Introduction
The application of the theories, principles and methods of physics to medicine and healthcare, in general, belongs to a branch of medicine called ‘medical physics’. This chapter focuses on the use of medical physics in obstetrics and gynaecology, with a focus also on its surgical application.
Physics for Surgery
A trainee will encounter plenty of situations where the principles of physics are integral to the surgical learning experience. Summarised below are the most relevant, but, first, some basic physics and formulae.
Tissues are composed of cells, which are, in turn, composed of atoms. Electrons orbit the nucleus of an atom (composed of a neutron and proton) and bring about current flow when they move from one atom into an orbit of another atom. The flow is generated by a force that comes from a voltage which is, in turn, generated by a power generator.
Ohm’s law, in simple terms, defines the current (electrons flowing through an electrical conductor, tissue or space during a period of time; unit – ampere ) passing between two points as being directly proportional to the voltage (or the potential difference; work needed per unit of charge to move a test charge between two points; unit – volt ) between the exact same two points. Resistance is the constant of that proportionality and the hindrance to the flow of current ( unit – ohm ).
The formula that binds all three together is: Current ( I ) = Voltage ( V )/Resistance ( R ). In addition, Power ( W ) = Current ( I ) × Voltage ( V ).
Electrosurgery
Electrosurgery (ES) is defined as the application of a high (radio) frequency electrical current during surgical operations on biological/human tissue. The concept was first initiated in the 20th century, by the father of neurosurgery, Harvey Cushing and a physicist, William Bovie. Four modes have been defined: cutting , coagulation , desiccation and fulguration .
All the modes rely on the application of an alternating electrical current, pioneered by the famous Serbian physicist, Nikola Tesla, onto tissue, which, in turn, generates heat. This basic principle has resulted in turning gynaecology into a full-blown surgical speciality. It involves improved, efficient procedures being performed to treat both benign and malignant conditions with less complications. Procedures include minimally invasive operations such as hysteroscopies and laparoscopies, open surgeries such as laparotomies (pelvic-focused and multivisceral resections), vulval/vaginal surgeries and treatments for cervical pathologies.
Equipment
In the operating room, the source of the voltage, electron flow, and, hence, electric current is the ES generator. The current then arrives to the instrument itself – in fact, the tip of the instrument –, which acts as the active electrode. The current subsequently returns to the generator via the patient and a different electrode, known as the patient return electrode. Therefore, the circuit consists of the following (in order): ES generator (or ES unit), active electrode, patient and patient return electrode.
Tissue acts as a conductor for the current, albeit a poor one, via interstitial fluid. During conduction, current meets resistance, resulting in generation of heat within the tissues, as the electrons overcome the resistance. To ensure that only required heat is produced, the generated voltage is modified by an ES generator. The modification usually depends on the variable tissue resistance.
An example is Faradic effect. Sixty kilohertz is the standard frequency at which an electric current alternates (examples include kitchen electrical appliances). An alternating current allows for modification of generated voltage and, hence, input frequencies to be increased to more than 400 kHz. This increase acts as a protection mechanism by reducing muscle and nerve stimulation. The stimulation is known as Faradic effect, occurring at frequencies below 100 kHz. Therefore, at much higher frequencies, electricity can pass through a patient with no risk of injury secondary to electrocution and minimal neuromuscular stimulation.
Modes
When we talk about heat, what do we actually mean? As explained above, the tissue radio frequency alternating current is the key. It is the radio frequency that causes molecules within cells to oscillate. This, in turn, increases the temperature within a cell, leading to heat generation.
At 60°C, the cell dies instantly. Between 60°C and 100°C, the tissue is completely dried out (dehydrated or desiccated) and simultaneously coagulated (non-liquid contents of the cell, i.e. protein). Above 100°C, intracellular contents turn to gas, the tissue expands in volume exponentially, and, consequently, it aggressively vaporises.
So, let us go through the four modes of ES (cut, coagulate, desiccate and fulgurate). At a high power, with a high frequency and constant waveform current, the water content of cells (and, therefore, tissue) is vapourised upon direct electrode contact. This allows for tissue to be cut (rapid increase to 100°C). Intermittent and, thus, lower power waveforms are used to coagulate tissue. Because of the lower power, the heat here is insufficient to bring about explosive vaporisation but, instead, results in occlusion of blood vessels and haemostasis. The area produced is known as a thermal coagulum.
Desiccation, like cutting, leads to vaporisation of the liquid cellular content. However, this cellular ‘drying’ here occurs with lower power and, hence, lower heat than required with cutting. Therefore, the exposed tissue on which the instrument electrode is placed dries out but only the surface and area directly underneath the surface. The damage caused to tissue with desiccation is significantly less than with cutting and coagulation.
In complete contrast to the above, fulguration occurs when the electrode is held away from the tissue in question. The result is similar to coagulation but in this case, only superficial. High voltage modulated waveforms bring about ionisation of the air gap between the electrode and the tissue. This results in an electric arc which superficially coagulates the tissue. This coagulation is superficial but spread to an area larger than the electrode tip.
It is worth highlighting that the above differs from electrocautery, which describes heat conduction by the application of an instrument heated via direct electric current to a high temperature onto tissue. This is what leads to cautery. Therefore, the current does not enter the patient’s body. In ES, the current passes through the patient’s tissue or body and, therefore, the patient becomes part of the current.
Monopolar and Bipolar Diathermy
Diathermy is an obvious and most common example of ES in the operating field. Diathermy relates to the instrument used. Two types exist: monopolar (single electrode) and bipolar (two electrodes); monopolar diathermy is the more common of the two.
The monopolar instrument itself acts as the ‘active electrode’ and is in the surgical site when galvanised. Therefore, the patient return electrode is on a different part of the patient’s body. It acts as a ‘dispersive electrode’, which scatters the current that has passed through the patient. This closes the circuit but, importantly, prevents thermal injury to the underlying tissue.
An example of a bipolar diathermy instrument is forceps used to coagulate blood vessels. It includes two electrodes, both an active and a return electrode, whose functions are, therefore, carried out at the surgical site. This means that the only part of the patient that is included in the circuit is the tissue grasped between the electrodes. This is the main advantage of bipolar instruments: elimination of the risk of current diversion, a need for a patient return electrode and other risks. The main disadvantage is that it is difficult to vaporise or cut tissue with bipolar instruments.
The effect of heat on tissue when using either monopolar or bipolar diathermy will very much depend on the following variables: surface area, duration and proximity of instrument to tissue, as well as tissue conductivity. An increase in the latter three means more heat generated, but an increase in surface area results in a lower current concentration and less heat.
Thunderbeat, LigaSure and Harmonic
Integrated energy sources have vastly changed the landscape of minimally invasive surgery. They combine the ability to dissect tissues whilst simultaneously attaining haemostasis. Three are described here.
Thunderbeat (TB) is the only integrated form of surgical energy derived from a single instrument. It concurrently delivers ultrasonically generated frictional heat energy and electrically generated bipolar energy. It can realise 7-mm vessel sealing. Five variables determine the versality of TB: haemostasis, histologic sealing, cutting, dissection and tissue manipulation.
LigaSure vessel sealing system (LVSS) is a bipolar device for sealing vascular tissue. It seals tissue by administration of high current and low voltage as compared to conventional ES. It provides a secure seal of blood vessels measuring up to 7 mm diameter.
Harmonic scalpel (HS) is a high-power system that works at a frequency of 55.5 kHz or 55,500 vibrations/s.
TB and HS apply ultrasonic dissection by applying ultracision. The handpiece contains a transducer ‘comprised’ of piezoelectric crystals sandwiched among metal cylinders. The ultrasound (US) generator transforms ultrasonic energy into mechanical energy. Ultimately, vessels are sealed because of denatured protein coagulum secondary to tamponade and coaptation.
TB differs in many ways from LVSS and HS. The versatile score of TB is higher than LVSS and HS. This is demonstrated in many ways. First, the dissection time with TB is shorter, ensuring a faster operation time, reliable 7 mm vessel sealing, accurate dissection with fine jaw design, inconsequential thermal spread and best visibility at time of surgery. Second, TB has higher bursting pressure and highly reduced thermal speed compared to LVSS and HS.
Complications
A quick word about potential complications from using ES in the context of laparoscopy. Injuries, in general, are similar to those during open surgery, for example, laparotomy, and can be attributed to inappropriate identification of anatomical structures, mechanical trauma or electrothermal injuries. Our focus here is on electrothermal injuries. They can be grouped into four areas: (1) direct application; (2) insulation failure, (3) direct and capacitive coupling of current; and (4) return electrode or alternative site burns.
Direct application causes injury either via unintentional activation or incorrect targeting. Both are commonly attributed to momentary lack of attention and increased speed of the procedure meaning more coagulation and thermal spread. Dwell time is also important as it determines the amount of tissue effect. Prolonged or unintended activation means increased involuntary dwell time, hence wider and deeper tissue damage.
Poor insulation means, in simple terms, a stray current escaping to injure bowel, bladder ureters or blood vessels. Attention to detail is paramount here, both pre- and post-operatively, to check the actual instruments for defective insulation. Frequent sterilisation, to ensure asepsis, leads to a weakening of insulation, as does repeated use of disposable equipment and a mismatch in size between an instrument and port size (‘wear and tear’).
Direct coupling occurs when the surgeon accidentally activates the active electrode when it is near to another metal instrument (e.g. laparoscope or metal grasper forceps). The metal instrument becomes energised, and, hence, the current will look to complete the circuit to the patient return electrode. Prevention of this involves visualising the active electrode and ensuring no contact with any other conductive instrument before its activation.
Coupling can be also indirect or capacitive . The process is as follows: electric current is transferred from the active electrode, that is, conductor, through its surrounding, unbroken insulation, into adjacent conductive tissue (e.g. bladder or bowel) without actual direct contact. Therefore, to prevent this, the surgeon must activate the active electrode only when it is in contact with target tissues. Additionally, ensuring appropriate and fail-proof insulation, shorter instrument length, and reduction in high-voltage peaks are beneficial.
The grounding (dispersive) pad offers the path of least resistance from the patient back to the generator and ensures an area of flow current density. If the return electrode is not able to disperse the current safely or is not completely in contact with the patient’s skin, it results in the exiting current having a high enough density to produce an unintended burn. It is important to have good contact between the patient and a dispersive pad. A burn at an alternative site can occur if the dispersive (ground) pad is not well connected to the patient’s skin. When the dispersive pad lacks in quantity or quality, or patient interface, the electrical circuit can be completed by a small grounded contact point, such as electrocardiogram leads and produce high current densities, causing a burn.
Laser
The effectiveness of Light Amplification by Stimulated Emission of Radiation (LASER) is derived from the production of a perfectly parallel beam, allowing extremely tight focusing. Concentrating energy into a very small area in a very brief pulse produces very high local temperatures, sufficient to vaporise tissue, surrounded very locally by a cauterising action, which seals the edges and reduces blood loss.
In the following description, the simplified Bohr model of the atom is used, modified where relevant by some of the constraints of wave mechanics. Neodymium-doped yttrium aluminium garnet (Nd-YAG) lasers are rod lasers that are pumped by a flash lamp, having a broad spectrum of light. A typical system is shown in Fig. 13.1 . The flash excites electrons orbiting a nucleus to orbits of higher energy, from where they drop back and may emit a photon of light as they do. In suitable materials, some of these orbits are relatively stable, and electrons tend to dwell in them for a relatively long time. The rod has polished, squared off ends and is placed between mirrors with surfaces that are exactly parallel, so that any light emitted within the rod is reflected back and forth. Those electrons in unstable orbits soon drop back at random times and take no significant part in the true laser beam. Those in semi-stable orbits accumulate. When they drop back, they also emit a photon, although most are not parallel to the axis of the rod and mirrors. When a photon has a wavefront parallel to the mirrors, it is reflected exactly back to the other mirror, which sends it back to the first, etc. Because its wavelength is exactly the same as that represented by the energy difference in all the similar semi-stable electrons, they are stimulated to join in as the wave passes by, adding their energy to the beam in precisely the same direction and phase. The stronger the beam becomes, the more likely any remaining excited electrons are to join in, producing a sudden, very intense, flash of visible or infrared light. This is how lasers get their name: LASER.

Part of the light is allowed to escape through one of the mirrors, and it is described as ‘coherent’. Coherent light is monochromatic and highly parallel, sharing the same spatial and temporal phase. Because it is so perfectly parallel, it can be focused onto much smaller spots than sunlight. Spots of 0.5 µm can be used to cut into single cells in vitro. Although the conversion efficiency from energy in the flash tubes to laser output is very small, typically less than 1/1000, this fine focusing produces very high local energy deposition, termed irradiance. For instance, a typical CO 2 laser might briefly produce an irradiance of up to 20 kW/cm 2 over a 0.3-mm diameter spot. The wavelength of the radiation (colour, if in the visible range) depends on the material doing the lasering. This has an important bearing on the effect on the tissue. CO 2 lasers produce infrared radiation at a 10.6 μm wavelength, which is strongly absorbed by water in the tissue. Tightly focused intensities of 100 W/cm 2 produce tissue vaporisation depths of 3 to 4 mm, giving the effect of a laser knife. A laser knife has the further advantage that, at the edge of its beam, blood vessels become coagulated, sealing off the operative field as they cut. Cone excisions from the cervix may be produced by mechanical rotation of the beam. Spreading the beam by partial de-focusing is useful for surface ablation or thermocoagulation.
The physical properties of CO 2 lasers make them unsuitable for operating on thicker tissues, for example to ablate endometriosis. Here, the Nd-YAG laser is ideal because its wavelength is in the near infrared range (0.532 μm), at which water is transparent, but blood pigments readily absorb. The radiation is conducted to the site via a 0.4-mm diameter fibre light guide. This is a fine glass fibre whose outer surface is coated with a thin layer of another glass of a different refractive index. This ensures that any light not quite parallel to the axis of the fibre is totally reflected back into the fibre as if the fibre were surrounded by a perfect mirror. The fibre is flexible. It can then be passed down endoscopes and positioned using a pilot beam of normal light. When the desired position is attained, and the trigger button pressed, a shutter blocks the eyepiece, the flash lamp fires and the shutter re-opens. It is likely that a whole range of different laser beams will become available, each tailored to a specific surgical application.
Imaging
Computed Tomography
Computed tomography (CT) represents an accurate and fast medical imaging technique. Whole body images are currently obtained using continuous, helical data acquisition instead of sequential and axial (transverse anatomical plane, which is perpendicular to the body). Hence, these images are taken by rotation around a fixed axis.
A single slice can be 1 to 5 mm thick and contains the attenuation of different tissues to ionising radiation. A mean value for a given volume of tissue is calculated and is known as a voxel. Furthermore, a voxel is represented in greyscale as a single point in the final 2D image. It is commonly referred to as a pixel. The greyscale of the image is measured using the Hounsfield scale, which is relative to the attenuation of water (water is 0 Hounsfield units). Air measures −1000 Hounsfield units and metal above a 1000. Common CT techniques include CT angiography, CT urography, perfusion CT and CT combined with positron emission tomography (PET).
With regards to the latter, it has become a key investigatory tool in oncology, both for diagnosis and follow-up. A gantry holds the CT and PET scanners together. The sequential images acquired from both devices are combined into a single superimposed image. PET images are functional, mapping glucose metabolism in the body. This is possible due to the uptake (by metabolically active tissues) of 18 F-fluorodeoxyglucose (FDG), a glucose analogue with a half-life of 2 hours. Its decay results in the release of a positron, whose positive electric charge, makes it seek an electron. It is this collision with an electron that brings about a release of high energy photons. They are subsequently detected by PET. In combination with CT images, one can see how the aforementioned activity correlates with anatomical imaging acquired by CT scanning. This allows for identification of hot spots, that is, primary carcinosis or metastases, where uptake of FDG is high.
Magnetic Resonance Imaging
Magnetic Resonance Imaging (MRI) is a type of non-ionising radiation. It used to be called ‘nuclear magnetic resonance’, but that had negative associations in patients’ minds. No beams of light or sound are produced; instead, the nuclei of certain atoms in the patient are induced to report their presence and condition by absorbing or sending out radio waves. Th SI for a magnetic field is a Tesla. A magnetic field of 0.5 to 3 T is produced by medical MRI magnetic equipment. This is a type of electromagnetic radiation that does not carry enough energy per quantum to ionise atoms or molecules. Images of body organs are generated by magnetic fields and their gradients and radio waves. The principle is based on fat and water (which, together, make up ~80% of the human body) containing a high number of hydrogen nuclei (unpaired protons).
These hydrogen nuclei have a positively charged spin that gives it magnetic polarity. When spinning within an external magnetic field, they are able to produce or absorb radio waves, manifested as radio frequency energy. Hence, the frequency is dependent on the electromagnetic field and the energy state of the radio waves. Moreover, the electro-magnetic field aligns atomic (hydrogen) nuclei within the human body, an act that allows for the electromagnetic field to become rotating. Using resonant frequency, the radio frequency pulse flips nuclei away from their original alignment via an angle depending on the amount of energy they absorb. Upon cessation of the electromagnetic field, the radio frequency pulse stops, and the nuclei relax and flip back to their original alignment. An MRI machine will detect a photon, released as a difference in energy between the two states that the nuclei find themselves in. To get a picture of the internal abdominal contents, the difference in energy released is measured according to location.
The correct terminology for the greyscale seen on images is signal intensity. High signal is white and low signal is black. The time between the radio frequency pulses and the time between a radio frequency pulse and an ECHO determine weighting. Weighting is commonly divided into two groups for the purposes of MRI: T1-weighted (focus is on anatomy) and T2-weighted (focus is on pathology). T1 refers to spin-lattice relaxation time, which is the time taken for the nuclei to revert to their original energy state once the field is turned off (dissipating energy previously absorbed). In the images produced, fluid-containing tissues are dark and fat-containing tissues are bright. With the radiofrequency field off, the nuclei eventually lose their alignment, with a loss of synchronisation. As opposed to spin-lattice relaxation time (T1), this is known as spin-spin relaxation time (T2). The opposite is the case here; water and fluid-containing tissues are bright and fat-containing tissues are dark.
Ionising Radiation
Radiation Defined
First, we define radiation. The term ‘radioactivity’ refers to occurrences in the nuclei of atoms. The nucleus is the positively charged centre around which the negatively charged electrons of the atom circulate in orbits up to 10,000 times the nuclear diameter. There is continual exchange of energy between particles constituting the nucleus, and, in some atoms, it is possible for sufficient energy to be acquired by a particle to allow it to escape ( Fig. 13.2 ).
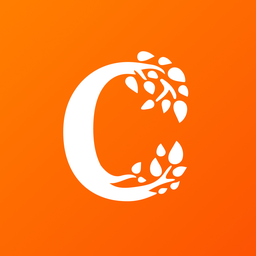
Full access? Get Clinical Tree
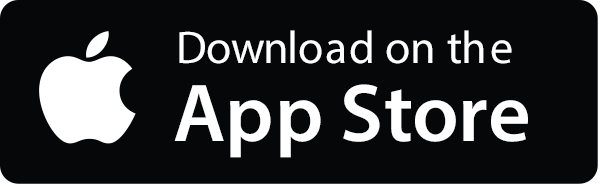
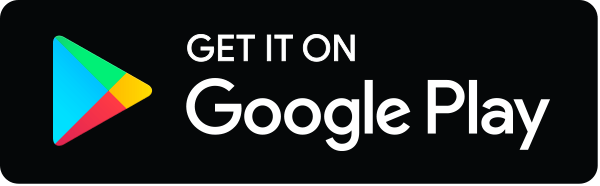