Pharmacokinetic interactions, sometimes leading to adverse clinical situations, have long been recognized as an occasionally unavoidable facet of antiepileptic drug (AED) treatment (1,2). Since the mid-1990s, there has been an exponential increase in the number of AEDs available, six of which have entered the U.S. market within the past 10 years. Referred to as either older or newer, with more recent AEDs available since 2009, some use the terminology first, second, and third generation. One general advantage of these newer medications is an improved pharmacokinetic profile, including a reduced potential for participating in drug–drug interactions, as compared to the older medications. This is significant because drug interactions may be a significant contributor to both patient morbidity and mortality (3,4).
The aim of this chapter is to update and review newly approved AEDs regarding drug interactions in terms of absorption, distribution, metabolism, and elimination. Particular importance is paid to mechanisms of interactions via hepatic enzyme induction, inhibition, and P-glycoprotein. Clinical implications of these interactions are also be discussed.
Clinicians should recognize that as a group, patients with epilepsy, including both children and adults, tend to receive more medications than does the general population. As the number of concomitant medications increases, so does the likelihood of drug interactions. The patients with the most refractory epilepsy are consequently more likely to encounter problems with drug interactions related to concomitant AED therapy than their controlled counterparts. Although, historically, more attention has been paid to AED-to-AED interactions, there has been increasing attention to the potential for certain AEDs to interact (perhaps adversely) with other medications that patients may be receiving. Even if noted, it can be difficult to quantify the impact of the interaction and its clinical importance, as there is much variability between dose, duration, clinical status, pharmacogenetics, diseases, and other medications in patients. Those at the greatest risk for drug interactions are usually those who are the most severely ill. This includes patients in the intensive care unit, geriatric patients, premature neonates, and young children. This is due to either the greater number of medications they may be receiving or potential liver or renal impairment in critically ill patients, as well as different pharmacokinetic parameters at the extremes of age.
MECHANISMS OF DRUG INTERACTIONS
Most clinically relevant drug interactions result from alterations in the pharmacokinetics of a drug, namely its metabolism, which takes place in the gut and liver. Other pharmacokinetic parameters that will be discussed later include absorption, distribution, and elimination. There are various mechanisms by which drugs are metabolized. They are Phase I (oxidation, reduction, hydrolysis), which rely principally on cytochrome P450 (CYP450) enzymes; and Phase II glucuronide conjugation via enzyme uridine 5′-diphospho-glucuronyltransferase (UGT) (5). Phase II reactions serve mainly as detoxifying reactions to produce, in most cases, inactive metabolites. Phase I reactions may also yield inactive metabolites, but in many cases will produce metabolites with at least partial pharmacologic activity of the parent molecule. Of the 15 CYP isoenzymes, CYP2C9, 2C19, 2D6, and 3A4 are responsible for the majority of oxidative metabolism of drugs, including AEDs, particularly CYP3A4.
Drug-metabolizing enzyme induction can result in an increased rate of metabolism of the affected drug, leading to both decreased oral bioavailability and increased systemic clearance of extensively metabolized concomitant medications. The clinical result therefore would be potentially subtherapeutic serum concentrations of that drug. Conversely, a number of drugs have been shown to be inhibitors of various drug-metabolizing enzymes, and concomitant administration of these agents can slow the rate of metabolism of the affected drug and cause increased serum levels of drug, leading to toxicity. Since new AEDs are first licensed as adjunctive therapy, there is a high likelihood of AED-to-AED interactions.
The metabolic pathways of AEDs can vary; however, most metabolism is achieved via oxidative metabolism and/or glucuronidation (6–8). Oxidative metabolism is accomplished via the cytochrome P450 (CYP) isoenzyme system. This system consists of three main families of enzymes: CYP1, CYP2, and CYP3. There are seven primary isoenzymes that are involved in the metabolism of most drugs: CYP1A2, CYP2A6, CYP2C9, CYP2C19, CYP2D6, CYP2E1, and CYP3A4. Of these, the ones commonly involved with metabolism of AEDs include CYP2C9, CYP2C19, and CYP3A4 (9). Moreover, CYP 3A4 metabolizes close to 50% of all drugs, which adds to the potential of interactions via this particular isoenzyme (10). Another important metabolic pathway for several AEDs, including valproic acid, lorazepam, and lamotrigine, is conjugation via the enzyme UGT.
These pharmacokinetic interactions can clearly complicate therapy in individuals receiving multiple AEDs. In some cases, it may be difficult to distinguish whether a change in a person’s clinical state (change in seizure frequency or appearance of toxicity) is due to an additive pharmacologic effect of the added drug or simply to a change in serum concentration in the original AED. One approach to rational polytherapy would be to combine agents that do not interact with each other. In this way, the confounders of changes in drug disposition can be excluded from the evaluation of therapeutic response to combined AED treatment. Although this may be ideal, avoiding combinations of AEDs is not always possible; therefore, monitoring of drug levels may be warranted in these circumstances. Interactions between AEDs and hepatic enzymes are summarized in Table 47.1 and discussed in the following paragraphs.
Hepatic Enzyme Induction
Compounds that are hepatic inducers increase the synthesis of enzyme protein and thus increase the capacity for drug metabolism. Induction of hepatic enzymes occurs over a gradual period of days to weeks and is a reversible process. Addition of an inducer will cause a lowering of serum concentrations of the target drug, conceivably resulting in inadequate therapeutic response. Conversely, removal of an enzyme inducer will cause a rise in the levels of the target drug, potentially causing toxicity.
TABLE 47.1
EFFECT OF ANTIEPILEPTIC DRUGS ON CYP ISOENZYMES OR OTHER ENZYME SYSTEMS | ||
Drug | Effect on Metabolism | Enzymes |
Phenobarbital, carbamazepine, phenytoin | Inducers | Broad CYP, UGT inducers |
Valproic acid | Inhibitor | CYP 2C19, UGT, epoxide hydrolase |
Gabapentin, pregabalin | No effect | — |
Lamotrigine | Weak inducer | UGT |
Levetiracetam | No effect | — |
Oxcarbazepine | Inducer (modest) | CYP3A4 |
Tiagabine | No effect | — |
Topiramate | Inhibitor (modest) Inducer (modest) | CYP2C19 CYP3A4 |
Vigabatrin | None | — |
Zonisamide | No effect | — |
Rufinamide | Inducer (weak) | CYP3A4 |
Lacosamide | No effect | — |
Clobazam | Inhibitor Substrate | CYP2D6 CYP2C19 |
Ezogabine | None | — |
Perampanel | Substrate Inducer (weak) Inhibitor (weak) | CYP3A4/5, 1A2, 2B6 CYP3A4/5, 2B6 CYP2C8, UGT1A9 |
Eslicarbazepine | Inhibitor (moderate) Inducer (weak) | CYP2C19 CYP3A4 |
Enzyme induction occurs by binding one or more chemical activators to the CYP isozyme receptor, which increases the ligand-activated transcription of CYP genes, ultimately leading to increased CYP protein (5). Although the degree of induction may differ among various CYP isoenzymes, they all involve activation of receptor transcription factors such as pregnane X receptor (PXR), constitutive androstate receptor (CAR), and aryl hydrocarbon receptor (Ahr). Because induction involves upregulation or synthesis of new enzymes, this process is not immediate, but rather gradual; it can take days to weeks to complete, dependent on the drug half-life.
Among the older-generation AEDs, carbamazepine, phenytoin, and the barbiturates (phenobarbital and primidone) are inducers of both the cytochrome P450 (CYP) and UGT enzyme systems (11). Combining these agents with other AEDs that are metabolized by either of these enzyme systems can result in markedly enhanced systemic clearance, and reduced serum concentrations of the affected drug, requiring higher doses in order to maintain comparable (as compared to monotherapy) steady-state serum concentrations. An example of this sort of interaction would be the combination of phenytoin and lamotrigine. Second- and third-generation AEDs are more appealing in their pharmacokinetic profile, with weaker inducing activity yet still clinically significant. Oxcarbazepine, eslicarbazepine, felbamate, rufinamide, topiramate (doses ≥200 mg/day), and perampanel (doses ≥12 mg/day) induce CYP and/or UGT isoenzymes (12). Patients on an enzyme inducer, particularly first generation, are at risk of decreased efficacy of concomitant medications, including AEDs, which can lead to loss of seizure control. Clinicians should be aware of which medications are CYP inducers, particularly when treating women of childbearing age, as this can reduce the efficacy of hormonal contraception (13).
Hepatic Enzyme Inhibition
Hepatic enzyme inhibition can occur when two drugs compete for the same enzyme site, reducing the metabolism of the target drug. A resultant increase in the object drug can occur if a substantial portion of the target drug is prevented from occupying the enzyme site. Inhibition is usually a rapid process that is dose/concentration dependent. Addition of an enzyme inhibitor may cause a very rapid rise in serum concentrations of the target drug, potentially leading to acute toxicity (6). In addition to competitive inhibition, which is reversible, irreversible inhibition may also occur. The so-called mechanism-based inhibition can occur if a drug forms an intermediate metabolite that forms a covalent bond with the CYP enzyme, resulting in a nonfunctional enzyme. These inhibitory reactions tend to be time-dependent, as onset will require formation of the reactive metabolite, and offset of the interaction will depend upon the turnover half-life of the CYP enzyme. A number of commonly used medications can result in mechanism-based inhibition of CYP 3A4. Common examples include erythromycin, clarithromycin, diltiazem, and verapamil (13).
In contrast to enzyme induction, inhibition of selected CYP and/or UGT enzymes can be caused by several AEDs of both the older and newer generations. These combinations may result in unexpectedly high serum concentrations of the affected AED. An example is the interaction of valproic acid and lamotrigine. Lamotrigine’s half-life is increased to approximately 59 to 70 hours when it is coadministered with valproate, resulting from valproate’s inhibition of glucuronidation. Inhibition of lamotrigine clearance can occur with valproate doses as low as 125–250 mg/day, and becomes maximal at dosages approaching 500 mg/day (14). The clinical implication is that lamotrigine dose and dose escalation will have to be substantially reduced in order to reduce the potential for adverse effects (including perhaps severe rash). An example of an interaction of a non-AED inhibitor and AED would be that of erythromycin, a CYP3A4 inhibitor, and carbamazepine, a CYP3A4 substrate, leading to potentially serious toxicity of carbamazepine. Likewise, if an inhibitor is withdrawn, lower serum concentrations of the target drug will be seen, as its metabolism will now revert to normal, if dose adjustments are not made preemptively.
In addition to valproic acid, other weak CYP2C19 inhibitors include oxcarbazepine, eslicarbazepine, topiramate, and felbamate. Any of these medications can result in a decrease in serum concentration of phenytoin, a CYP 2C19 substrate (12).
OTHER PHARMACOKINETIC PRINCIPLES
Other pharmacokinetic mechanisms for drug interactions, aside from metabolism, include absorption, distribution, and elimination.
Absorption
Absorption refers to the movement of drug from the site of administration to the site of action. The degree to which a drug administered reaches systemic circulation (bioavailability) is dependent upon absorption. AEDs are generally absorbed via passive diffusion, but can also involve transports, such as P-glycoprotein. Most AEDs are well absorbed following oral administration, and are not associated with many clinical interactions from this mechanism. However, absorption of some compounds can be altered by drug–drug or drug–food interactions. These interactions can affect maximum plasma concentration, time to reach maximum concentration, and even overall extent of absorption. Among the older, traditional AEDs, oral absorption of phenytoin appears to be the most problematic. Of particular concern is the issue of concomitant administration of an AED with an enteral nutrition supplement. Concomitant administration of phenytoin with these nutritional formulations can result in marked reductions in oral bioavailability (15–17). Because of this interaction, it is commonly suggested that the administration of phenytoin and enteral feedings be separated by at least 2 hours. Unfortunately, this may not be practical, particularly for patients requiring continuous feedings. Alternatively, clinicians can overcome this interaction by simply increasing the phenytoin dosage and using serum drug concentrations as a guide. This approach is also problematic. If, for example, enteral feedings are discontinued, or interrupted for a significant period of times, and phenytoin doses are not readjusted downward, there will likely be a marked rise in phenytoin concentrations, potentially leading to drug intoxication. If possible, therefore, this drug–nutrient interaction should be avoided. Concomitant ingestion of food may also delay the rate of absorption of older agents such as valproic acid, but this is unlikely to impact overall absorption (18).
Generally speaking, oral absorption interactions with the newer-generation AEDs are unlikely to be of clinical significance in most patients. This includes lacosamide, clobazam, ezogabine, perampanel, and eslicarbazepine. Oral bioavailability is more than 85% for rufinamide, eslicarbazepine, lacosamide, and perampanel, with ezogabine at 60%. Peak plasma concentrations are reached rapidly, ranging from 0.25 to 3 hours for the previously mentioned AEDs, with the exception of rufinamide, which takes 4 to 6 hours (19). Despite the fact that gabapentin and pregabalin are chemically very similar, structural differences can affect some pharmacokinetic properties. Gabapentin is actively absorbed via the L-amino acid transport system (System L). This can lead to saturable, nonlinear oral absorption. Unlike gabapentin, pregabalin does not display saturable oral absorption. This property seems to confer a greater degree of interpatient variability in absorption with gabapentin as compared to pregabalin (20). Clinically, this leads to more predictable pharmacokinetics (21).
Unlike older, traditional compounds such as phenytoin or carbamazepine, the newer-generation AEDs tend to be quite water soluble and are rapidly and completely absorbed. Indeed, in contrast to the problems described for phenytoin, absorption of gabapentin, lamotrigine, and levetiracetam does not appear to be impaired when coadministered with enteral nutritional supplements (22,23), and similarly is not expected to be impaired with any of the newer AEDs.
Of the newly approved AEDs or new formulations, the two that may have a drug–food interaction are extended-release oxcarbazepine and rufinamide. When oxcarbazepine is given with food, the mean maximum serum concentrations of the active monohydroxy metabolite is increased by 23% (24,25). Whether this is clinically meaningful is unclear. In contrast, the more recently available extended-release oxcarbazepine produces a higher peak plasma concentration (by 60%) and shorter time to peak under fed as opposed to fasting conditions, and therefore it is recommended to administer the medication on an empty stomach (1 hour before or 2 hours after a meal) to avoid peak-related side effects (26).
A number of studies have evaluated the effect of food on rufinamide. It was shown that food increases the maximum concentration, but without affecting the time to this concentration. It is noteworthy that administering rufinamide after periods of fasting may lead to lower plasma levels, and thus possibly lower seizure control (27). Although it is unlikely that patients will be fasting for periods of time prolonged enough to affect absorption, the manufacturer does recommend administering rufinamide with food.
Drug transporters may affect AED absorption, especially when being affected by an efflux transporter. ATP-dependent drug transporters, including members of the multidrug resistance protein (MRP) family and P-glycoprotein (Pgp), have been implicated as a major limiting factor in drug pharmacokinetics (28). Based on the location of Pgp, it plays an important role in the absorption, distribution, metabolism, and elimination of drugs. Pgp and MRP are located on the apical side of capillary endothelial cells and are thought to extrude drug molecules back into blood (or intestine) from cells. These efflux pumps appear to act in conjunction with drug-metabolizing enzymes such as CYP 3A4 to limit drug access to both the systemic circulation and various cellular compartments (29). This may be clinically important, in that several of the older AEDs, such as carbamazepine, display the ability to induce the activity of CYP 3A4 and Pgp (30). At the intestinal level, induction of both CYP 3A4 and these efflux pumps would serve to significantly reduce the oral bioavailability of a number of medications. While most attention has been focused on the role of these transporters in modulating oral drug absorption, it has also become clear that these transporter proteins are localized in a variety of tissues, including the liver, kidney, and placenta, as well as the blood–brain barrier. In addition to potentially limiting oral drug absorption or blood–brain barrier penetration, these drug efflux pumps may be important in protecting the fetus from drug or chemical exposure. Although not the focus of this chapter, it is hypothesized that repeat seizures may lead to overexpression of Pgp at the blood–brain barrier in patients with medically intractable epilepsy. This would lead to drug being transported out of the brain, causing lower drug concentrations at the desired site of action. AEDs that are substrates of Pgp include phenytoin, phenobarbital, lamotrigine, levetiracetam, topiramate, clobazam, and carbamazepine (31). Inhibitors of Pgp include verapamil and ketoconazole. In addition to seizures inducing Pgp, some AEDs have been shown in vitro to induce Pgp as well; those are phenytoin, phenobarbital, carbamazepine, and valproic acid, which were both dose- and time-dependent (31). There are overlapping substrates between CYP3A4 and Pgp, with drug interactions affecting both the enzyme and the efflux transporter. In addition, several studies have now demonstrated that Pgp is expressed in the trophoblast layer of the placenta and may provide an important mechanism of protection to the fetus from maternal drug exposure (32). Further studies are needed to fully understand the interactions with AEDs, Pgp, and refractory epilepsy.
Distribution
After an AED is absorbed, it has to distribute into the tissues, allowing it to reach the site of action. The main factors that play a role include volume of distribution (hydro- or lipophilicity), protein binding, and barriers, particularly the blood–brain barrier.
In most cases, changes in protein binding are not clinically significant, but in some situations these alterations, as a result of either changes in protein concentration (e.g., hypoalbuminemia) or protein binding displacement, may lead to misinterpretation of serum drug concentrations (32). Protein binding displacement interactions can occur when two highly protein-bound (>90%) agents are administered together and compete for a limited number of binding sites. Typically, the drug with the greater affinity for the binding site displaces the competing agent, increasing the unbound fraction of the displaced drug. It is the unbound drug concentration that is responsible for the drug’s pharmacologic activity. Unbound drug concentrations are dependent on the drug dose and drug-metabolizing activity of enzymes (intrinsic clearance). Unbound drug concentrations may rise initially following the concomitant administration of two competing drugs, but should return to preinteraction values fairly quickly. In other words, these interactions are transient, and largely clinically irrelevant. Total concentrations of drug, however, will be lower than expected. If serum concentrations are being monitored, this may lead to misinterpretation.
Among the AEDs, the potential for protein binding interactions is greatest for phenytoin and valproic acid. Both phenytoin and valproic acid are extensively bound to plasma proteins (>90%). Valproic acid is also an inhibitor of cytochrome P450 2C19, one of the enzymes responsible for phenytoin metabolism. When these two agents are coadministered, unbound phenytoin concentrations are higher than typically expected and total (bound + unbound) concentrations are lower (33). When using this combination, it may be prudent to monitor unbound phenytoin concentrations as well as total.
Among the newer-generation AEDs, several are extensively protein bound; these and include tiagabine (96%), ezogabine (80%), and perampanel (95%). To date, there is a paucity of experimental or clinical data regarding the impact of protein binding displacement with these medications. Being aware of the potential for displacement of one of these drugs is important when interpreting serum drug concentrations.
Elimination
Because of their primarily renal clearance, and absence of substantial hepatic metabolism, levetiracetam, gabapentin, pregabalin, and ezogabine are not subject to inhibition. In addition, none of these drugs appears to inhibit metabolism of any other medication. However, renal function does decline with age and can be impaired during times of critical illness. Estimating patients’ glomerular filtration rate through the various equations may be of benefit when patients are critically ill, to determine if dose adjustments are warranted. Similarly, dose or frequency for some AEDs may vary at the extremes of age due to the differences in renal function. One example is levetiracetam, clearance of which has been shown to be higher in neonates and infants compared to children and adults; therefore, more frequent dosing is recommended (34).
Lamotrigine is extensively (>90%) metabolized hepatically by N-glucuronidation via UGT 1A3 and UGT 1A4. Lamotrigine does not appear to significantly alter concentrations of carbamazepine or carbamazepine epoxide (9,35) nor any of the other AEDs. However, the half-life of lamotrigine is reduced from 24 hours to 15 hours when administered with enzyme-inducing drugs, as just described. Following the withdrawal of the enzyme inducers like carbamazepine and phenytoin, lamotrigine plasma concentrations have been observed to increase by 50% and 100%, respectively (36).
Levetiracetam shows limited metabolism in humans, with 66% of the dose renally excreted unchanged. Its major metabolic pathway is via hydrolysis of the acetamide group to yield a carboxylic derivative, which is mainly recovered in the urine. Levetiracetam is not significantly metabolized by CYPs or UGTs and appears to be devoid of pharmacokinetic drug interactions (37,38). Similarly, the drugs gabapentin and pregabalin appear to be devoid of enzyme-inducing (or inhibiting) properties.
Oxcarbazepine is converted to 10-hydroxycarbam-azepine (OHCZ), the metabolite primarily responsible for pharmacologic activity. This active metabolite is mostly excreted by direct conjugation to glucuronic acid. Oxcarbazepine does not seem to be a broad-spectrum enzyme inducer, although it does possess modest, specific induction potential toward the CYP3A subfamily, as evidenced by the increased metabolism of estrogens and dihydropyridine calcium channel antagonists (1,2). Clinicians should be aware that this drug does indeed have modest potential for causing enzyme induction interactions, but that this potential may vary among different patients.
Topiramate is approximately 60% excreted unchanged in the urine. It is also metabolized by hydroxylation and hydrolysis. Two of its metabolites are conjugated as glucuronides. While not considered a potent enzyme inducer, topiramate can increase clearance of valproate by approximately 13% and may lower oral contraceptive serum concentrations (39,40). Whether these changes in valproic serum concentration are clinically meaningful is unclear. Topiramate metabolic clearance can be increased when it is administered with enzyme-inducing AEDs, thereby reducing half-life and lowering serum concentrations by up to 40%.
Zonisamide is a synthetic 1,2-benzisoaxole derivative that is metabolized in large part by reduction and conjugation reactions. Oxidative reactions involving CYP3A4 and CYP2D6 are also involved. Zonisamide elimination can be altered by other drugs. Specifically, enzyme-inducing drugs such as carbamazepine and phenytoin can significantly increase the clearance of this drug, effectively reducing the half-life of zonisamide by about half.
Topiramate may decrease the clearance of phenytoin, suggesting inhibition of CYP2C19. Topiramate has been shown to increase phenytoin serum concentration in some patients. While this interaction is not clinically meaningful in most patients, given the nonlinear pharmacokinetics of phenytoin, the potential does exist for this interaction to result in phenytoin intoxication.
A significant advancement of oxcarbazepine over carbamazepine is its lack of susceptibility to inhibitory interactions. Consistent with its differing metabolism (as compared to carbamazepine), oxcarbazepine’s pharmacokinetics are not altered by erythromycin. Oxcarbazepine is a weak inhibitor of CYP2C19, however, and, like topiramate, it may increase the plasma concentrations of phenytoin (1).
Rufinamide is extensively metabolized in the liver via carboxylesterase-mediated hydrolysis of the carboxylamide group to CGP 47292, which is an inactive metabolite. It is not dependent on CYP450 enzymes for its metabolism, but has been shown to be a weak inhibitor of CYP2E1 and a weak inducer of CYP3A4 (18). The elimination half-life in adults is 6–10 hours. Although population PK analysis would not anticipate AED interactions, studies have shown a decrease in rufinamide plasma concentrations ranging from 14% to 46% for patients on concomitant carbamazepine, phenytoin, phenobarbital, primidone, methsuximide, oxcarbazepine, or vigabatrin. Clearance values of rufinamide decreased, meaning an increase in serum rufinamide concentrations occurred in patients receiving lamotrigine or valproic acid. These are generally clinically insignificant interactions, but there is the potential for toxicity, especially in patients who are already on higher doses.
Lacosamide exhibits moderate hepatic metabolism via demethylation to the major metabolite, O-desmethyl-lacosmide. It is a substrate of CYP3A4, 2C9, and 2C19 without any inhibitory or inducing effects. AED drug interactions are not expected, although a 15% reduction in the active metabolite of oxcarbazepine has been shown in patients receiving higher doses of lacosamide (400 mg).
Eslicarbazepine acetate is a pro-drug rapidly metabolized in the liver via hydrolysis to eslicarbazepine, the most active metabolite, and to a minor extent, R-licarbazepine, a less active species. These enantiomers are subsequently conjugated with glucuronic acid via UGT 1A4, 1A9, 2B4, 2B7, and 2B17 (18). The elimination half-life in adults is 10 to 20 hours, allowing for once-daily dosing. As a moderate inhibitor of CYP2C9 and 2C19, eslicarbazepine may interact with non-AED medications metabolized in this pathway. Due to the similar mechanism and active metabolite, use is not recommended with oxcarbazepine.
Clobazam is metabolized in the liver primarily via N-demethylation, mainly by CYP3A4 (with 2C19 and 2B6 to a lesser extent), to the active metabolite N-desmethylclobazam. CYP2C19 facilitates hydroxylation of the N-desmethyl metabolite. As a CYP2C19 substrate, up to fivefold increases in the active metabolite of clobazam can be seen if administered with a strong CYP2C19 inducer such as fluconazole or omeprazole (41). It is therefore recommended to avoid these medications and use an alternative agent.
Ezogabine (known as retigabine outside of the United States) exhibits moderate hepatic metabolism via hydrolysis/N-acetylation and glucoronidation via UGT1A1, 1A3, 1A4, and 1A9. The elimination half-life in adults is 8 to 10 hours, requiring three times daily dosing. Although metabolism does not rely on CYP enzymes, interactions may occur via UGT. Based on limited studies, ezogabine may increase phenobarbital and decrease lamotrigine plasma concentrations.
Perampanel undergoes extensive hepatic metabolism via oxidation (through CYP3A4/5) followed by glucuronidation. It is a weak inducer of CYP3A4/5. Given its extensive metabolism via CYP3A4, perampanel oral clearance is markedly increased when given concomitantly with enzyme-inducing AEDs such as phenytoin, carbamazepine, and oxcarbazepine. Although not specifically studied, similar interactions would be expected when given with eslicarbazepine. Given that concomitant treatment with enzyme inducers will reduce perampanel plasma concentrations, higher doses may be required for some patients in this setting (42).
Although inhibitory interactions might be expected when being coadministered with strong CYP3A4 inhibitors such as ketoconazole, studies to date suggest only modest effects on perampanel clearance. The likely explanation for this is that perampanel appears to be a somewhat weaker substrate for CYP3A4, which is evidenced by its very long elimination half-life (~100 hours).
INTERACTIONS BETWEEN AEDs AND OTHER MEDICATIONS
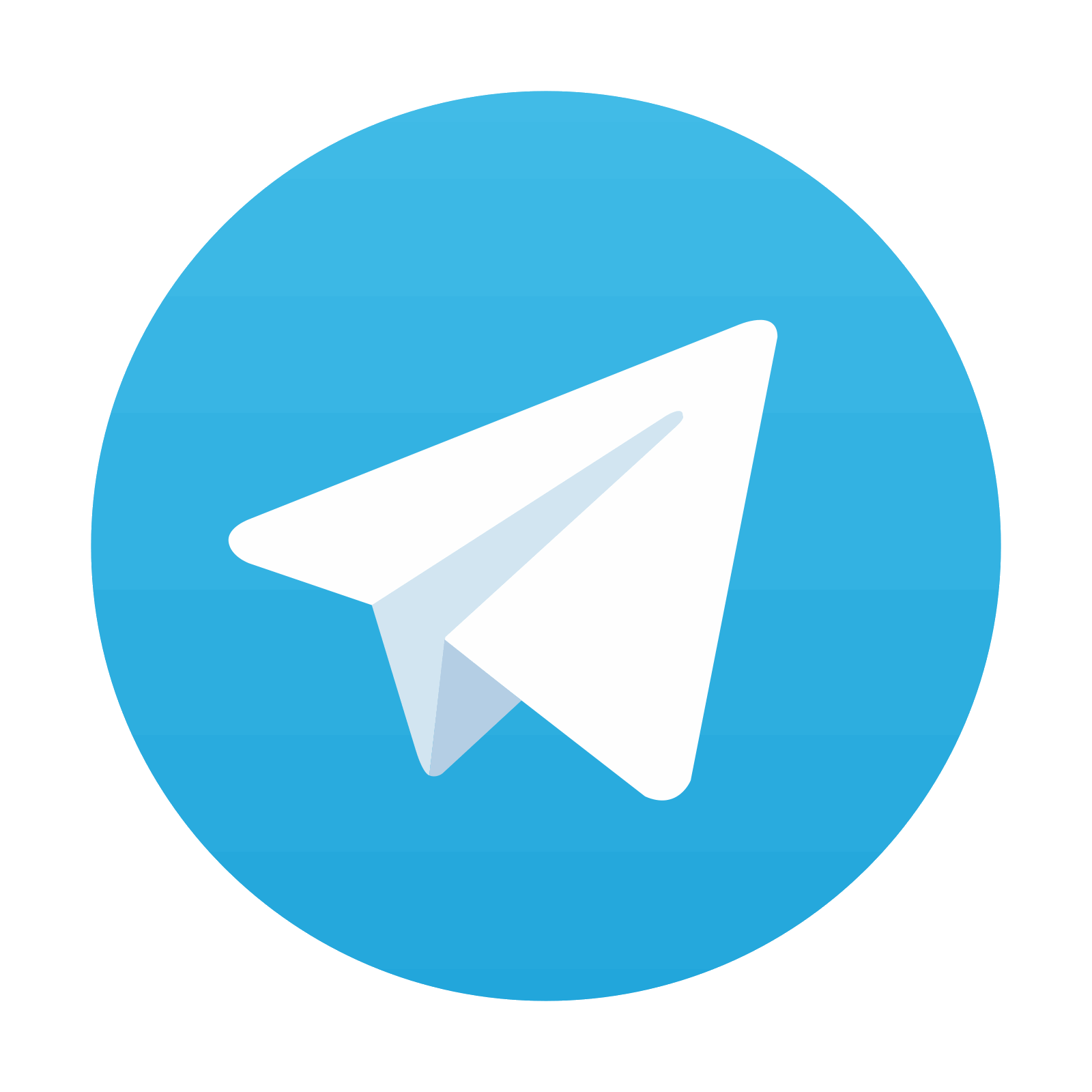
Stay updated, free articles. Join our Telegram channel
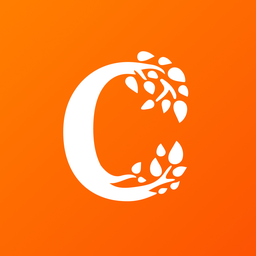
Full access? Get Clinical Tree
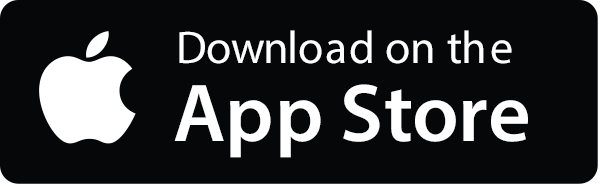
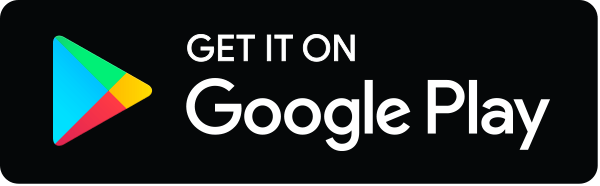