Epilepsy is suspected when there is repetition of seizures or an unprovoked seizure is accompanied by a probability of further seizures similar to the general recurrence risk after two unprovoked seizures (1). Children with epilepsy present multiple challenges to the clinician. The cause and clinical spectrum of epilepsy are extremely wide-ranging in children (2). Moreover, there are age-specific changes in the semiology/types of seizures that might be the result of the differences in the connectivity and functionality of different brain regions. Epilepsy is more than a repetition of seizure. There is an increased risk of psychiatric and cognitive comorbidities that impact the quality of life of children even more than the seizures themselves, and have a significant impact on the life of the family (3).
Epidemiologic studies show that seizures and epilepsy affect infants and children more than any other age group (4). Febrile seizures are also very frequent, occurring in about 2% to 5% of children worldwide (5). Febrile seizure usually occurs between 3 months and 5 years, with a peak incidence at 18 months (6,7). New-onset seizure occurs more frequently during the first few years of life (8). Finally, the incidence of status epilepticus (SE) in developed countries is higher in children than in adults (9–11). These clinical data suggest that the developing brain is more prone to exhibit seizure than an adult brain. This has been shown in experimental models with immature animals (12). The propensity of the immature brain to generate seizures as shown in experimental models derives from multiple factors. Some cellular and molecular developmental characteristics contribute to the seizure susceptibility of the immature brain (Table 1.1). In addition, there are multiple underlying etiologies of epilepsy in children and adolescents contributing to the emergence of seizures. However, our understanding of the reasons why specific syndromes appear with precise age-relatedness is still very limited.
SEIZURE SUSCEPTIBILITY OF THE IMMATURE BRAIN
In contrast to seizures in infants and children, most neonatal seizures are acute and symptomatic. Very few seizures are part of a defined epilepsy syndrome. Neonatal seizures might result from various etiologies. During the birthing process, the newborn is at risk for insults that can result in seizures. These insults include birth trauma, hypoxic-ischemic insults, perinatal acquired infections, intracranial hemorrhage in preterm babies, and metabolic disturbances.
The rates of incidence of unprovoked seizures in children range from 63 to 134 per 100,000 children per year (13–16). Even if the methods of these studies are really heterogeneous, the incidence is the highest in the first year of life (4,17). The incidence of the first unprovoked seizure or newly diagnosed epilepsy was 134.4/100,000 (51.1–217.7) in children under 1 year old, decreasing to 50.4/100,000 (CI 95%: 24.9–75.9) in children aged 1 to 4 years old, and continuing to decrease across age categories 5 to 9 (41.5, CI 95%: 21.8–61.3) and 10 to 14 years old (30.7, CI 95%: 13.3–48.1) (4). The observational adult and pediatric prospective studies, taken as a whole, provide an estimate of a 2-year recurrence risk of 40% (18–20).
The immature brain is also vulnerable to epileptic encephalopathies with age-specific features.
Data of Seizures During Development From Animal Models
Rats are commonly used during development in epilepsy research. There are differences in the rate of maturation of different processes in the nervous system (such as synaptogenesis, myelination, etc.) between human and animal brains, making estimation of equivalences in developmental milestones between species a multidimensional and inexact task (21,22). Using various parameters, we consider the correspondence in maturation as follows: P7: the postnatal day; P10: term human newborn; P14 to P21: toddlers to young children; P28: older children prior to puberty; P35: adolescent (21,22).
TABLE 1.1
FACTORS THAT POSSIBLY CONTRIBUTE TO SEIZURE SUSCEPTIBILITY OF THE DEVELOPING BRAIN COMPARED TO AN ADULT BRAIN* |
Normal brain development |
• Paradoxical depolarizing action of GABA • Maturation of GABAergic synapse and subunit composition of GABA receptors • Developmental subunit composition of AMPA and NMDA receptors • Incomplete glial clearance of glutamate and potassium • Greater probability of nonsynaptic (ephaptic) spread of excitation • More sensitive to inflammation and hyperthermia |
Abnormalities during brain development |
• Gene mutation • Abnormal brain or cortical development • Brain injury around birthing process: hypoxia, infection, hemorrhage, stroke • Early life seizure (seizures beget seizures) • Epileptogenesis (following a double hit injury, following status epilepticus) |
*Some factors have been established only in animal studies.
Various seizure precipitants such as chemoconvulsants (kainic acid, pentylenetetrazol) (23), pilocarpine or lithium-pilocarpine (24), electrical stimulation (25), hypoxia (26), or hyperthermia have shown that the rodent brain in the P7 to P21 rats is highly susceptible to seizure. The amount of the chemoconvulsant required to precipitate seizure in the young animal is lower than in the adult (12).
Several factors contribute to the higher susceptibility to seizures in the developing brain. These include external factors such as hypoxia, hypoxic-ischemic insults, and CNS infection. These factors are particularly frequent in the newborn. Structural brain abnormalities and/or genetic factors are also frequent causes of the seizure susceptibility. In addition to these factors, some developmental characteristics of the immature brain predispose the immature brain to seizure.
Developmental Factors Predisposing the Immature Brain to Seizures (Table 1.1)
Regardless of the underlying factors, such as gene mutation, structural brain abnormalities, or any type of brain injury, several intrinsic factors contribute to a higher susceptibility in the immature brain to generate seizures compared to the mature brain (27). The developmental changes of the neurotransmitter system in the brain seem to be key factors of the seizure susceptibility. Neurotransmission in early life is characterized by an enhancement of the excitatory system, while the inhibitory system is less efficient or exhibits a paradoxical excitatory effect (28).
A change of the composition of the glutamate receptors during development results in changes in the brain’s excitability. The subunit composition of the NMDA receptors in early life promotes extrasynaptic localization, slow kinetics (NR2B), decreased calcium influx (NR3), and decreased sensitivity to magnesium blockade (NR2D, NR3) (29–31). Across development, the increase in the NR2A subunit promotes the postsynaptic localization of the NMDA receptors (32,33). NMDA receptors binding studies have also demonstrated age- and region-specific patterns (34). The subunit composition of α-amino-3-hydroxy-5-methyl-4-isoxazolepropionic acid (AMPA) receptors also changes during brain development. AMPA receptors of the immature brain tend to be relatively deficient in glutamate receptor 2 subunit (GluR2) as compared to the more mature brain. This subunit regulates the permeability to calcium though the AMPA receptors (30), such that the immature brain is more permeable to calcium. Immature brains also exhibit higher expression of the flip GluR1 isoform that reduces AMPA receptor desensitization (31). These changes in both receptor types result in increased excitatory properties of the developing brain that will gradually decrease until late childhood.
There are several developmental facts (presynaptic and postsynaptic) that lead to a decrease in the efficiency of the GABAergic pathways. The most predominant is probably that GABA exerts a paradoxical depolarizing action during the early postnatal period. However, presynaptic mechanisms, such as incomplete migration of GABAergic interneurons, are not fully developed. GABAergic synapses (35) are also probably involved in the developmental changes of the GABAergic pathways. Postsynaptic changes include a modification of the subunit composition of GABAA receptors with a preferential expression of subunits conferring slow kinetics during early development (36,37). The subunit composition of GABAA receptors makes them also less sensitive to benzodiazepine augmentation (38–40).
The paradoxical depolarizing action of GABA has been shown in all animal species and brain structures studied to date. In rats, GABA produces depolarization of neurons up to P14 (41,42) and until the third trimester in utero in primates (43). The lack of efficient GABAergic inhibition increases excitability and can facilitate synchronicity (44). Indeed, a correlation has been observed between the incidence of seizures and the excitatory to inhibitory shift of GABA’s effect (Figure 1.1). The paradoxical excitatory action of GABA is the result of a change across the development of chloride transporters. During early development, there is a preponderance of chloride importers in neurons, such as the sodium-potassium-chloride cotransporter 1, NKCC1, over chloride exporters, like the potassium-chloride cotransporter 2, KCC2. This results in a relatively elevated intracellular chloride level (45,46). When GABAA receptors are activated, chloride flow is outwards, causing neuronal depolarization (45–50). During development, there is a gradual increase in the KCC2 cotransporter, which lowers the intracellular chloride level, resulting in a facilitation of hyperpolarizing GABAeffect in more mature neurons (46) (Figure 1.1).
When GABA interact with GABAAR, the opening of the GABAAR results in a chloride flux driven by the intracellular and the extracellular concentration of chloride. In the case of an immature neuron, NKCC1, which is responsible for the import of chloride into the cell, is more important than KCC2, which is responsible for the export of chloride out of the cell. This results in a relatively higher intracellular chloride concentration compared to the extracellular compartment, leading to an outward current inducing a depolarization of the cells. In the case of a mature neuron, KCC2 is more important than NKCC1, resulting in a chloride flow into the cell. A hyperpolarization is observed.
Understanding of this phenomenon leads to the use of the loop diuretic, bumetanide, inhibiting NKCC1 (51). The preclinical studies were promising with an enhancement of the efficiency of the barbiturates to suppress neonatal seizures (52) and an inhibition of rapid kindling in rat pups (53). However, the clinical trial brought to light some safety issues without demonstrating a clear benefit to human newborns (54).
The depolarizing effect of GABA should not be seen only as a vulnerability of the immature brain that increases the risk of seizure. This phenomenon seems necessary for normal brain development, including neuronal differentiation and neuronal migration (47,48,55). During early brain development, depolarizing GABA receptors mediate fast excitation, a process that is eventually replaced by glutamatergic action on non-NMDA receptors sensitive to AMPA. It has been shown that the shift to hyperpolarization of the GABAergic may disrupt the maturation of dendritic processes and excitatory synapses with a change in the properties of the neurons to process and exchange information (47,48,55–58).
FIGURE 1.1 Neuronal chloride concentration according to the development.
Other factors that contribute to the abnormal excitability of the immature brain include the presence of gap-junctions, smaller extracellular spaces (partly due to paucity of glial proliferation), all contributing to nonsynaptic modes of synchronization and spread of excitation (59). Further, lack of glial maturation is also associated with a limited capacity for removal of glutamate (60) and potassium (61) from the immediate synaptic milieu.
Febrile Seizures
FS account for the majority of seizures seen in children younger than 5 years old (62). A FS is a seizure accompanied by fever, without any infection of the central nervous system, occurring in children between 6 months and 5 years of age. The International League Against Epilepsy defined FS in 1993 as “an epileptic seizure occurring in childhood after the age of 1 month, associated with a febrile illness not caused by an infection of the central nervous system, without previous neonatal seizures or a previous unprovoked seizure, and not meeting criteria for other acute symptomatic seizures” (63). The age-specific occurrence of FS also indicates that, even after the neonatal period, the developing brain continues to be predisposed to seizures.
The underlying mechanisms responsible for the occurrence of FS remain incompletely understood (64). Figure 1.2 represents the current understanding of the various factors contributing to the occurrence of febrile seizure. The association of several factors are involved in each patient, among which one can find an immature brain, increase in body temperature, inflammatory response, genetics, and viral infection (65).
FS are genetically complex disorders believed to be influenced by variations in several susceptible genes. Six susceptible FS loci have been identified: FEB1 (8q13–q21), FEB2 (19p13.3), FEB4 (5q14–q15), FEB5 (6q22–q24), FEB6 (18q11.2), and FEB7 (21q22). Recently, several association studies on FS have been reported, but the results vary among the different groups and no consistent or convincing FS susceptibility gene has emerged. IL-1β increases NMDA receptor function through activation of tyrosine kinases and subsequent NR2A/B subunit phosphorylation, resulting in an increase of NMDA-induced calcium influx. TNF-α causes an increase in surface expression of neuronal AMPA receptors responsible for an increase of synaptic efficacy. Viral infections are the most common causes of fever in FS. Human herpesvirus-6 and influenza are frequently discussed as a possible mechanism of FS because of their neurotropic properties, but the role of the virus and the related inflammation remain to be understood.
There is no evidence to support the common belief that the rise in temperature per se is more important for the development of FS than the actual temperature achieved (66,67).
Numerous studies have pointed out the genetic influences on FS. A positive family history of FS is a definite risk factor for both a first FS (68,69) and recurrent FS (68,70–73). The concordance rate for febrile seizure is 56% in monozygotic twins and 14% in dizygotic twins (74). Currently, it seems that FS have a multifactorial mode of inheritance, but there may be a subset of children with an autosomal dominant mode of inheritance (75).
FIGURE 1.2 Suspected mechanisms of febrile seizures.
There are also cumulative data suggesting that inflammation has an important role in both the development and long-term consequences of FS. IL-1β seems to play a key role in the factors acting to precipitate FS. Using hyperthermia-induced seizures in mice, Dubé et al showed that an intracerebroventricular (i.c.v.) injection of IL-1β lowered the threshold for hyperthermic seizures in mouse pups, and was even capable of producing seizures without a thermal provocation. This effect is related to the interaction of IL-1β with its receptor (76). In humans, an association between polymorphisms involving the interleukin-1β-511T allele prolonged febrile convulsions, and the emergence of temporal lobe epilepsy has been reported in the Japanese population (77). These data have been challenged by other studies. However, a meta-analysis suggests that a link between IL-1β gene polymorphism and TLE probably exists (78).
In order to induce fever in animal models, injections of lipopolysaccharide (LPS) have also been used. In adult rats, the injection of LPS results in a biphasic increase in body temperature driven by an inflammatory response. The use of LPS at room temperature in rat pups does not result in such an increase in body temperature. However, an increase in body temperature is observed when the room temperature is about 30°C, corresponding to thermoneutrality in rat pups (79).
When LPS is injected i.p. 2 hours before a subconvulsant dose of kainate (1.75 mg/kg), P14 rat pups experience an increase in body temperature and seizures. They exhibit face scratching, lying on one side, and wet-dog shakes (79). At the onset of seizures, IL-1β is significantly increased in the hippocampus but not in the hypothalamus or cortex when compared to rats that do not undergo seizures, despite the fact that they are also treated with LPS and kainate. When IL-1β is given i.c.v., the number of animals experiencing a seizure after a subconvulsant dose of kainate is significantly increased and the time to seizure onset is reduced, while the opposite occurs after i.c.v. injection of an antagonist of the interleukin-1β receptor (IL-1RA). Thus, IL-1β clearly contributes to seizure occurrence in P14 rats in this model (80,81).
In contrast, LPS at low doses, which does not increase core temperature, does not change acute susceptibility to short hyperthermic seizures in either P11 or P16 rats (82). However, these animals develop a lower threshold for pentylenetetrazol-induced seizures when they are adults. A similar nonfebrile dose of LPS does not exacerbate lithium-pilocarpine-induced status epilepticus at P7 or P14, although it does induce a delay in the onset time of status epilepticus; however, these rats display an increase in seizure-induced hippocampal damage (83). It appears from these findings that relatively low doses of LPS in immature rats, which do not increase core temperature, do not alter acute susceptibility to seizures although they increase seizure-induced cell loss (described previously) and long-term predisposition to seizures, described later in this article. The effects of LPS in immature and adult animals are clearly different, since nonfebrile doses also exacerbate seizures in adults (84,85).
The mechanisms underlying these effects, and the role played by fever and brain inflammation in determining the threshold to acute seizures, remain to be elucidated. However, these studies provide evidence that inflammation, especially IL-1β, contributes to the occurrence of seizures in the developing brain.
Effects of Seizure on the Developing Brain: Do Seizures Beget Seizures?
The idea that seizures beget seizures was first suggested by William Gowers (86). Clinical data are conflicting about this because the etiology or natural history of different pediatric seizure syndromes varies, making it difficult to confirm this concept readily in animal models that bear direct relevance to the clinical circumstances involving children. Self-limited epilepsy syndrome, such as rolandic epilepsy, remit spontaneously (87). Other epilepsy syndromes have a progressive worsening of the seizure frequency and seizure severity. However, the natural history of each syndrome is related to the underlying mechanisms, even if an interindividual variability also induces some variability in the electroclinical phenotype (88).
The natural history and the consequences of prolonged FS in children has been addressed by the FEBSTAT study (89). This multicenter prospective study included children from 1 month to 5 years who experienced a FS lasting 30 minutes or more. MRI studies performed within 72 hours of the episode of febrile SE of 191 children were compared to 96 controls (historical cohort of children with simple FS). T2 hyperintensity in the hippocampus was more frequently observed in children with febrile SE suggestive of an acute hippocampal injury (90). This acute injury seems to be followed frequently by hippocampal sclerosis (91). It is currently unknown if these patients carry a higher risk to develop temporal lobe epilepsy.
Kindling models of epilepsy suggest that seizures beget seizures. In the kindling model, the repetition of a subconvulsive stimulation, usually focal electrical stimulation, to limbic structures results in the progressive emergence of focal and then generalized seizures. When the model is established, the increased susceptibility to exhibit a seizure with a subconvulsive stimulation persists over time (92,93). Moshé (25) and Moshé and Albala (94) were the first to show that immature rats can be kindled and that the effects of kindling are life-long. In 1985, Lothman introduced a model of epileptogenesis that was based on the rapid kindling phenomenon (95). Under conditions of conventional kindling, the epileptic state is generally achieved within 1 to 2 weeks. However, by manipulating the parameters of the electrical stimuli applied to the ventral hippocampus of adult rats, Lothman et al were able to compress the progression to the kindled state to several hours (95). The feasibility of the model in the immature rat was confirmed by Michelson and Lothman (96), who provided a detailed description of both behavioral and electrographic hallmarks of rapid kindling in immature rats at several ages, including postnatal days 7 (neonatal), 14 (postneonatal), 21 (preadolescent), and 28 (adolescent). These data show that at different stages of development it is possible to induce kindling. This finding also created a new opportunity for pediatric epilepsy research; indeed, using the rapid kindling model, epileptogenesis could now be reproduced within a very narrow and specific ontogenic window of interest, thus creating a system usable both for basic research and preclinical development of antiepileptic drugs tailored to specific ages (97).
Recurrent seizures induced in developing rodents using flurothyl or pentylenetetrazol also result in a decrease of seizure threshold in adulthood (98–100). Hypoxia-induced seizures, in a neonatal rat model, are also responsible for an increase in seizure susceptibility. Despite the usefulness of the in vivo studies in providing some evidence that seizures beget seizures, limited data are available on the mechanisms leading to this long-term change in brain excitability. Using transgenic knock-in mice with a deficit in GluR1 S831 and S845 phosphorylation, it has been shown that GluR1 S831 and GluR1 S845 phosphorylation form a critical link in epileptogenesis. This has been shown with pentylenetetrazol-induced seizures in P7 mice and P9 hypoxic seizure in mice. The GluR1 S831 and GluR1 S845 phosphorylation has been also found in human hippocampal samples from neonatal seizure autopsy cases (101).
Effects of Seizure on the Developing Brain: Risk of Cognitive Impairment
Epilepsy syndromes are more than a “simple” recurrence of seizure. Children and adolescents with epilepsy have a higher risk of exhibiting cognitive and psychiatric comorbidities even in syndromes that were considered benign in the recent past.
Children with epilepsy more frequently have cognitive impairment than the general population. The intelligence quotient (IQ) scores are more frequently lower than controls. Specific learning difficulties without any decrease of the IQ can also be observed (102–104). This results in a higher risk for academic underachievement, and they are more likely to be placed in special education classes in school (102,105). Even children with a normal IQ and well-controlled seizures are at high risk for learning problems. In most children with epilepsy, the patients maintain stable IQ scores. There is evidence that some children with epilepsy display compromised mental development (8). In some epilepsy syndromes such as epileptic encephalopathy, a cognitive decline or a regression of development can be observed.
There are multiple factors contributing to cognitive impairment in children with epilepsy: age of onset, seizure type, seizure frequency, the antiepileptic drugs, and the etiology (103). There exists substantial evidence that repetitive seizures contribute to cognitive impairment. The study of mechanisms responsible for the cognitive deficit related to repetitive seizures is an area of active research.
Animal models have been helpful to evaluate the consequences of seizures on the developing brain. Since SE might have consequences different from those of repetitive seizures (including the presence of cell injury) on the developing brain, we focus here on short recurrent seizures. Experimental models of repetitive seizures seem more relevant to pediatric epilepsy. A section on status epilepticus is also included in this chapter (see later text).
Numerous studies demonstrate that recurrent seizures during brain development result in long-term changes (106). Various models of seizures with low mortality rate, such as pentylenetetrazol (107), flurothyl inhalation (108) and hyperthermic seizures, have been used (109,110). Rats that have experienced recurrent seizures during the first 2 weeks of life exhibit cognitive impairment when they are adults (106).
After recurrent seizures in early life, an impairment of visual–spatial memory has been usually shown using the Morris water maze test. When they are adults, the rats require longer times to find the hidden platform than control animals (106). This cognitive impairment is not associated with any discernible cell loss (99,111,112). The rats that have been exposed to recurrent seizures have aberrant sprouting of mossy fibers (axons of dentate granule cells) in the CA3 and supragranular regions of the hippocampus, reduced neurogenesis, morphologic changes in dendritic spines, and changes in glutamatergic and GABAergic receptor distribution (98,99,113–116). It is known that cognitive impairment can also occur after seizures in early life without mossy fiber sprouting (109). Whether the histologic or molecular changes observed are compensatory or maladaptive has been a matter of considerable debate and research (117–119).
Cognitive Impairment in Epileptic Encephalopathies
Epileptic encephalopathy (EE) as a concept suggests that not only the seizures but also the epileptiform abnormalities themselves can have an impact on cognition and behavior, above and beyond what might be expected from the underlying pathology alone (eg, cortical malformation), and that these impairments can worsen over time (2). The understanding of the mechanisms of EE remains limited, and may not be the same in different genetic etiologies of EE or may find expression leveraging final common pathways, regardless of the etiology. There are probably multiple mechanisms resulting in cognitive regression or cognitive involvement in EE. Here, we propose to present the current understanding of the cognitive deficit in two contrasting cases in terms of the occurrence of seizures: (1) Dravet syndrome with medically refractory seizures including bouts of SE and a cognitive impairment that seems more related to the gene mutation responsible for the emergence of the electroclinical phenotype; and (2) EE with continuous spike-and-wave during sleep (EE-CSWS) that is characterized by few seizures but discernible regression of cognition.
Dravet Syndrome
Dravet syndrome (DS) is a refractory epilepsy syndrome characterized by early-onset, febrile or afebrile, generalized or unilateral, clonic or tonic–clonic seizures. Usually the first seizures are long-lasting episodes of SE occurring during the first year of life in an otherwise normal infant. Later, myoclonus, atypical absence, and partial seizures are observed. Mutations in the voltage-gated sodium channel gene SCN1A are the main genetic cause of DS (60%–80% of the patients) (120). Developmental delay is constant, resulting from stagnation rather than cognitive regression. This usually begins to be evident after 2 years of age. Behavioral disturbances are also very common and seem to correlate with the degree of cognitive impairment (121,122). Until recently, DS has been considered as an EE and it has been suggested that the epileptic characteristics (age at onset, type, and duration of seizure) play an important role in determining the heterogeneity of outcomes in these patients.
At adulthood, all patients showed intellectual disability; but even though severe intellectual disability was prevalent, heterogeneous outcomes were also described. The electroclinical phenotype seemed to be predictive of the level of cognitive impairment, with milder intellectual disability observed in patients with atypical DS (123). Two other studies analyzed in greater detail the developmental profile across childhood. The first study showed that cognitive impairment occurred early, and no patient with DS had a normal IQ after the age of 6 years. They exhibited a profile of poor communication and poor capacity for autonomy, whereas their socialization skills seemed better preserved. Interestingly, there was no correlation between IQ (between 6 and 10 years of age) and epileptic characteristics (age at seizure onset, number of prolonged seizures) during early development, that is, the first 2 years of life (124). These data are consistent with a second report of 81 neuropsychologic evaluations performed in 67 patients with DS (125). In this study, cognitive involvement is also an early process, with an IQ above 70 observed until 3 years of age followed by a marked decrease (mean IQ: 48) after this age. Notably, none of the patients experienced psychomotor or cognitive regression. Once again, the cognitive impairment of patients with DS appeared unrelated to major epileptic factors (age, type or duration of first seizure, number of fever-related episodes of SE, photosensitivity at EEG recording, or treatment parameters). Both studies underline that cognitive impairment in DS is likely not a direct consequence of epilepsy, and that the presence of a mutation in SCN1A was actually a more reliable predictor of cognitive outcome (125).
The use of a “Dravet” mouse model generated by heterozygous knockout (KO) of the Scn1a gene (Scn1a+/−) has contributed to a better understanding of the neurobiology of this syndrome. The genetic modification is responsible for an altered function of Nav1.1 sodium channels in neurons clustered throughout the brain (126). An impairment of the γ-aminobutyric acid GABAergic firing has been observed in the hippocampal interneurons, resulting in the seizure susceptibility and in the cerebellar Purkinje cells, explaining the motor symptoms such as the ataxia (126,127). In the mouse model, the consequences of SCN1A abnormality differ according to the genetic background. In a heterozygous Scn1a knockout (Scn1a+/−) mouse model of DS, the animals exhibited strain-dependent seizure severity and survival: Scn1a+/− in the mouse strain 129S6/SvEvTac (129.Scn1a+/−) had no overt phenotype and showed normal survival compared to Scn1a+/− mice bred from a C57BL/6J background (F1.Scn1a+/−), which displayed severe epilepsy and premature mortality. These findings also correlated with cellular recordings (sodium current density in pyramidal neurons and GABAergic interneurons and spontaneous action potential firing in pyramidal neurons) (128). Differences in the extent of preservation of sodium current density in GABAergic interneurons between the two different genetic backgrounds probably explains the different phenotypes (128).
More recently, behavioral problems have also been reported in the Dravet mouse models, including learning impairment, hyperactivity, stereotyped behaviors, and deficits in social interactions (129,130). These behavior disorders seem to be related to an impaired GABAergic neurotransmission in the prefrontal cortex, which has a particular low expression of Nav1.1 channel in the GABAergic interneurons. Interestingly, low-dose clonazepam, a positive allosteric modulator of GABA-A receptors, completely rescued the abnormal social behaviors and deficits in fear memory (130), suggesting that behavior/cognition is due to the impairment of the GABAergic neurotransmission. Other experimental studies have demonstrated that genetic modifications of SCN1A can result in cognitive impairment without seizure. Using an siRNA approach to selectively knock down Nav1.1 in mice, the effective reduction in Nav1.1 expression in the medial septum and diagonal band of Broca led to a dysregulation of hippocampal theta oscillations in association with a spatial memory deficit, although the mice did not exhibit spontaneous seizures (131). This study also suggests that the SCN1A mutation directly contributes to cognitive impairment independent of seizure induction. Both the neuropsychologic studies and these experimental studies suggest that the genetic factors play an important role in the cognitive outcome of DS, and that this syndrome should be regarded as a genetic encephalopathy with epilepsy rather than an EE (132).
Epileptic Encephalopathy With Continuous Spike-and-Wave During Sleep/Landau–Kleffner Syndrome
EE-CSWS and Landau–Kleffner syndrome (LKS) are two distinctive EEs with different clinical phenotypes. In the EE-CSWS, there are typically few seizures between 2 to 4 years of age. Around 5 to 6 years of age, the seizures become more frequent, including head drops in some cases and a marked cognitive and behavior regression is observed. Landau-Kleffner syndrome is mainly characterized by a gradual inability to understand and use spoken language due to verbal agnosia while the seizures usually respond well to treatment.
Both syndromes are characterized by a regression of all or a part of the cognitive abilities (verbal agnosia for LKS) in a child with spike–wave discharges during sleep. These syndromes should be considered as a prototype for the EEs. These rare epilepsy syndromes typically start with seizures around 2 to 4 years of age in a child with normal or moderately abnormal baseline development. Seizures are not frequent. Around age 5 to 6 years, severe and global neuropsychologic regression occurs and seizures usually become more frequent. The sleep EEG recording shows continuous spike and wave. At 2 to 4 years after the occurrence of the regression, seizures tend to remit and the neuropsychologic deficits tend to stabilize or even improve, although severe residual impairments remain, particularly when the treatments were not able to modify the CSWS EEG pattern (133).
The cognitive regression has a wide range of severity, resulting in a majority of patients with an evident decrease of the intelligence quotient. In the particular case of LKS, the regression consists of verbal agnosia that later results in global language deterioration (133).
The sleep EEG pattern was first described in 1971 (134). The EEG pattern is sometimes called electrical status epilepticus in sleep (ESES) in the literature, as opposed to CSWS, the latter term preferentially used to refer to the epilepsy syndrome. ESES pattern consists of generalized bilateral and symmetric 1.5 to 3 Hz spike-waves. It was initially believed that 85% of the non-REM sleep is occupied by the SW discharges (135,136). It is currently unclear if the percentage of SW discharges occupying the non-REM sleep represents a threshold quantity to observe/initiate the behavior–cognitive involvement in these syndromes.
The literature does not use a common terminology to distinguish an isolated EEG pattern (spike-wave discharge during sleep) from EE-CSWS (137). There are many names for these epilepsy syndromes. Most often, the authors use “ESES” when referring to the EEG pattern, “CSWS” when referring to EE with global regression, and “Landau–Kleffner syndrome” when discussing EE with predominant language regression. In EE-CSWS, the main feature is the cognitive regression that is associated with continuous spike-and-waves during sleep. The recording of a similar pattern in a patient without any cognitive regression should not lead to the diagnosis of EE-CSWS. The interchangeable use of “ESES,” “CSWS,” and “Landau–Kleffner syndrome” in the literature leads to the difficulties in the understanding of concepts and terminology (137).
The role of prolonged epileptiform activity on neuropsychologic deficits remains unclear. It was initially hypothesized that a “functional ablation” of eloquent cortical areas was induced by the “persistent convulsive discharge,” resulting in neuropsychologic impairment. Epileptiform activity during sleep may also interfere with learning and the mechanisms of memory consolidation (138,139). Consequently, near continuous epileptiform discharges have been considered to underlie the severe neuropsychologic regression in EE-CSWS (140,141). While some authors reported a good correlation between the cortical location of interictal discharges and the disrupted function, others did not find any correlation (133,142). Moreover, the temporal association between the occurrence of CSWS on EEG and neurologic regression is not always strict (143,144).
In addition to a deficit in learning and memory consolidation mechanisms during sleep, there are probably several components involved in the neuropsychologic regression, including the cause of EE-CSWS, the effect of seizures on brain activity, and a deficit in information processing during the epileptiform activity. The role of polytherapy with multiple medications and high antiepileptic drug burden may also contribute to cognitive dysfunction and worsen the neuropsychologic deficit (145).
Connectivity studies by functional imaging provided some insights in the mechanisms of EE-CSWS. FDG-PET has shown areas with increased glucose metabolism corresponding to the epileptic foci and other hypometabolic cortical areas (142,146). It was, therefore, suggested that the epileptic foci affect other cortical areas by surrounding inhibition when the hypometabolic area is located at the border of the hypermetabolic area, or by remote inhibition when the hypometabolic area is distant from it. In some patients, the epileptic focus cannot be intense enough to be imaged by PET (142,146). At remission, regression of both focal hypermetabolism and hypometabolism is observed and functional connectivity between regions showing increased and decreased metabolism for glucose at the active phase of CSWS returns to normal, suggesting that in addition to the epileptic focus, the inhibited area plays a role in cognitive regression (146). The data from EEG-fMRI studies also support this hypothesis, showing an increase in perfusion in the epileptic focus and a decrease in perfusion in distinctive connected cortical areas (147,148). The adverse effect of interictal spikes on information processing has been described by investigators (149–151).
Animal models mimicking EE-CSWS are currently unavailable. However, experimental studies have explored how interictal epileptiform activities may impact the cognition. Interictal spikes cause transitory cognitive impairment in adult rodents (152). Interictal spikes during early brain development may have long-term adverse effects on the developing neural circuits. In particular, interictal spikes can have two types of effect on cognitive functions: transitory interruption inducing direct behavioral effects, and alteration of brain plasticity and memory processes (153). Transitory interictal spikes during development, induced by prefrontal intracortical injections of bicuculline in P21 rats, led to a deficit in attention and in sociability (154). Interictal spikes can be induced without seizure using low dose flurothyl in rat pups (155). When rats were tested as adults, there was impairment in spatial memory as well as impaired long-term potentiation (LTP). Moreover, the animals had a decrease in neurogenesis and in hippocampal cell counts (155).
A better understanding of the mechanisms of EE-CSWS might have a global impact on the understanding of cognitive involvement in many types of epileptic encephalopathies. There are typically few seizures in EE-CSWS while the cognitive regression is the major issue explaining why this syndrome could be a prototype of cognitive involvement of EEs. Identifying the neurobiologic mechanisms of EE-CSWS is a real challenge and needs the development of new animal models.
STATUS EPILEPTICUS
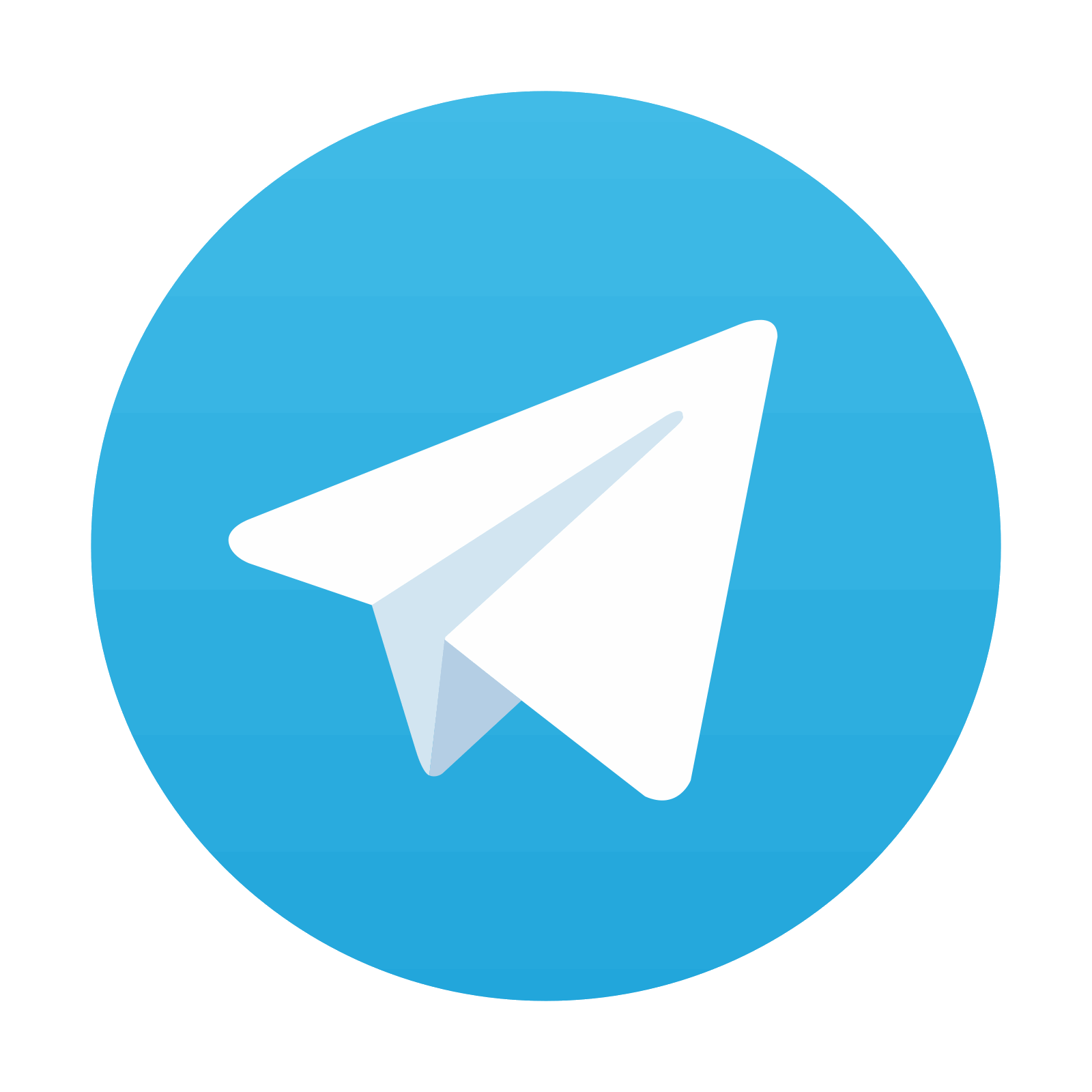
Stay updated, free articles. Join our Telegram channel
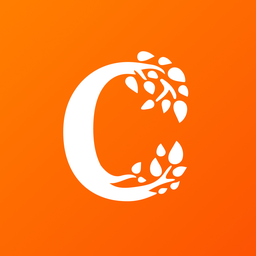
Full access? Get Clinical Tree
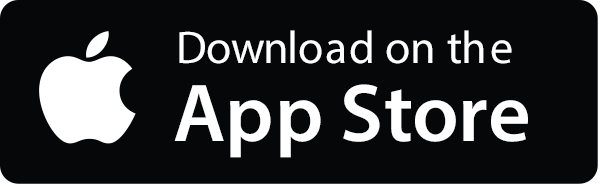
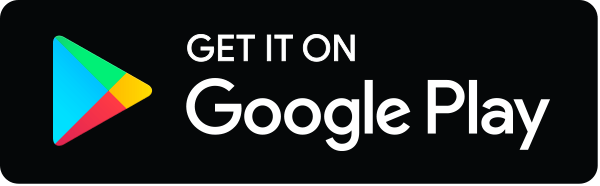