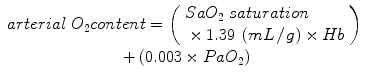
6.2 Oxygen Delivery Systems
The various methods to physically deliver oxygen depend on the patient’s respiratory status and oxygen demands. In the neonatal and pediatric populations, the options are patient specific as body habitus, size, and tolerance for various oxygen delivery devices vary significantly. The most important factor is the gas flow rate since this determines if the oxygen delivery system is sufficient to meet a patient’s inspiratory demand. If gas flow is inadequate, then in turn, the FiO2 is likely to be variable and insufficient as the patient entrains room air. We can better delineate this concept by dividing the available delivery systems into low-flow and high-flow devices.
Low–flow devices tend to provide a less consistent concentration of inspired oxygen and include traditional nasal cannulae, simple face masks, and tracheostomy collars.
High–flow devices provide a more reliable concentration of inspired oxygen to the patient regardless of respiratory effort. These devices include partial and non-rebreather face masks, nebulizers, high-flow nasal cannulae, oxygen hoods, noninvasive ventilation (NIV), and mechanical ventilation.
6.2.1 Low-Flow Devices
6.2.1.1 Nasal Cannulae
Various sizes of nasal prongs are available for the neonatal and pediatric populations. Oxygen can be supplied from a wall or tank source at a rate of 1–6 liters/minute (L/min) for the pediatric population. This provides an approximate FiO2 of 0.24–0.50. The actual FiO2 delivered to the patient depends on the patient effort and the potential for entrainment of room air. Humidification is important to minimize drying of nasal and upper airway secretions. In addition, a blender with ambient air can be used to decrease flows to as low as 0.025 L/min, which can be especially useful in the premature neonatal population.
6.2.1.2 Simple Face Mask
Such a device fits over the nose and mouth and provides a gas flow rate of 6–10 L/min and FiO2 of 0.35–0.60.
6.2.1.3 Venturi Face Mask or Tracheostomy Collar
This is a face mask with a controlled jet of high-flow oxygen which entrains a continuous flow of ambient air. Such a device allows a controlled provision of FiO2 at 0.24, 0.28, 0.31, 0.35, 0.40, or 0.50. This approach can be crucial for patients with chronic carbon dioxide retention. The manufacturer lists the predetermined flow rates required to provide the chosen FiO2. The FiO2 is dependable as long as the flow rate does not exceed the inspiratory demand of the patient.
6.2.2 High-Flow Devices
6.2.2.1 High-Flow Nasal Cannulae
In the neonatal intensive care unit (NICU), high-flow nasal cannula (HFNC) (>1 L/min for the neonatal population) has been introduced as an alternative to nasal continuous positive airway pressure (NCPAP) which has been the traditional standard of care for infants with respiratory distress syndrome (RDS) or apnea. Current HFNC devices deliver gases heated to near body temperature. These gases are saturated with water vapor and deliver typical gas flow of 1–5 L/min in the NICU population via a nasal cannula setup (Kubicka et al. 2008). This 1–5 L/min has a higher partial pressure than flow from a simple nasal cannula secondary to the degree of humidification. A theoretical concern with the use of HFNC has been the potential to produce an unknown and excessive pressure accumulation in the lungs. For a more in-depth review of this topic, please refer to Chap. 4 of this text.
6.2.2.2 Non-rebreather Face Masks
This delivery device combines a simple face mask with a reservoir bag and an inflow system for fresh gas, thus, allowing the system to theoretically deliver up to 100 % oxygen to the patient. This is made possible by two separate one-way valves. One valve is located between the mask and the reservoir, while the other is located at the exhalation port. This setup ensures fresh gas delivery with each inspiration while preventing the exhaled gas from being rebreathed. A safety backup in the design allows a port to open in case the flow of oxygen is disrupted, such that the patient can then entrain room air with inspiratory effort.
6.2.2.3 Partial Rebreather Face Masks
This mask is essentially the same setup as the non-rebreather mask except there is no one-way valve between the mask and the reservoir. Therefore, exhaled gas can mix with the oxygen in the reservoir bag, and thus, the delivered fraction of inspired oxygen is less than 1.0.
6.2.2.4 Oxyhood
This device allows for the delivery of high-flow oxygen to a contained space surrounding the patient’s head while allowing easy access for patient care. The FiO2 quickly re-equilibrates after any movement of the hood, which could allow for potential entrainment of room air. The oxygen level must be continuously monitored near the patient’s face as there is an increasing gradient in the FiO2 from the top of the hood to the bottom due to the slight increase in density of oxygen (1.42 kg/m3) as compared to room air (1.29 kg/m3).
6.2.2.5 Noninvasive Ventilation (NIV)
This mode of ventilation and oxygen delivery can be effective in decreasing atelectasis, improving alveolar ventilation, and decreasing respiratory muscle fatigue in specific groups of neonatal and pediatric patients (RDS, neuromuscular disease). Unfortunately, there are minimal pediatric studies in the acute care setting and even less so in direct comparison with intubation and mechanical ventilation. NIV does allow for close control of FiO2 from 0.21 to essentially 1.0. NIV is generally the preferred mode of support for patients with neuromuscular weakness and upper airway obstruction from poor pharyngeal tone and/or enlarged tonsils and adenoids. Although there are a variety of nasal and full-face masks for large pediatric patients and adults, the options for infants and small children in the USA are limited. For a more in-depth review of this topic, please refer to Chap. 4 of this text.
6.2.2.6 Oxygen Delivery via Invasive Mechanical Ventilation
Invasive mechanical ventilation allows for complete control of the gas mixture being administered to the patient via an endotracheal tube. A FiO2 of 1.0 can be delivered consistently regardless of patient effort, but this should be minimized to prevent oxygen toxicity to the lungs.
6.2.2.7 Hyperbaric Oxygen
Hyperbaric oxygen therapy is appropriate for those clinical conditions involving severely impaired oxygen delivery and altered hemoglobin-oxygen binding, such as carbon monoxide (CO) poisoning. Please see the corresponding section later in this chapter for further detail.
6.3 Physiologic Effects of Oxygen Breathing
6.3.1 Respiratory Effects
6.3.1.1 Breathing Control
Respiratory control is possible because of three key components: sensors, central control, and effectors (the muscles of respiration) (West 2000).
6.3.1.1.1 Sensors
Sensors include (1) central chemoreceptors, (2) peripheral chemoreceptors, and (3) pulmonary stretch receptors, each of which responds to changes in arterial oxygenation and inadequate ventilation.
1.
Central chemoreceptors exist in the milieu of the brain’s extracellular fluid and are located between the blood vessels and the cerebrospinal fluid (CSF). Carbon dioxide diffuses from the vasculature into the CSF, thus decreasing pH. This decrease in CSF pH stimulates the central chemoreceptors to increase ventilation.
2.
Peripheral chemoreceptors are located in the carotid bodies above and below the aortic arch. The carotid bodies are the most crucial element of the receptor system as they are responsible for an appropriate increase in ventilation in response to arterial hypoxemia. The response to changes in PCO2 is a less important role of the peripheral chemoreceptors.
3.
Pulmonary stretch receptors, a type of lung receptor, occur within airway smooth muscle. These receptors aid in decreasing respiratory frequency with lung distention. This is known as the Hering-Breuer reflex and is thought to be of greatest importance in newborn infants. Included within this group of receptors are the irritant receptors which lie between airway epithelial cells and are stimulated by noxious gases, such as cigarette smoke. This stimulus causes bronchoconstriction and hyperpnea. The juxta–capillary or J–receptors lie in the alveolar wall and are attributed to causing the rapid, shallow breathing exhibited in heart failure and interstitial lung disease.
6.3.1.1.2 Central Control
Central control of ventilation involves the brainstem as the primary mediator or triage center for the various types of input from the abovementioned sensors. Central control begins in the cerebral cortex, which supports voluntary (corticospinal) breathing, and incorporates input from the brainstem which is involved with automatic (reticulospinal) breathing. These signals are then conducted to the anterior horn cells of the spinal cord and on to the motor neurons that supply the muscles of respiration. The motor neurons (in the cervicothoracic portion of the spinal cord) propagate these signals to the peripheral nerves and finally across the neuromuscular junctions leading to initiation of the respiratory muscles. Alterations with any part of this system, from the brainstem to the musculature, can result in respiratory insufficiency or failure.
6.3.1.1.3 Effectors (Respiratory Muscles)
Effectors are the muscles involved in ventilation including the diaphragm. Excess effector activity does provide negative feedback to the brainstem, which efficiently allows control of ventilation and oxygenation such that the PaCO2 and PaO2 levels are usually maintained within the normal range. The lower motor neurons propagate the incoming signal to the peripheral (or efferent) nerves supplying the muscles of respiration. The efferent nerves extend to the diaphragm, intercostals muscles, and the accessory muscles of the neck. These nerves divide into branches that reach the specific muscle fibers and apply themselves to the muscle membrane at the motor endplates. At these neuromuscular junctions, the chemical transmitter acetylcholine is released. Acetylcholine is responsible for depolarizing the muscle membrane, which results in the release of intracellular calcium, thus, initiating the contraction of the muscle fiber (Polkey and Moxham 2001). The muscles of respiration are divided into three main groups: (1) inspiratory muscles, (2) expiratory muscles, and (3) accessory muscles of respiration.
1.
Inspiratory muscles: Inspiratory muscles provide outward forces and include our main inspiratory muscle, the diaphragm, which contributes almost three quarters of the inspiratory workload. Cervical nerves 3–5 supply the phrenic nerve, which controls the diaphragm. The external intercostal muscles provide additional inspiratory force, thus allowing elevation of the lower ribs and expansion of the rib cage during inspiration. They are innervated via the intercostal nerves, which derive from the thoracic spinal nerve roots.
2.
Expiratory muscles: This group includes the internal intercostals which aid in expiration by passive relaxation. In addition, the abdominal muscles (internal obliques, external obliques, and transverse abdominus) help to reduce the thoracic volume by contracting to displace the diaphragm into the thoracic cavity. An additional contributor to this group, the rectus abdominus, helps to raise the pleural pressure during exhalation thus aiding in airflow out of the thorax.
3.
Accessory muscles of respiration: This group includes the sternocleidomastoid, scalene, trapezii, latissimus dorsi, platysma, and pectoralis muscle groups. The accessory muscles contribute most prominently in situations associated with increased ventilatory demand, such as exercise, impending respiratory failure, and neuromuscular weakness. By contributing to rib cage expansion, these muscles support inspiration during active spontaneous breathing, though they may also add support during quiet breathing (Benditt 2006; Phillipson and Duffin 2005). The upper airways must maintain their patency throughout inspiration, and there is a corresponding increase in neural output to the pharyngeal wall muscles (genioglossus and arytenoid muscles) just prior to diaphragmatic contraction. Thus, the pharyngeal wall muscles are a crucial adjunct when the accessory muscles are in use.
6.3.1.1.4 Special Considerations for Infants
The infant’s compliant chest wall and more circular thorax make the above respiratory processes less efficient. With increased inspiratory effort and diaphragmatic contraction, the lower ribs descend rather than elevate secondary to the compliant nature of the chest wall. The result is subcostal retractions and deformation of the chest wall rather than full lung expansion. Compared to older children and adults, infants are more likely to exhibit muscle fatigue due to the increased workload on the diaphragm. In addition, the diaphragm of infants has fewer type I muscle fibers which are slow-twitch, high-oxidative fibers inherently more resistant to fatigue. As children mature, the rib cage and diaphragm achieve a less horizontal position, and the quantity of type I fibers increases. These changes lead to increased generation of maximal inspiratory pressures and, hence, a less compliant chest wall.
6.3.1.2 Pulmonary Vasodilatation
Pulmonary vascular resistance (PVR) is predominantly determined by the diameter, length, and number of pulmonary vessels. The ability to cause or allow pulmonary vasodilatation is dependent on the status and reactivity of the pulmonary vascular bed of each individual patient and may change within a given patient over time. The most important factor is the diameter of the pulmonary vessels, which is directly related to the vascular smooth muscle tone. In normal circumstances, minimal tone is present in the pulmonary circulation, and thus, pulmonary vascular resistance is low. Unlike the systemic vascular system, the pulmonary vasculature reacts to hypoxia with vasoconstriction. The exact mechanism is unknown but occurs when the alveolar PO2 falls below approximately 50–60 mmHg. It should be noted that alveolar hypoxia is a more important factor in influencing PVR than pulmonary arterial hypoxia. This pulmonary vasoconstriction is an adaptive attempt to prevent flow of blood to hypoxic regions of the lung, thus improving ventilation-perfusion matching. The pulmonary arterial pH also affects the pulmonary vasculature in a manner opposite to what occurs systemically with acidosis causing pulmonary vasoconstriction. The prevention and treatment of metabolic acidosis help to limit any existing pulmonary hypertension. In addition, elevated levels of carbon dioxide in the blood increase PVR independent of an acidotic or alkalotic metabolic milieu.
6.3.1.2.1 Specific Therapies to Decrease PVR
Vasodilators are used to decrease pulmonary artery pressure (PAP) and to halt or reverse the vascular changes related to an elevated PVR.
Oxygen: From the perspective of the pulmonary vasculature, the purpose of optimal oxygen delivery is to reverse hypoxic pulmonary vasoconstriction. With chronic hypoxemia, supplemental oxygen is given to maintain a goal arterial oxygen saturation level of ≥90 % (Rubin and Rich 1997).
Nitric oxide (NO): Inhaled nitric oxide (iNO) is a potent, selective pulmonary vasodilator that acts to dilate the pulmonary vascular smooth muscle (endothelium) via cyclic GMP (guanosine monophosphate). Inhaled NO has a very short half-life of approximately 4 s and is synthesized from L-arginine by NO synthase. Nitric oxide is avidly bound to hemoglobin, so the intended effect is local. Impaired endogenous production of NO is intrinsic to the pathogenesis of pulmonary arterial hypertension. Inhaled NO via a ventilator circuit, face mask, or nasal cannula has been used clinically since the 1990s in the treatment of pulmonary hypertension. It has also been used diagnostically in the cardiac catheterization lab to determine pulmonary vasoreactivity. Inhaled NO lowers pulmonary artery pressure (PAP) in various disease states, including idiopathic pulmonary hypertension, congenital heart disease (CHD), and postoperative pulmonary hypertension (Atz et al. 2002) (Table 6.1). Rare side effects include systemic hypotension, methemoglobinemia, and excess generation of nitrogen dioxide (a highly reactive oxidant). The most significant risk of iNO is rebound pulmonary hypertension, which can occur when weaning a patient from iNO. There are data advocating a slow wean of iNO at doses less than 5 ppm. The addition of a single dose of sildenafil 1 h prior to discontinuing iNO therapy has been demonstrated to blunt the rebound effect (Namachivayam et al. 2006).
Table 6.1
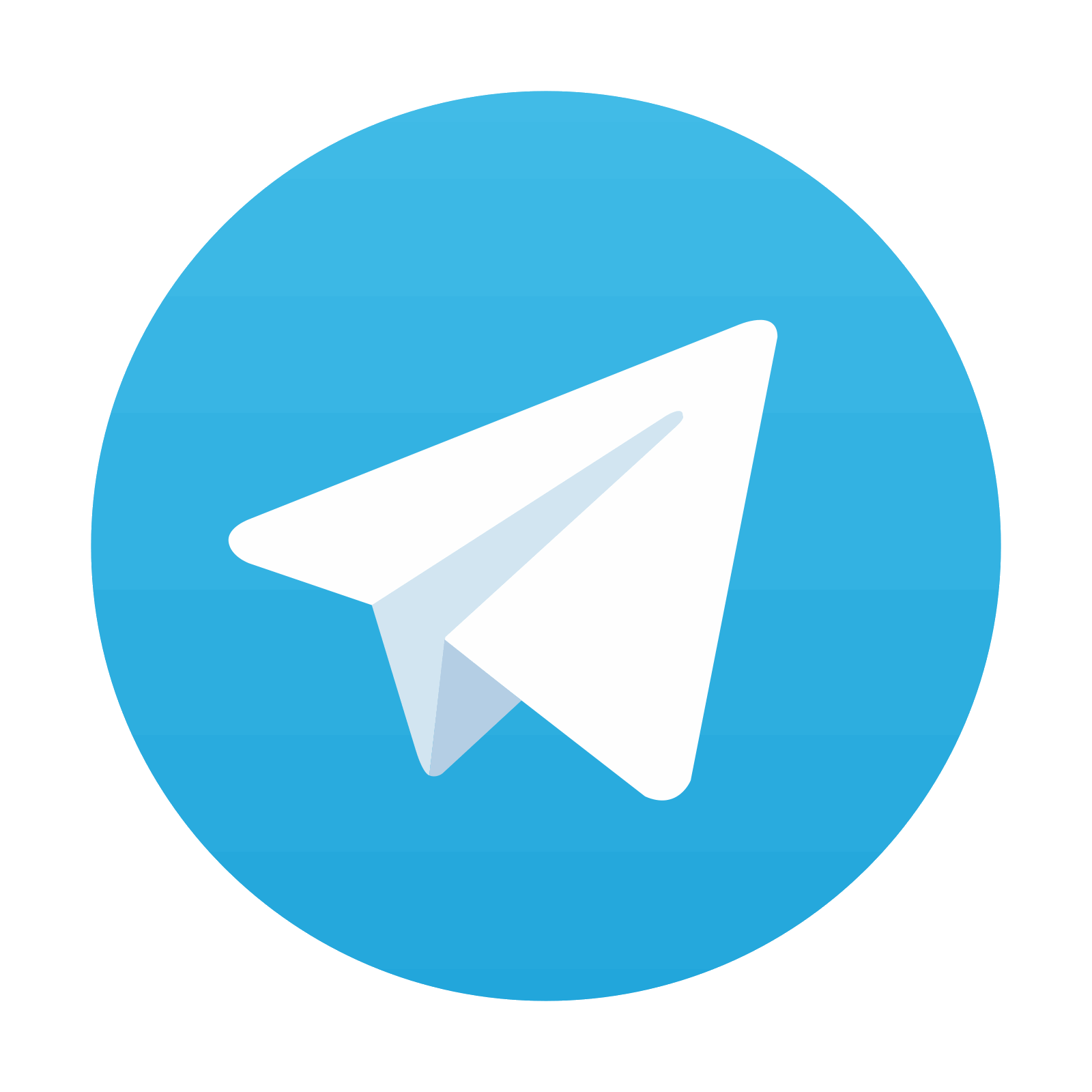
Fraction of inspired oxygen by various delivery systems
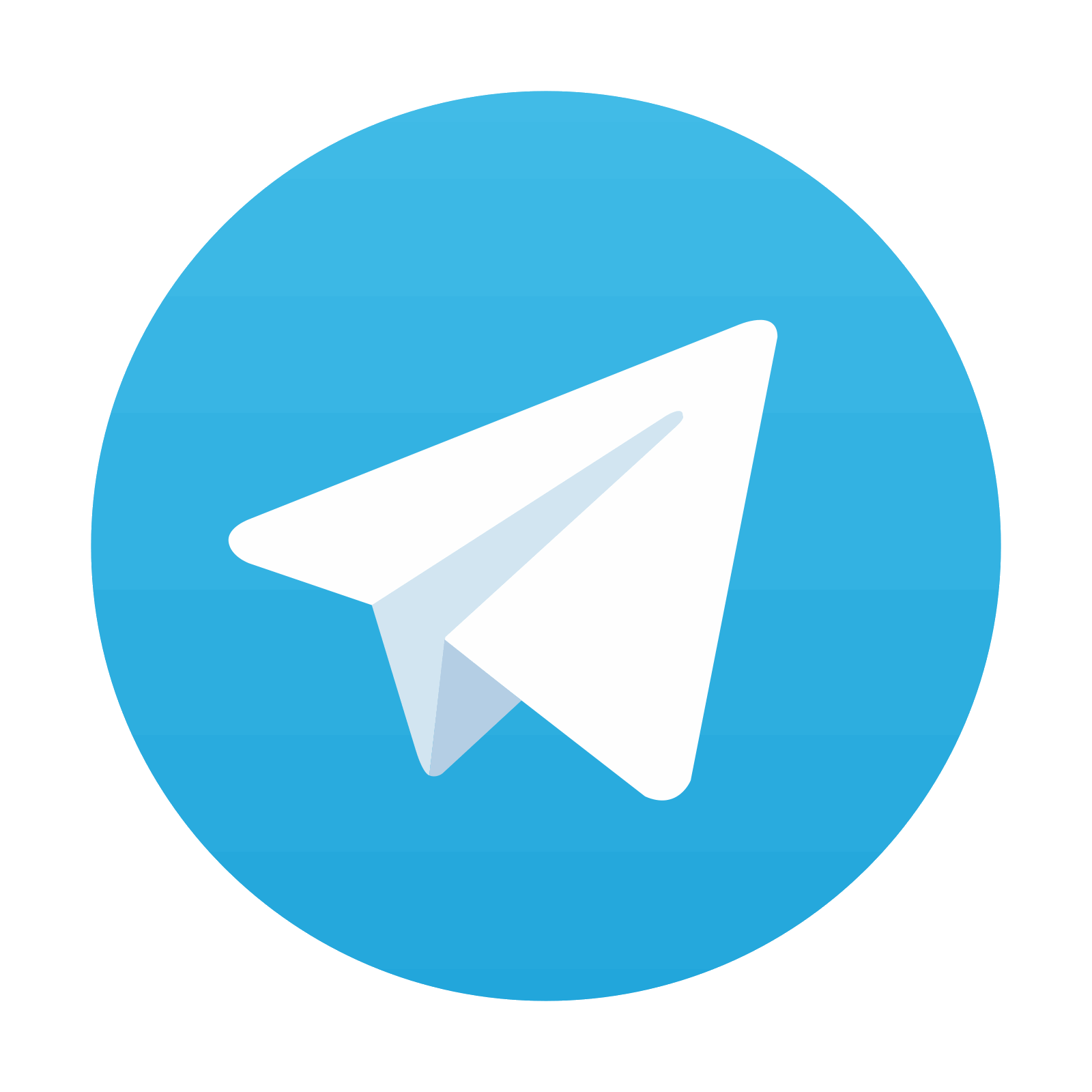
Stay updated, free articles. Join our Telegram channel
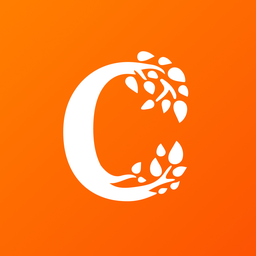
Full access? Get Clinical Tree
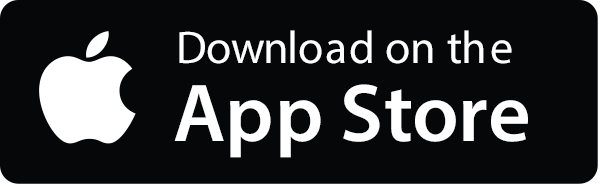
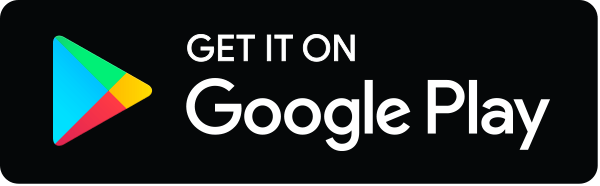
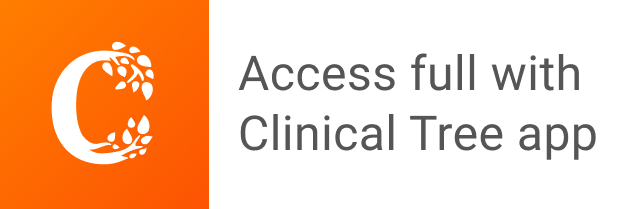