FIGURE 21-1 The phases of parturition.
FIGURE 21-2 Composite of the average dilatation curve for labor in nulliparous women. The curve is based on analysis of data derived from a large, nearly consecutive series of women. The first stage is divided into a relatively flat latent phase and a rapidly progressive active phase. In the active phase, there are three identifiable component parts: an acceleration phase, a linear phase of maximum slope, and a deceleration phase. (Redrawn from Friedman, 1978.)
Phase 1 of Parturition: Uterine Quiescence and Cervical Softening
Uterine Quiescence
Beginning even before implantation, a remarkably effective period of myometrial quiescence is imposed. This phase normally comprises 95 percent of pregnancy and is characterized by uterine smooth muscle tranquility with maintenance of cervical structural integrity. The inherent propensity of the myometrium to contract is held in abeyance, and uterine muscle is rendered unresponsive to natural stimuli. Concurrently, the uterus must initiate extensive changes in its size and vascularity to accommodate the pregnancy and prepare for uterine contractions. The myometrial unresponsiveness of phase 1 continues until near the end of pregnancy. Some low-intensity myometrial contractions are felt during the quiescent phase, but they do not normally cause cervical dilatation. Contractions of this type become more common toward the end of pregnancy, especially in multiparous women, and are referred to as Braxton Hicks contractions or false labor (Chap. 4, p. 47).
Cervical Softening
The cervix has multiple functions during pregnancy that include: (1) maintenance of barrier function to protect the reproductive tract from infection, (2) maintenance of cervical competence despite increasing gravitational forces, and (3) orchestration of extracellular matrix changes that allow progressive increases in tissue compliance.
In nonpregnant women, the cervix is closed and firm, and its consistency is similar to nasal cartilage. By the end of pregnancy, the cervix is easily distensible, and its consistency is similar to the lips of the oral cavity. Thus, the first stage of this remodeling—termed softening—is characterized by an increase in tissue compliance, yet the cervix remains firm and unyielding. Hegar (1895) first described palpable softening of the lower uterine segment at 4 to 6 weeks’ gestation, and this sign was once used to diagnose pregnancy.
Clinically, the maintenance of cervical anatomical and structural integrity is essential for continuation of pregnancy to term. Preterm cervical dilatation, structural incompetence, or both may forecast delivery (Iams, 1996).
Structural Changes with Softening. Cervical softening results from increased vascularity, stromal hypertrophy, glandular hypertrophy and hyperplasia, and slow, progressive compositional or structural changes of the extracellular matrix (House, 2009; Leppert, 1995; Mahendroo, 2012; Word, 2007). During matrix changes, collagen, the main structural protein in the cervix, undergoes conformational changes that alter tissue strength and flexibility. Specifically, collagen processing and the number or type of covalent cross-links between collagen triple helices are altered. These cross-links are normally required for stable collagen fibril formation (Canty, 2005). A reduction in cross-links between newly synthesized collagen monomers results from reduced expression and activity of the cross-link forming enzymes, lysyl hydroxylase and lysyl oxidase, beginning in early pregnancy (Akins, 2011; Drewes, 2007; Ozasa, 1981). Concurrently there is reduced expression of the matricellular proteins thrombospondin 2 and tenascin C. These proteins also influence collagen fibril structure and strength. Together, these early pregnancy changes contribute to the gradual increase in tissue compliance during pregnancy.
The clinical importance of these matrix changes is supported by the greater prevalence of cervical insufficiency in those with inherited defects in collagen and elastin synthesis or assembly (Anum, 2009; Hermanns-Lê, 2005; Paternoster, 1998; Rahman, 2003; Wang, 2006). Examples are Ehlers-Danlos and Marfan syndromes, discussed in Chapter 59 (p. 1181). Additionally, human cervical stromal cells express a transcription factor, microphthalmia-associated transcription factor (MiTF-Cx). During pregnancy, this factor maintains cervical competency by repressing the expression of genes involved in cervical dilation and parturition (Hari Kishore, 2012).
Phase 2 of Parturition: Preparation for Labor
To prepare for labor, the myometrial tranquility of phase 1 of parturition must be suspended—so-called uterine awakening or activation. This phase 2 is a progression of uterine changes during the last 6 to 8 weeks of pregnancy. Importantly, shifting events associated with phase 2 can cause either preterm or delayed labor.
Myometrial Changes
Phase 2 myometrial changes prepare it for labor contractions. This shift probably results from alterations in the expression of key proteins that control contractility. These contraction-associated proteins (CAPs) include the oxytocin receptor, prostaglandin F receptor, and connexin 43 (Smith, 2007). Thus, myometrial oxytocin receptors markedly increase along with increased numbers and surface areas of gap junction proteins such as connexin 43. Together, these lead to increased uterine irritability and responsiveness to uterotonins—agents that stimulate contractions.
Another critical change in phase 2 is formation of the lower uterine segment from the isthmus. With this development, the fetal head often descends to or even through the pelvic inlet—so-called lightening. The abdomen commonly undergoes a shape change, sometimes described by women as “the baby dropped.” It is also likely that the lower segment myometrium is unique from that in the upper uterine segment, resulting in distinct roles for each during labor. This is supported by baboon studies that demonstrate differential expression of prostaglandin receptors within myometrial regions. There are also human studies that report an expression gradient of oxytocin receptors, with greater expression in fundal myometrial cells (Fuchs, 1984; Havelock, 2005; Smith, 2001).
Cervical Ripening During Phase 2
Before contractions begin, the cervix must undergo more extensive remodeling. This eventually results in cervical yielding and dilatation upon initiation of forceful uterine contractions. Cervical modifications during this second phase principally involve connective tissue changes—so-called cervical ripening. The transition from the softening to the ripening phase begins weeks or days before onset of contractions. During this transformation, the total amount and composition of proteoglycans and glycosaminoglycans within the matrix are altered. Many of the processes that aid cervical remodeling are controlled by the same hormones regulating uterine function. That said, the molecular events of each are varied because of differences in cellular composition and physiological requirements. The uterine corpus is predominantly smooth muscle, whereas the cervix is primarily connective tissue. Cellular components of the cervix include fibroblasts, epithelia, and few smooth muscle cells.
Endocervical Epithelia
During pregnancy, endocervical epithelial cells proliferate such that endocervical glands occupy a significant percentage of cervical mass. The endocervical canal is lined with mucus-secreting columnar and stratified squamous epithelia, which protect against microbial invasion. Mucosal epithelia function as sentinels for antigens by expressing Toll-like receptors that recognize pathogens. In addition, epithelia respond in ways that lead to bacterial and viral killing. For this, the epithelia express antimicrobial peptides and protease inhibitors and signal to underlying immune cells when a pathogenic challenge exceeds their protective capacity (Wira, 2005).
In mice, studies suggest that cervical epithelia may also aid cervical remodeling by regulating tissue hydration and maintenance of barrier function. Hydration may be regulated by expression of aquaporins—water channel proteins. Maintenance of barrier function and paracellular transport of ion and solutes is regulated by tight junction proteins, such as claudins 1 and 2 (Anderson, 2006; Timmons, 2007). In the human cervical and vaginal mucosal epithelia, junctional proteins are also reported to be expressed (Blaskewicz, 2011).
Cervical Connective Tissue
Collagen. The cervix is an extracellular matrix-rich tissue. Constituents of the matrix include type I, III, and IV collagen, glycosaminoglycans, matricellular proteins, proteoglycans, and elastin. Of these, collagen is largely responsible for structural disposition of the cervix. Collagen is the most abundant mammalian protein and has a complex biosynthesis pathway that includes at least six enzymes and chaperones to accomplish maturation. Each collagen molecule is composed of three alpha chains, which wind around each other to form procollagen. Multiple collagen triple-helical molecules are cross-linked to one another by the actions of lysyl oxidase to form fibrils. Collagen fibrils interact with small proteoglycans such as decorin or biglycan, as well as matricellular proteins such as thrombospondin 2. These interactions determine fibril size, packing, and organization (Fig. 21-3). This ensures that collagen fibrils are of uniform diameter and are packed together in a regular and highly organized pattern (Canty, 2005).
FIGURE 21-3 Fibrillar collagen synthesis and organization. Collagen fibrils are assembled into collagen fibers. Fibril size and packing are regulated in part by small proteoglycans such as decorin that bind collagen. Before cervical ripening, fibril size is uniform, and fibrils are well packed and organized. During cervical ripening, fibril size is less uniform, and spacing between collagen fibrils and fibers is increased and disorganized.
During cervical ripening, collagen fibril diameter is increased, and there is increased spacing between fibrils. These changes may result in part from accumulation of poorly cross-linked collagen and reduced expression of matricellular proteins. Dispersion of collagen fibrils leads to a loss of tissue integrity and increased tissue compliance. Matrix metalloproteases (MMPs) are proteases capable of degrading extracellular matrix proteins. Of these, collagenase members of the MMP family degrade collagen. Some studies support a role of MMPs in cervical ripening. But, others suggest that the biomechanical changes are not consistent solely with collagenase activation and loss of collagen. For example, Buhmschi and colleagues (2004) performed tissue biomechanical studies in the rat and suggest that ripening correlates with changes in the three-dimensional structure of collagen rather than its degradation by collagenases. Moreover, mouse and human studies document no changes in collagen content between nonpregnancy and term pregnancy (Akins, 2011; Myers, 2008; Read, 2007).
Thus, it is likely that dynamic changes in collagen structure rather than collagen content may regulate remodeling. This point is well illustrated in specialized microscopy images of mouse and human cervical collagen (Zhang, 2012). In further support, polymorphisms or mutations in genes required for collagen assembly are associated with an increased incidence of cervical insufficiency (Anum, 2009; Paternoster, 1998; Rahman, 2003; Warren, 2007).
Glycosaminoglycans (GAGs). These are high-molecular-weight polysaccharides that complex with proteins to form proteoglycans. One glycosaminoglycan is hyaluronan (HA), a carbohydrate polymer whose synthesis is carried out by hyaluronan synthase isoenzymes. Expression of these enzymes is increased in the cervix during ripening (Akgul, 2012; Osmers, 1993; Straach, 2005). The functions of hyaluronans are dependent on size, and the breakdown of large- to small-molecular-weight molecules is carried out by a family of hyaluronidase enzymes. Hyaluronidase genes are expressed in both the mouse and human cervix, and increased hyaluronidase activity is reported in the mouse cervix at term (Akgul, 2012). Large-molecular-weight HA predominates in the mouse cervix during ripening and has a dynamic role to increase viscoelasticity and matrix disorganization. Low-molecular-weight HA has proinflammatory properties, and studies in mice and women reveal increased concentrations during labor and in the puerperium (Akgul, 2012; Ruscheinsky, 2008). The importance of regulated changes in HA size during cervical ripening and dilatation is supported by a study reporting hyaluronidase administration to the cervix for ripening in term pregnant women (Spallicci, 2007). Activation of intracellular signaling cascades and other biological functions requires interactions with cell-associated HA-binding proteins such as versican (Ruscheinsky, 2008).
Proteoglycans. These glycoproteins are composed of a protein core and GAG chains. Changes in the amount of core protein or in the number, length, or degree of sulfation of GAG chains can influence proteoglycan function. Although not well-defined, changes in proteoglycan composition are thought to accompany cervical ripening. At least three small leucine-rich proteoglycans are expressed in the cervix—decorin, biglycan, and fibromodulin (Westergren-Thorsson, 1998). In other connective tissues, decorin and other family members interact with collagen and influence the packing and order of collagen fibrils (Ameye, 2002). Collagen fibrils are rearranged in the skin of decorin-deficient mice and result in collagen fibers that are weakened, shortened, and disorganized (see Fig. 21-3). In addition to the cervix, these proteoglycans are expressed in the fetal membranes and uterus. Changes in expression levels may regulate fetal membrane tensile strength and uterine function (Meiner, 2007; Wu, 2012).
Inflammatory Changes. The marked changes within the extracellular matrix during cervical ripening in phase 2 are accompanied by stromal invasion with inflammatory cells. This has led to a model in which cervical ripening is considered an inflammatory process. As such, cervical chemoattractants attract inflammatory cells, which in turn release proteases that may aid degradation of collagen and other matrix components. In phase 3 or 4 of parturition, there is increased cervical expression of chemokines and collagenase/protease activity. It was assumed that processes regulating phases 3 and 4 of dilation and postpartum recovery of the cervix were similar to those in phase 2 of cervical ripening (Bokström, 1997; Osman, 2003; Sennström, 2000; Young, 2002). This has been challenged by observations from both human and animal studies. Sakamoto and associates (2004, 2005) found no correlation between the degree of clinical cervical ripening and the tissue concentrations of cervical neutrophil-chemoattractant interleukin 8 (IL-8). Microarray studies comparing gene expression patterns at term before and after cervical ripening report little increase in expression of proinflammatory genes. In contrast, there is a robust increase in proinflammatory and immunosuppressive genes in the cervix after delivery compared with during cervical ripening (Bollapragada, 2009; Hassan, 2006, 2009).
In mouse models, monocyte migration, but not activation, takes place before labor (Timmons, 2006, 2007, 2009). Mice deficient in the chemokine receptor CCR2, important in monocyte homing to tissues, have normally timed labor. This further supports the suggestion that labor is not initiated by an inflammatory response (Menzies, 2012). Furthermore, tissue depletion of neutrophils before birth has no effect on the timing or success of parturition. Finally, activation of neutrophils, proinflammatory M1 macrophages, and alternatively of activated M2 macrophages is increased within 2 hours after birth. This suggests a role for inflammatory cells in postpartum cervical remodeling and repair.
Induction and Prevention of Cervical Ripening
There are no therapies to prevent premature cervical ripening. Cervical cerclage is used to circumvent cervical insufficiency, although success appears limited (Owen, 2012). In contrast, treatment to promote cervical ripening for labor induction includes direct application of prostaglandins E2 (PGE2) and F2α (PGF2α). Prostaglandins likely modify extracellular matrix structure to aid ripening. Although the role of prostaglandins in the normal physiology of cervical ripening remains unclear, this property is useful clinically to assist labor induction (Chap. 26, p. 526). In some nonhuman species, the cascades of events that allow cervical ripening are induced by decreasing serum progesterone concentrations. And in humans, administration of progesterone antagonists causes cervical ripening. As discussed later, humans may have developed unique mechanisms to localize decreases in progesterone action in the cervix and myometrium.
Phase 3 of Parturition: Labor
This phase is synonymous with active labor, which is customarily divided into three stages. These compose the commonly used labor graph shown in Figure 21-2. The clinical stages of labor may be summarized as follows. The first stage begins when spaced uterine contractions of sufficient frequency, intensity, and duration are attained to bring about cervical thinning, or effacement. This labor stage ends when the cervix is fully dilated—about 10 cm—to allow passage of the term-sized fetus. The first stage of labor, therefore, is the stage of cervical effacement and dilatation.
The second stage begins when cervical dilatation is complete and ends with delivery. Thus, the second stage of labor is the stage of fetal expulsion. Last, the third stage begins immediately after delivery of the fetus and ends with the delivery of the placenta. Thus, the third stage of labor is the stage of placental separation and expulsion.
First Stage of Labor: Clinical Onset of Labor
In some women, forceful uterine contractions that effect delivery begin suddenly. In others, labor initiation is heralded by spontaneous release of a small amount of blood-tinged mucus from the vagina. This extrusion of the mucus plug that had previously filled the cervical canal during pregnancy is referred to as “show” or “bloody show.” There is very little blood with the mucous plug, and its passage indicates that labor is already in progress or likely will ensue in hours to days.
Uterine Labor Contractions
Unique among physiological muscular contractions, those of uterine smooth muscle during labor are painful. The cause of this is not known definitely, but several possibilities have been suggested: (1) hypoxia of the contracted myometrium—such as that with angina pectoris; (2) compression of nerve ganglia in the cervix and lower uterus by contracted interlocking muscle bundles; (3) cervical stretching during dilatation; and (4) stretching of the peritoneum overlying the fundus.
Of these, compression of nerve ganglia in the cervix and lower uterine segment by the contracting myometrium is an especially attractive hypothesis. Paracervical infiltration with local anesthetic usually produces appreciable pain relief with contractions (Chap. 25, p. 509). Uterine contractions are involuntary and, for the most part, independent of extrauterine control. Neural blockade from epidural analgesia does not diminish their frequency or intensity. In other examples, myometrial contractions in paraplegic women and in women after bilateral lumbar sympathectomy are normal but painless.
Mechanical stretching of the cervix enhances uterine activity in several species, including humans. This phenomenon has been referred to as the Ferguson reflex (Ferguson, 1941). Its exact mechanism is not clear, and release of oxytocin has been suggested but not proven. Manipulation of the cervix and “stripping” the fetal membranes is associated with an increase in blood levels of prostaglandin F2α metabolite (PGFM).
The interval between contractions diminishes gradually from approximately 10 minutes at the onset of first-stage labor to as little as 1 minute or less in the second stage. Periods of relaxation between contractions, however, are essential for fetal welfare. Unremitting contractions compromise uteroplacental blood flow sufficiently to cause fetal hypoxemia. In active-phase labor, the duration of each contraction ranges from 30 to 90 seconds, averaging about 1 minute. There is appreciable variability in contraction intensity during normal labor. Specifically, amnionic fluid pressures generated by contractions during spontaneous labor average 40 mm Hg, but vary from 20 to 60 mm Hg (Chap. 24, p. 498).
Distinct Lower and Upper Uterine Segments. During active labor, the anatomical uterine divisions that were initiated in phase 2 of parturition become increasingly evident (Figs. 21-4 and 21-5). By abdominal palpation, even before membrane rupture, the two segments can sometimes be differentiated. The upper segment is firm during contractions, whereas the lower segment is softer, distended, and more passive. This mechanism is imperative because if the entire myometrium, including the lower uterine segment and cervix, were to contract simultaneously and with equal intensity, the net expulsive force would be markedly decreased. Thus, the upper segment contracts, retracts, and expels the fetus. In response to these contractions, the softened lower uterine segment and cervix dilate and thereby form a greatly expanded, thinned-out tube through which the fetus can pass.
FIGURE 21-4 Sequence of development of the segments and rings in the uterus at term and in labor. Note comparison between the uterus of a nonpregnant woman, the uterus at term, and the uterus during labor. The passive lower uterine segment is derived from the isthmus, and the physiological retraction ring develops at the junction of the upper and lower uterine segments. The pathological retraction ring develops from the physiological ring. Anat. I.O. = anatomical internal os; E.O. = external os; Hist. I.O. = histological internal os; Ph. R.R. = physiological retraction ring.
FIGURE 21-5 The uterus at the time of vaginal delivery. The active upper segment retracts around the presenting part as the fetus descends through the birth canal. In the passive lower segment, there is considerably less myometrial tone.
The myometrium of the upper segment does not relax to its original length after contractions. Instead, it becomes relatively fixed at a shorter length. The upper active uterine segment contracts down on its diminishing contents, but myometrial tension remains constant. The net effect is to take up slack, thus maintaining the advantage gained in expulsion of the fetus. Concurrently, the uterine musculature is kept in firm contact with the uterine contents. As the consequence of retraction, each successive contraction commences where its predecessor left off. Thus, the upper part of the uterine cavity becomes slightly smaller with each successive contraction. Because of the successive shortening of the muscular fibers, the upper active segment becomes progressively thickened throughout first- and second-stage labor (see Fig. 21-4). This process continues and results in a tremendously thickened upper uterine segment immediately after delivery.
Clinically, it is important to understand that the phenomenon of upper segment retraction is contingent on a decrease in the volume of its contents. For this to happen, particularly early in labor when the entire uterus is virtually a closed sac with only minimal cervical dilatation, the musculature of the lower segment must stretch. This permits an increasing portion of the uterine contents to occupy the lower segment. The upper segment retracts only to the extent that the lower segment distends and the cervix dilates.
Relaxation of the lower uterine segment mirrors the same gradual progression of retraction. Recall that after each contraction of the upper segment, the muscles do not return to their previous length, but tension remains essentially the same. By comparison, in the lower segment, successive lengthening of the fibers with labor is accompanied by thinning, normally to only a few millimeters in the thinnest part. As a result of the lower segment thinning and concomitant upper segment thickening, a boundary between the two is marked by a ridge on the inner uterine surface—the physiological retraction ring. When the thinning of the lower uterine segment is extreme, as in obstructed labor, the ring is prominent and forms a pathological retraction ring. This abnormal condition is also known as the Bandl ring, which is discussed further and illustrated in Chapter 23 (p. 470).
Changes in Uterine Shape During Labor. Each contraction produces an elongation of the ovoid uterine shape with a concomitant decrease in horizontal diameter. This change in shape has important effects on the labor process. First, there is increased fetal axis pressure, that is, the decreased horizontal diameter serves to straighten the fetal vertebral column. This presses the upper pole of the fetus firmly against the fundus, whereas the lower pole is thrust farther downward. The lengthening of the ovoid shape has been estimated at 5 and 10 cm. Second, with lengthening of the uterus, the longitudinal muscle fibers are drawn taut. As a result, the lower segment and cervix are the only parts of the uterus that are flexible, and these are pulled upward and around the lower pole of the fetus.
Ancillary Forces in Labor
After the cervix is dilated fully, the most important force in fetal expulsion is that produced by maternal intraabdominal pressure. Contraction of the abdominal muscles simultaneously with forced respiratory efforts with the glottis closed is referred to as pushing. The force is similar to that with defecation, but the intensity usually is much greater. The importance of intraabdominal pressure is shown by the prolonged descent during labor in paraplegic women and in those with a dense epidural block. And, although increased intraabdominal pressure is necessary to complete second-stage labor, pushing accomplishes little in the first stage. It exhausts the mother, and its associated increased intrauterine pressures may be harmful to the fetus.
Cervical Changes
As the result of contraction forces, two fundamental changes—effacement and dilatation—occur in the already-ripened cervix. For an average-sized fetal head to pass through the cervix, its canal must dilate to a diameter of approximately 10 cm. At this time, the cervix is said to be completely or fully dilated. Although there may be no fetal descent during cervical effacement, most commonly the presenting fetal part descends somewhat as the cervix dilates. During second-stage labor in nulliparas, the presenting part typically descends slowly and steadily. In multiparas, however, particularly those of high parity, descent may be rapid.
Cervical effacement is “obliteration” or “taking up” of the cervix. It is manifest clinically by shortening of the cervical canal from a length of approximately 2 cm to a mere circular orifice with almost paper-thin edges. The muscular fibers at the level of the internal cervical os are pulled upward, or “taken up,” into the lower uterine segment. The condition of the external os remains temporarily unchanged (Fig. 21-6).
FIGURE 21-6 Schematic showing effacement and dilatation. A. Before labor, the primigravid cervix is long and undilated in contrast to that of the multipara, which has dilatation of the internal and external os. B. As effacement begins, the multiparous cervix shows dilatation and funneling of the internal os. This is less apparent in the primigravid cervix. C. As complete effacement is achieved in the primigravid cervix, dilation is minimal. The reverse is true in the multipara.
Effacement may be compared to a funneling process in which the whole length of a narrow cylinder is converted into a very obtuse, flaring funnel with a small circular opening. Because of increased myometrial activity during uterine preparedness for labor, appreciable effacement of a softened cervix sometimes is accomplished before active labor begins. Effacement causes expulsion of the mucous plug as the cervical canal is shortened.
Because the lower segment and cervix have lesser resistance during a contraction, a centrifugal pull is exerted on the cervix and creates cervical dilatation (Fig. 21-7). As uterine contractions cause pressure on the membranes, the hydrostatic action of the amnionic sac in turn dilates the cervical canal like a wedge. In the absence of intact membranes, the pressure of the presenting fetal part against the cervix and lower uterine segment is similarly effective. Early rupture of the membranes does not retard cervical dilatation so long as the presenting fetal part is positioned to exert pressure against the cervix and lower segment. The process of cervical effacement and dilatation causes formation of the forebag of amnionic fluid. This is the leading portion of fluid and amnionic sac located in front of the presenting part.
FIGURE 21-7 Hydrostatic action of membranes in effecting cervical effacement and dilatation. With labor progression, note the changing relations of the internal and external os in (A), (B), and (C). Although not shown in this diagram, with membrane rupture, the presenting part, applied to the cervix and the forming lower uterine segment, acts similarly.
Referring back to Figure 21-2, recall that cervical dilatation is divided into latent and active phases. The active phase is subdivided further into the acceleration phase, the phase of maximum slope, and the deceleration phase (Friedman, 1978). The duration of the latent phase is more variable and sensitive to changes by extraneous factors. For example, sedation may prolong the latent phase, and myometrial stimulation shortens it. The latent phase duration has little bearing on the subsequent course of labor, whereas the characteristics of the accelerated phase are usually predictive of labor outcome. Completion of cervical dilatation during the active phase is accomplished by cervical retraction about the presenting part. The first stage ends when cervical dilatation is complete. Once the second stage commences, only progressive descent of the presenting part will foretell further progress.
Second Stage of Labor: Fetal Descent
In many nulliparas, engagement of the head is accomplished before labor begins. That said, the head may not descend further until late in labor. In the descent pattern of normal labor, a typical hyperbolic curve is formed when the station of the fetal head is plotted as a function of labor duration. Station describes descent of the fetal biparietal diameter in relation to a line drawn between maternal ischial spines (Chap. 22, p. 449). Active descent usually takes place after dilatation has progressed for some time (Fig. 21-8). In nulliparas, increased rates of descent are observed ordinarily during cervical dilatation phase of maximum slope. At this time, the speed of descent is also maximal and is maintained until the presenting part reaches the perineal floor (Friedman, 1978).
FIGURE 21-8 Labor course divided on the basis of expected evolution of the dilatation and descent curves into three functional divisions. The preparatory division includes the latent and acceleration phases. The dilatational division is the phase of maximum slope of dilatation. The pelvic division encompasses both the deceleration phase and the second stage, which is concurrent with the phase of maximum slope of fetal descent. (Redrawn from Friedman, 1978.)
Pelvic Floor Changes During Labor
The birth canal is supported and is functionally closed by several layers of tissues that together form the pelvic floor. These anatomical structures are shown in detail in Chapter 2 (p. 22). The most important are the levator ani muscle and the fibromuscular connective tissue covering its upper and lower surfaces. There are marked changes in the biomechanical properties of these structures and of the vaginal wall during parturition. These result from altered extracellular matrix structure or composition (Lowder, 2007; Rahn, 2008). The levator ani consists of the pubovisceral, puborectalis, and iliococcygeus muscles, which close the lower end of the pelvic cavity as a diaphragm. Thereby, a concave upper and a convex lower surface are presented. The posterior and lateral portions of the pelvic floor, which are not spanned by the levator ani, are occupied bilaterally by the piriformis and coccygeus muscles.
The levator ani muscle varies in thickness from 3 to 5 mm, although its margins encircling the rectum and vagina are somewhat thicker. During pregnancy, the levator ani usually undergoes hypertrophy, forming a thick band that extends backward from the pubis and encircles the vagina about 2 cm above the plane of the hymen. On contraction, the levator ani draws both the rectum and the vagina forward and upward in the direction of the symphysis pubis and thereby acts to close the vagina.
In the first stage of labor, the membranes, when intact, and the fetal presenting part serve to dilate the upper vagina. The most marked change consists of stretching of the levator ani muscle fibers. This is accompanied by thinning of the central portion of the perineum, which becomes transformed from a wedge-shaped, 5-cm-thick tissue mass to a thin, almost transparent membranous structure less than 1 cm thick. When the perineum is distended maximally, the anus becomes markedly dilated and presents an opening that varies from 2 to 3 cm in diameter and through which the anterior wall of the rectum bulges.
Third Stage of Labor: Delivery of Placenta and Membranes
This stage begins immediately after fetal delivery and involves separation and expulsion of the placenta and membranes. As the neonate is born, the uterus spontaneously contracts around its diminishing contents. Normally, by the time the newborn is completely delivered, the uterine cavity is nearly obliterated. The organ consists of an almost solid mass of muscle, several centimeters thick, above the thinner lower segment. The uterine fundus now lies just below the level of the umbilicus.
This sudden diminution in uterine size is inevitably accompanied by a decrease in the area of the placental implantation site (Fig. 21-9). For the placenta to accommodate itself to this reduced area, it increases in thickness, but because of limited placental elasticity, it is forced to buckle. The resulting tension pulls the weakest layer—decidua spongiosa—from that site. Thus, placental separation follows the disproportion created between the relatively unchanged placental size and the reduced size of the implantation site.
FIGURE 21-9 Diminution in size of the placental site after birth of the infant. A. Spatial relations before birth. B. Placental spatial relations after birth.
Cleavage of the placenta is aided greatly by the loose structure of the spongy decidua, which may be likened to the row of perforations between postage stamps. As separation proceeds, a hematoma forms between the separating placenta/decidua and the decidua that remains attached to the myometrium. The hematoma is usually the result rather than the cause of the separation, because in some cases bleeding is negligible.
Fetal Membrane Separation and Placental Extrusion. The great decrease in uterine cavity surface area simultaneously throws the fetal membranes—the amniochorion and the parietal decidua—into innumerable folds (Fig. 21-10). Membranes usually remain in situ until placental separation is nearly completed. These are then peeled off the uterine wall, partly by further contraction of the myometrium and partly by traction that is exerted by the separated placenta.
FIGURE 21-10 Postpartum, membranes are thrown up into folds as the uterine cavity decreases in size. (Photograph contributed by Dr. Kelley S. Carrick.)
After the placenta has separated, it may be expelled by increased abdominal pressure. Completion of the third stage is also accomplished by alternately compressing and elevating the fundus, while exerting minimal traction on the umbilical cord (Fig. 27-12, p. 546). The retroplacental hematoma either follows the placenta or is found within the inverted sac formed by the membranes. In this process, known as the Schultze mechanism of placental expulsion, blood from the placental site pours into the membrane sac and does not escape externally until after extrusion of the placenta. In the other form of placental extrusion, known as the Duncan mechanism, the placenta separates first at the periphery and blood collects between the membranes and the uterine wall and escapes from the vagina. In this circumstance, the placenta descends sideways, and its maternal surface appears first.
Phase 4 of Parturition: The Puerperium
Immediately and for about an hour or so after delivery, the myometrium remains in a state of rigid and persistent contraction and retraction. This directly compresses large uterine vessels and allows thrombosis of their lumens to prevent hemorrhage (Fig. 2-11, p. 27). This is typically augmented by uterotonics (Chap. 27, p. 547).
Uterine involution and cervical repair, both remodeling processes that restore these organs to the nonpregnant state, follow in a timely fashion. These protect the reproductive tract from invasion by commensal microorganisms and restore endometrial responsiveness to normal hormonal cyclicity.
During the early puerperium, there is onset of lactogenesis and milk let-down in mammary glands, as described in Chapter 36 (p. 672). Reinstitution of ovulation signals preparation for the next pregnancy. This generally occurs within 4 to 6 weeks after birth, but it is dependent on the duration of breast feeding and lactation-induced, prolactin-mediated anovulation and amenorrhea.
PHYSIOLOGICAL AND BIOCHEMICAL PROCESSES REGULATING PARTURITION
There are two general contemporaneous theorems concerning labor initiation. Viewed simplistically, the first is the functional loss of pregnancy maintenance factors, whereas the second focuses on synthesis of factors that induce parturition. Some investigators also speculate that the mature fetus is the source of the initial signal for parturition commencement. Others suggest that one or more uterotonins, produced in increased amounts, or an increased population of myometrial uterotonin receptors is the proximate cause. Indeed, an obligatory role for one or more uterotonins is included in most parturition theories, as either a primary or a secondary phenomenon in the final events of childbirth. Both rely on careful regulation of smooth muscle contraction.
Myometrial Action
Anatomical and Physiological Considerations
There are unique characteristics of smooth muscle, including myometrium, compared with those of skeletal muscle that may confer advantages for uterine contraction efficiency and fetal delivery. First, the degree of smooth-muscle cell shortening with contractions may be one order of magnitude greater than that attained in striated muscle cells. Second, forces can be exerted in smooth muscle cells in multiple directions. In contrast, the contraction force generated by skeletal muscle is always aligned with the axis of the muscle fibers. Third, smooth muscle is not organized in the same manner as skeletal muscle. In myometrium, the thick and thin filaments are found in long, random bundles throughout the cells. This plexiform arrangement aids greater shortening and force-generating capacity. Last, greater multidirectional force generation in the uterine fundus compared with that of the lower uterine segment permits versatility in expulsive force directionality. These forces thus can be brought to bear irrespective of the fetal lie or presentation.
Regulation of Myometrial Contraction and Relaxation
Myometrial contraction is controlled by transcription of key genes, which produce proteins that repress or enhance cellular contractility. These proteins function to: (1) enhance the interactions between the actin and myosin proteins that cause muscle contraction, (2) increase excitability of individual myometrial cells, and (3) promote intracellular cross talk that allows development of synchronous contractions.
Actin-Myosin Interactions. The interaction of myosin and actin is essential to muscle contraction. This interaction requires that actin be converted from a globular to a filamentous form. Moreover, actin must be attached to the cytoskeleton at focal points in the cell membrane to allow development of tension (Fig. 21-11). Actin must partner with myosin, which is composed of multiple light and heavy chains. The interaction of myosin and actin activates adenosine triphosphatase (ATPase), hydrolyzes adenosine triphosphate, and generates force. This interaction is brought about by enzymatic phosphorylation of the 20-kDa light chain of myosin (Stull, 1988, 1998). This is catalyzed by the enzyme myosin light-chain kinase, which is activated by calcium. Calcium binds to calmodulin, a calcium-binding regulatory protein, which in turn binds to and activates myosin light-chain kinase.
FIGURE 21-11 Uterine myocyte relaxation and contraction. A. Uterine relaxation is maintained by factors that increase myocyte cyclic adenosine monophosphate (cAMP). This activates protein kinase A (PKA) to promote phosphodiesterase activity with dephosphorylation of myosin light-chain kinase (MLCK). There are also processes that serve to maintain actin in a globular form, and thus to prevent fibril formation necessary for contractions. B. Uterine contractions result from reversal of these sequences. Actin now assumes a fibrillar form, and calcium enters the cell to combine with calmodulin to form complexes. These complexes activate MLCK to bring about phosphorylation of the myosin light chains. This generates ATPase activity to cause sliding of myosin over the actin fibrils, which is a uterine contractor. AC = adenylyl cyclase; Ca++ = calcium; DAG = diacylglycerol; Gs and Gα = G-receptor proteins; IP3 = inositol triphosphate; LC20 = light chain 20; PIP3 = phosphatidylinositol 3,4,5–triphosphate; PLC = phospholipase C; R-PKA = inactive protein kinase. (Redrawn from Smith, 2007.)
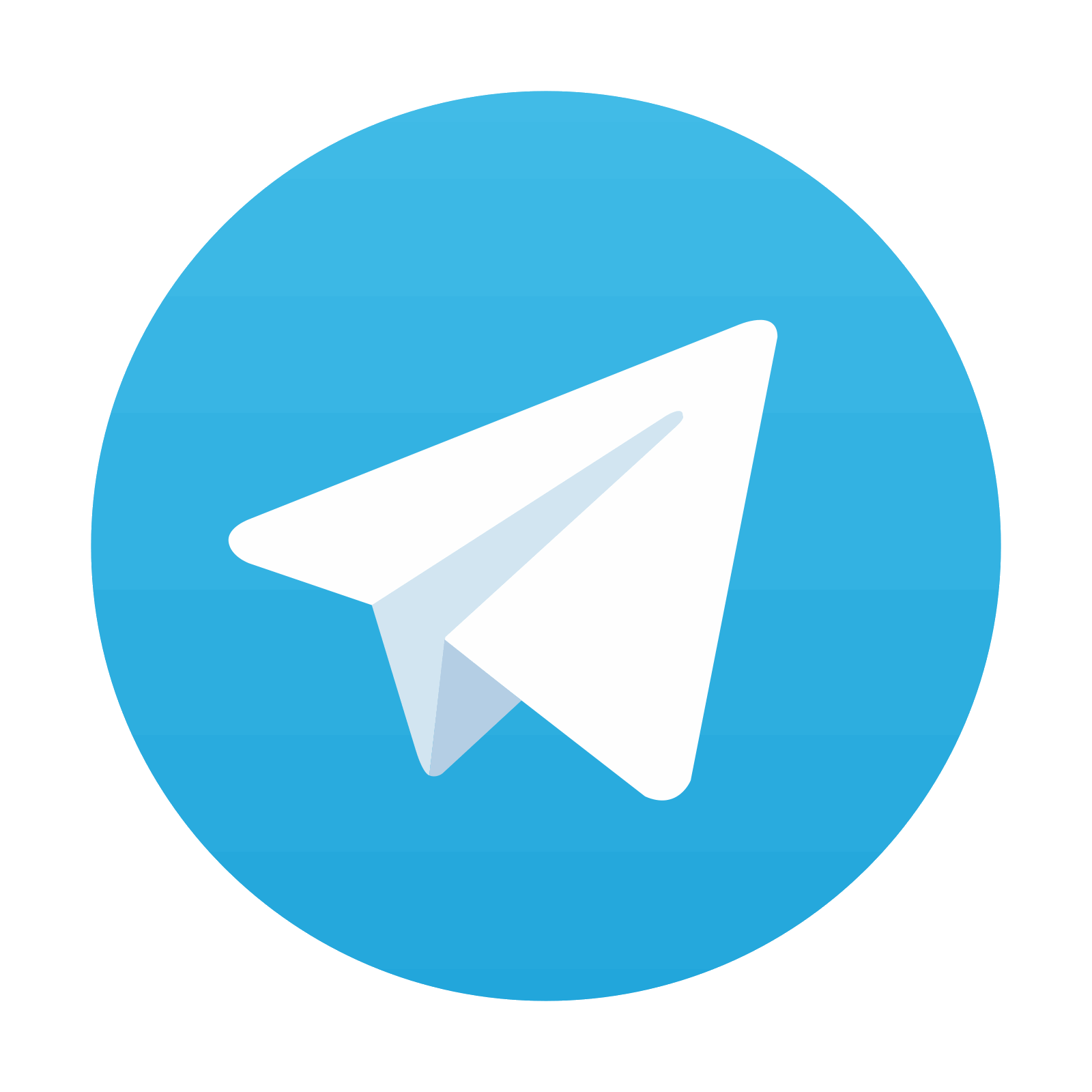
Stay updated, free articles. Join our Telegram channel
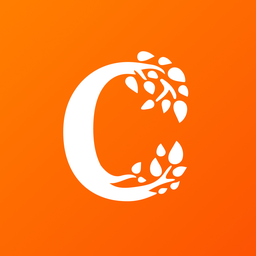
Full access? Get Clinical Tree
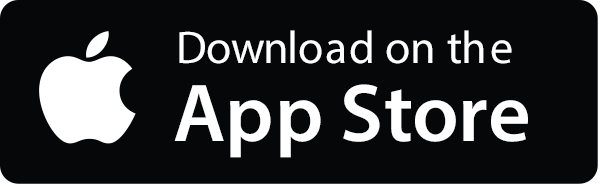
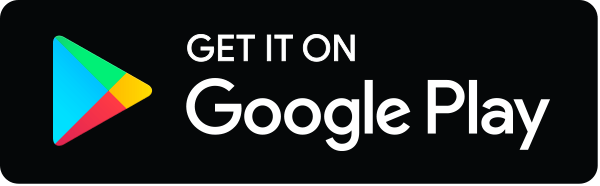