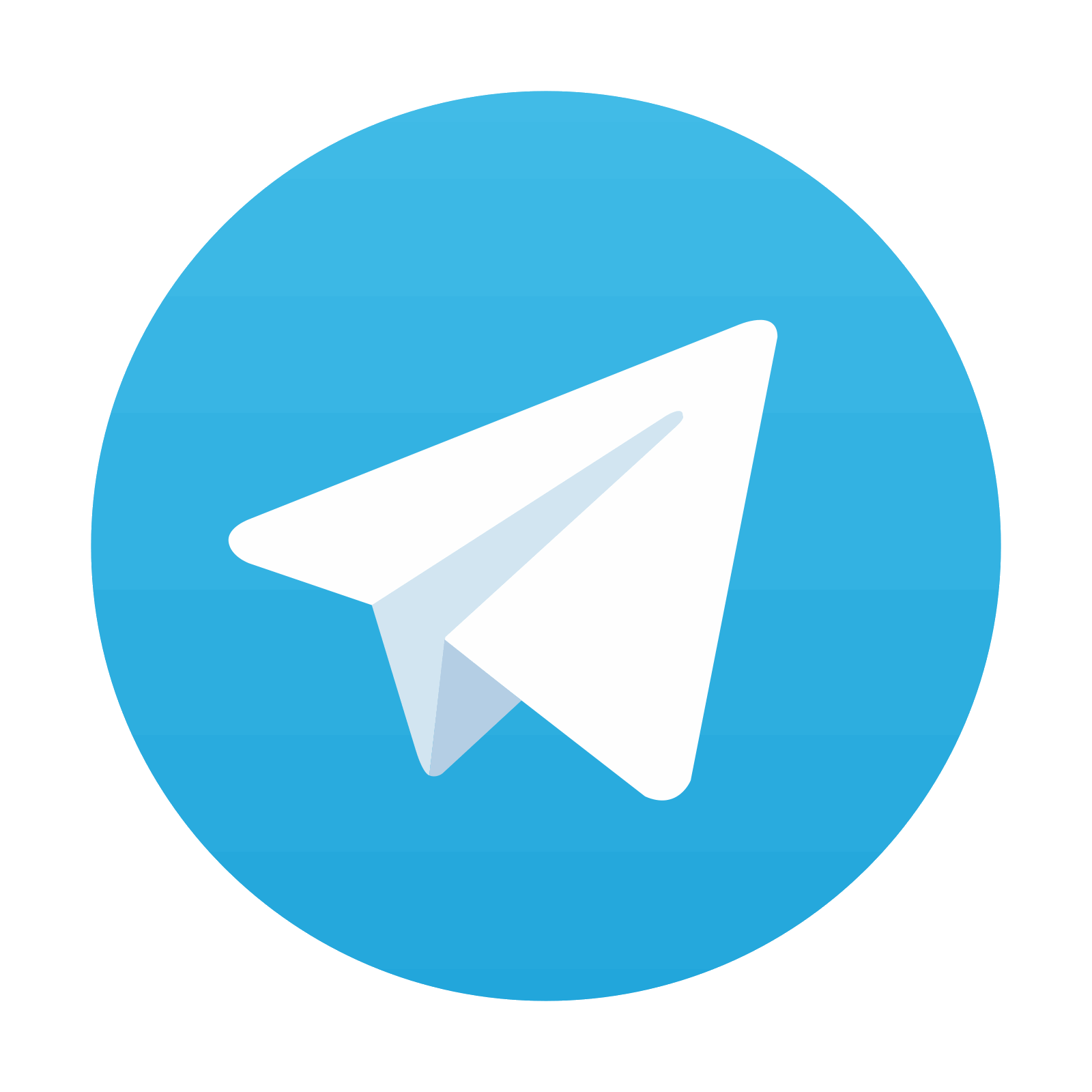
Stay updated, free articles. Join our Telegram channel
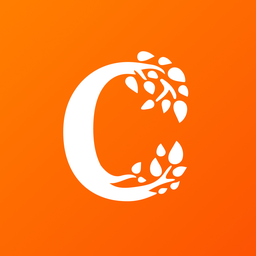
Full access? Get Clinical Tree
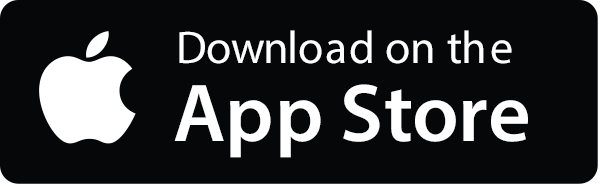
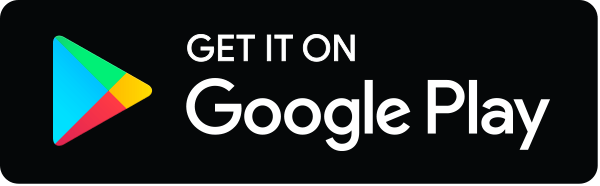