Fig. 8.1
Changes to motor evoked potentials (MEPs) during corpus callosotomy following placement of interhemispheric retractor resulting in loss of MEPs to lower extremities (arrow). Note changes recorded primarily from lower extremity myotomes. Following immediate discussion with surgical and anesthesia teams, retractors were repositioned with subsequent rapid recovery of all motor evoked potentials. Timebase 10 ms/division, amplitudes varying with no less than 100 μV/division, RtCtxMEP stimulation of right cortical hemisphere, LEC left extensor carpi radialis muscle, LFD left first dorsal interosseous muscle, LQD left quadriceps muscle, LTA left tibialis anterior muscle, LAH left abductor hallucis muscle, RFD right first dorsal interosseous muscle, RTA right tibialis anterior muscle (right-sided myotomes utilized to assess extent of contralateral current spread)
Once the neurophysiology team has observed changes, these should be communicated immediately to the surgical and anesthetic teams so that they may respond appropriately to the signal changes. The patient’s hemodynamic parameters and anesthetic agents may require rapid adjustments in response to a change or loss of signals, especially if the patient is in relative hypotension with subsequent hypoperfusion to the nervous system. Positioning should also be re-evaluated, especially in the setting of focal IONM changes. The surgeon should pause and consider any recent changes in the surgical field that can be reversed or mitigated to return to their prior state [29, 30]. This could include releasing distracting forces slowly during spinal procedures or removing clips during vascular procedures. Repeat evaluations and further modification of surgical and anesthetic techniques should continue until IONM signals have returned to their baseline; if it is felt the risk of proceeding is unacceptably high given the changes in monitoring signals, the procedure may need to be aborted until the patient is stabilized and evaluated post-operatively.
Cerebral Monitoring
The success of cortical monitoring in children is dependent on brain maturation and optimizing monitoring and stimulation parameters based on age and pathology. Long tract monitoring (SSEPs and tcMEPs) is the mainstay of cortical monitoring, with direct mapping (motor, somatosensory, and verbal) as powerful adjuncts for a select group of patients. Cortical regions associated with long tracts, including somatosensory and motor cortex, myelinate earlier—usually wholly so by 1 year of age—than other regions such as fronto-temporal cortex, and therefore can be monitored in very young patients [31].
Modalities such as SSEPs and tcMEPs allow for early detection of changes during positioning as well as intra-operatively for nearly all intracranial pathologies. The pediatric cranial IONM experience is most firmly established in epilepsy and tumor surgery, and SSEP/MEP-based IONM is recognized as a gold standard for these surgeries. The goal of this type of monitoring is to improve surgical safety by alerting the surgeon to early signs of ischemia and allow management changes ideally prior to permanent ischemic/hypoxic injury [32]. An important adjunct to traditional long tract monitoring within the brainstem is the use of BAERs to provide additional information on global cerebral changes such as temperature and ischemia [33]. Cranio-cervical junction pathology, such as Chiari malformations and skull base lesions, also benefits from monitoring to reduce the risk of injury to the junction while the head is fixed in the cranial frame and during decompression [34–36]. Outside of the realm of neurosurgery, cranial monitoring with SSEPs and electroencephalography (EEG) has been widely documented in cardiac surgery [37, 38]. Although changes from baseline trigger intra-operative interventions, evidence to support improved outcomes after pediatric cardiac surgery is still limited [38].
Cortical mapping has evolved into a standard technique for supratentorial pediatric surgery in regions of eloquent cortex—the most common form being direct motor cortex mapping. Traditionally, motor mapping has been performed via a bipolar handheld probe with low frequency sustained stimulation, known as the Penfield technique since its development in the 1930s [4, 39]. Monopolar electrical stimulation can also be reliably used to map primary motor cortex [40]. Its thresholds in pediatric cortical mapping may be more consistent in certain areas of motor cortex such as the upper extremities compared to face and lower extremities [41]. Cortical lesions including those found in tuberous sclerosis or cortical dysplasia can also alter monitoring considerations and exhibit higher stimulation thresholds [41].
Surgeons can more precisely delineate eloquent territories intraoperatively based on function rather than imaging alone, and thus guide their cortical entry point and subcortical path to the targeted lesion [4, 41, 42]. Direct identification of primary somatosensory cortex as well as probable location of the central sulcus utilizes recording the location of the SSEP phase reversal to contralateral peripheral nerve stimulation [43, 44] (Fig. 8.2). This technique often employs a strip electrode containing at least two and often four or more electrodes in a straight line placed perpendicular to the orientation of the putative central sulcus straddling both the primary motor and primary sensory cortices. Typically, half the electrodes on the strip are placed pre-centrally with remaining half post-centrally. The recorded SSEP will appear “in phase” when recorded over primary sensory cortex and appear “reversed” in phase when recorded from the electrode placed over the motor cortex leading to the technique being termed phase reversal.

Fig. 8.2
Somatosensory evoked potentials (SSEPs) with right ulnar nerve stimulation, recording electrodes 1 and 2 placed post-centrally, and recording electrodes 3 and 4 placed pre-centrally. Note that the fifth tracing represents a transcranial control electrode placed posterior to the sensory cortex to allow the neurophysiologist to rapidly identify the sensory side. A phase reversal was recorded between electrodes 2 and 3, confirming the locations of the physiologic motor and sensory cortical regions
Technical considerations to this technique should include possible distortion of parenchyma by a lesion. Distortion can affect both the amplitude and phase of the SSEP precluding strict identification of a phase reversal. In these scenarios, it’s important to recognize that the technique will most reliably identify primary somatosensory cortex even without a phase reversal. We routinely include a positive control transcranial recording of the expected SSEP when employing the phase reversal technique to guide “in phase” identification and therefore primary sensory cortex mediated responses. Thus, localization of the central sulcus, primary motor cortex, and primary somatosensory cortex is one of the primary indications for phase reversal and motor mapping. These techniques should be considered complementary and performed together in order to provide the most accurate mapping data to the surgeon.
Awake craniotomies have been adapted from adult neurosurgery to the pediatric population with awake mapping, and can allow for resection in eloquent territories previously deemed too risky for operative intervention. There is no established minimum age for participating in awake craniotomies, but a successful awake procedure hinges on the child’s cooperation. The patient must be able to cooperate while moderately sedated and secured on the operating table in an unfamiliar and overwhelming environment [4]. This requires a mature child or adolescent, without cognitive or developmental delays, and cooperation and consent from the parents to optimize pre-operative psychological patient preparation. Based on our institutional experience, we would suggest that mature pre-adolescents and adolescents may be candidates for awake monitoring. Younger children may not tolerate these procedures well and more likely to be unable to cooperate with testing. Cognitive, language, motor and somatosensory systems can be assessed via awake intraoperative mapping. Careful patient selection and precise neuroanesthesia are crucial in ensuring a calm, comfortable, and cooperative patient during this mapping process. Complications from awake mapping are both rare and similar to direct cortical mapping, including seizures, and additionally can include language or cognition-specific deficits such as transient dysphasias [45].
Spinal Cord Monitoring
Intraoperative neuromonitoring is especially important in pediatric spinal surgeries as they are associated with a higher risk of new neurologic deficits than in a similar adult population—1.3% vs 0.8%—and even more so in the setting of instrumentation [46, 47]. There is extensive literature on IONM in pediatric spinal deformity correction and its utility in detecting early neurological injuries, such that some advocate its use in every case [48]. Somatosensory and motor evoked potentials are regarded as the building blocks of successful neuromonitoring for spinal procedures—for both spine and nervous system procedures.
A multimodal approach with SSEPs, tcMEPs, and EMG can be 99.6% sensitive at detecting permanent neurological injuries during pediatric spinal procedures, even if lower thresholds increase the risk of false-positive monitoring alerts [49–51]. A base of SSEP and MEP monitoring can be effectively supplemented by indirect stimulation with transduced EMG during instrumentation placement, proven to be as safe as image-based navigation for instrumentation in pediatric deformity cases [52]. Alerts can lead to effective changes in surgical and anesthetic management in order to reduce the risk of permanent neurological deficits to 1% or less [50, 51]. Although changes in neuromonitoring are seen in up to 10% of spinal deformity cases, only 1% of pediatric patients will emerge with a permanent neurological deficit [53].
IONM is feasible in spine patients even at a very young age. In children less than 6 years old, MEP reliability in spine surgery only decreases to 86%, compared to 98% in older children and can still be safely and reliably utilized for very young patients [19, 54]. TcMEPs are exquisitely sensitive to altered spinal cord perfusion secondary to hypotension, ischemia or direct vascular injury, and can detect changes earlier than SSEPs in scoliosis surgery [55]. However if MEPs are not detectable or feasible in certain pediatric spine patients, SSEPs alone can safely be used for scoliosis surgery [56]. Somatosensory monitoring is flexible, and can be performed with epidural electrodes if needed [57]. If underlying diseases introduce risk factors that preclude reliable MEP evaluation and only SSEPs are utilized, it’s important to highlight that changes to both anterior spinal artery perfusion of the ventral two thirds of the spinal cord and the corticospinal motor tracts will not be detected. The use of somatosensory evoked potentials for spinal cord monitoring is not an adequate proxy for assessing the long tract motor pathways. Alternatively, preoperative neurophysiologic evaluation may help identify which patients could reasonably be monitored using combined MEPs and SSEPs [58].
Monitoring has become standard of care for surgery for intramedullary spinal cord lesions and has been recognized as a neurosurgical guideline [59]. Changes in IONM, especially SSEPs and tcMEPs, during resection of intramedullary spinal cord tumors can predict the severity of postoperative motor deficits in pediatric patients [60]. Though monitoring is widely utilized for intramedullary spinal cord operations, there is also evidence that it may mitigate the risk of neurological injury for intradural extramedullary tumor resection and syringomyelia procedures [61, 62]. Tethered cord procedures have also been improved by the addition of multimodal monitoring, including MEPs/SSEPs, as well as direct stimulation for tether and sacral root identification, and even reflex testing [63]. EMG has also been reported as a valuable supplemental modality for conus medullaris and cauda equina mapping in conjunction with MEPs/SSEPs [64].
Transcranial electrical stimulation activates fast conducting axons in descending motor pathways via both direct and indirect means. Direct activation can be recorded as a D-wave over the spinal cord utilizing either an epidural or subdural recording electrode, which is more reliable through all depths of anesthesia than its indirectly activated counterpart, the I-wave (Fig. 8.3). This technique, first described in 1954, can often be employed for spinal cord monitoring even when MEPs and SSEPs are not recordable and provides a robust general sense of spinal cord health intra-operatively [65]. It does not provide nearly the granularity of mapping compared to standard evoked potentials nor the sensitivity to cord ischemic insults of MEPs [66]. D-wave signals may become minimal below T11-12 when corticospinal motor fibers have already exited the cord towards the periphery, and may not be present at baseline with prior spinal cord pathology or secondary to age [66–68]. It is essentially absent in children younger than 21 months, and in older pediatric patients may only be present in 30–50% of patients, compared to up to 80% of adult patients with similar neurological status [66, 69]. If utilized, a 50% decrease from its baseline amplitude should be considered a critical threshold to alert the surgical and anesthetic teams in order to pause and alter management [66]. Perhaps the strongest case in adding D-wave to a multimodal IONM battery is that loss of the MEP during resection of intramedullary spinal cord lesions precedes loss of the D-wave. Finally, preservation of at least 50% of the D-wave supports a positive prognostic outcome for patients regaining motor function following surgery [67, 70].

Fig. 8.3
Recording of transcranial electrical stimulation. D-wave: timebase 2 ms/division, amplitude 5 μV/division. Left column represents right cortical stimulation and recording of left corticospinal tract D wave, right column represents left cortical stimulation and recording of right corticospinal tract D wave. First eight traces represent placement of subdural electrode rostral to the lesion and is considered a positive control recording normal D wave. Subsequent traces show recorded D wave following placement of subdural electrode caudal to lesion and throughout resection. Flat lines noted in the initial traces and again on eighth trace highlight unilateral stimulation. Remaining traces obtained via interleaved stimulation for lateral spinal cord specificity
Dorsal column mapping to identify the midline of the spinal cord can be utilized prior to performing a midline myelotomy for resection of an intramedullary lesion [71]. This is a general application of the phase reversal technique described above whereby instead of peripheral nerve stimulation, stimulation of the gracile fasciculus is performed directly, typically via placing a bipolar electrode over the spinal cord starting in a lateral position and walking the stimulation across midline. The neurophysiologist will subsequently record the phase reversal of the gracile fasciculus stimulated SSEP from the scalp and guide the surgeon in identifying the neurophysiological midline of the dorsal column of the spinal cord (Fig. 8.4).

Fig. 8.4
Dorsal column mapping: Phase reversal of scalp-recorded gracile-mediated SSEPs (Cp3–Cp4) responding to direct stimulation of the dorsal columns using a concentric bipolar electrode. Top to bottom: the first trial was performed on the left lateral dorsal column, the second trial was on the left medial dorsal column, the third trial was on the right medial dorsal column, and the fourth trial was on the right lateral dorsal column. Note the phase reversal to gracile fasciculus stimulation occurring between the second and third trials. Timebase 5 ms/division, amplitude 0.5 μV/division
Some of the challenges of performing IONM in children include pre-existing conditions and the dynamic nature of nervous system development, which can lead to abnormal baseline neurophysiology. Cardiopulmonary co-morbidities in these patients can increase the risk of neuromonitoring changes intraoperatively, which may require more frequent or escalated interventions [49]. In those patients with pre-existing spinal cord pathology, IONM may be slightly less consistent—more so for MEPs than SSEPs—and need to be more frequently supplemented with other confirmatory testing such as a wake-up test [72].
Peripheral Nerve Monitoring
Monitoring for the peripheral nervous system is an important adjunct for many pediatric procedures, especially in the extremities. Tissue handling and dissection alone can directly or indirectly injure peripheral nerves, and the safety profile of pediatric operations can be enhanced by IONM. Modalities discussed earlier in this chapter such as SSEPs and tcMEPs can be of great value in monitoring the health of long tracts. These can reflect neurological injuries both in the surgical field, as well as distant changes in conduction. Children at high risk of neurological deterioration from hemodynamic changes and positioning, such as scoliosis patients and those with genetic or metabolic disorders should be considered for IONM during extremity surgery that may either be lengthy or require special positioning. Specifically, patients with Morquio syndrome highlight the importance of not only a multimodal IONM approach, but a multidisciplinary approach to their surgical care [73]. These patients are at high risk of cervical myelopathy secondary to atlantoaxial instability, which may be precipitated or aggravated during routine surgical care without significant precautions. Spinal cord injury has been documented following pediatric extremity orthopedic surgery, thought to be due to relative hypotension and positioning in the setting of severe kyphosis and scoliosis [74].
Along with long tract monitoring, local testing in the surgical field can be employed for monitoring peripheral nerve pathways. EMG and direct nerve conduction studies can aid the surgeon in identifying segments of injured nerve, and guide surgical decision-making in order to minimize the risk of any further injury [75].
Peripheral nerves can be distinguished from nearby lesions such as neurofibromas using direct stimulation. These can provide a better understanding of local nerve health than a purely visual inspection of a nerve, as immediate feedback of their function instead of waiting to examine the patient post-operatively. EMG may also be useful for non-extremity procedures, such as recurrent laryngeal nerve monitoring for pediatric anterior cervical surgery, or even cranial nerve monitoring during endonasal procedures [36, 76, 77]. More specifically, facial nerve IONM via EMG and nerve conduction studies, which is commonly performed in adult patients, is both safe and feasible in the pediatric population [78].
Conclusion
Neuromonitoring plays a major role in detecting and minimizing neurological injuries and thus maximizing safety during procedures that put nervous system structures at risk, and has become a significant presence in many pediatric operating rooms. It allows for real-time feedback of nervous system tissue health to the surgical and anesthesia teams in order to minimize the risk of neurological injury and guide further management. This immediate feedback reduces the surgeon’s dependence on the Stagnara wake up test, especially beneficial in the pediatric population where a wake up test may often be unfeasible. Further studies are needed to fully evaluate the risk reduction and cost effectiveness of IONM. Although limited by some anesthetic and age-related physiological considerations, a multimodal IONM approach provides detailed insight into conduction from the cerebrum out to dermatomes and myotomes. It is both safe and feasible to employ neurophysiologic monitoring for pediatric patients with appropriate adjustments in monitoring parameters to take into account the child’s age, health, and developmental status. Intraoperative neuromonitoring can be tailored to the individual child’s needs, and thus integrates well into the era of personalized medicine.
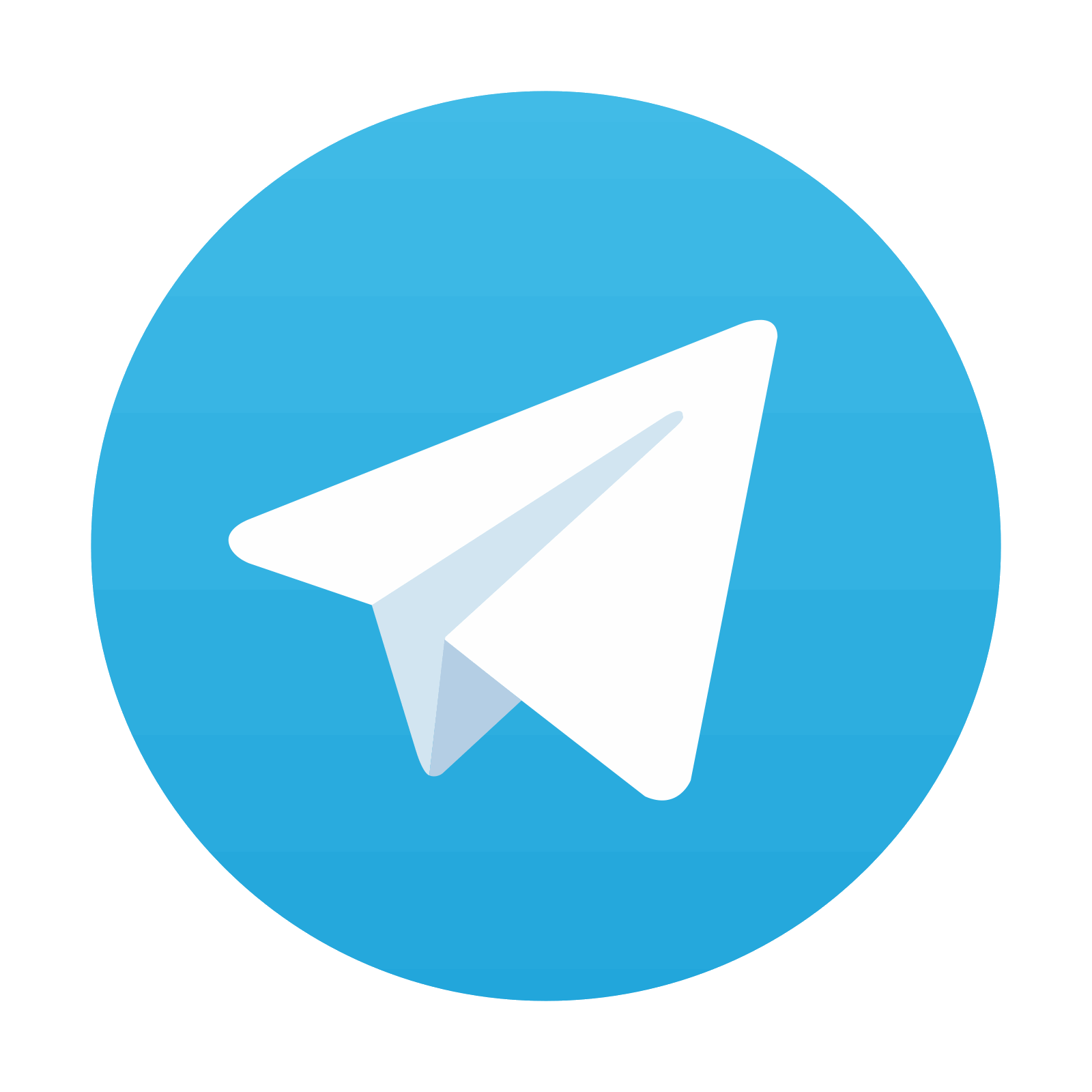
Stay updated, free articles. Join our Telegram channel
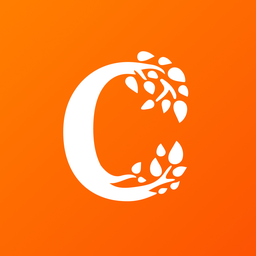
Full access? Get Clinical Tree
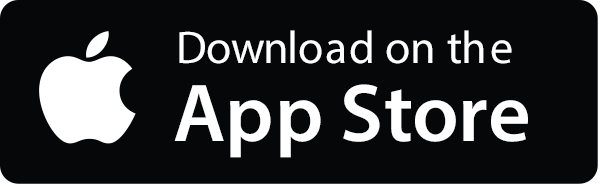
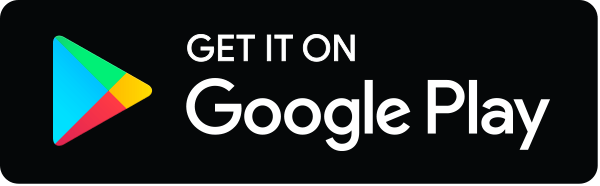