Neurology
Gary McCullagh, Dipak Ram, Nadya James
• Know the anatomy and embryology of the central and peripheral nervous systems
• Understand the role of cerebrospinal fluid (CSF) and how CSF analysis can be used for diagnosis of neurological conditions including infections
• Understand how the physiology of the central and peripheral nervous systems applies to neurophysiology (e.g. EEG, EMG)
• Understand the role of imaging in neurological disorders
• Know the genetic and environmental factors in the aetiology of important neurological disorders and brain development
• Understand the physiological and pathophysiological changes that occur in neurological disorders, including headache, migraine, raised intracranial pressure and epilepsy
• Know the pharmacology of agents commonly used in neurological disease
• Understand the basis of non-pharmacological treatments for the management of neurological disorders
Development of the central nervous system
During the third week of gestation, the three germ layers of the embryo are formed (ectoderm, mesoderm and endoderm; Fig. 28.1). The central nervous system appears at the beginning of the third week, being derived from the primitive streak. The cephalic end of this streak is known as the primitive node and surrounds the primitive pit. This process is called gastrulation.
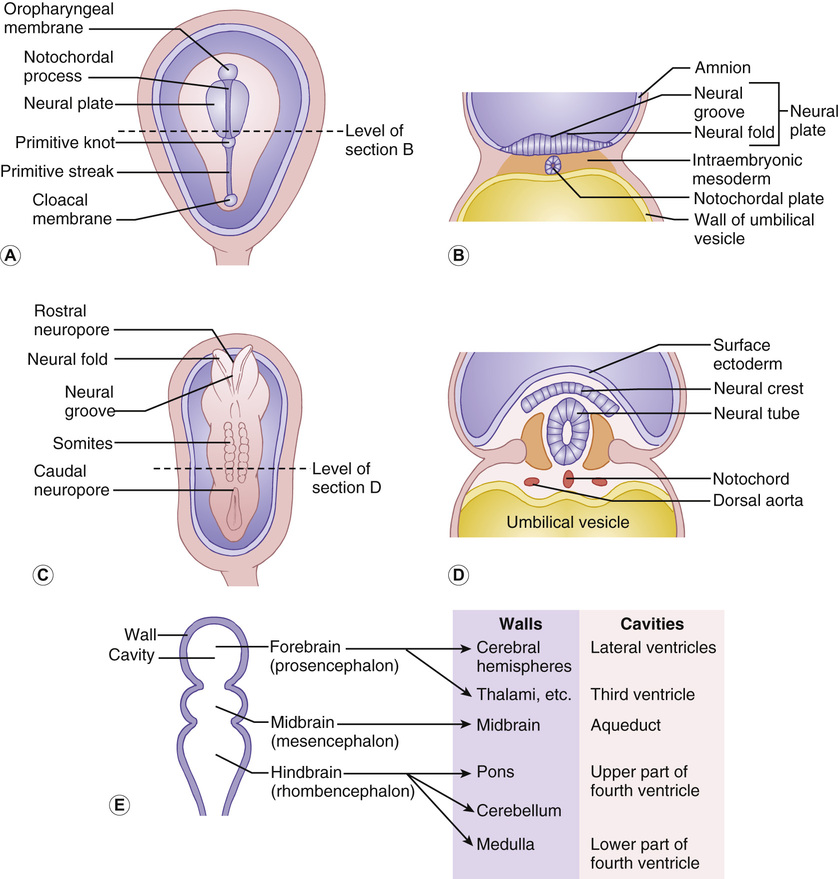
From the 3rd to 8th weeks, each of the three germ layers give rise to specific tissues and organs. This is the embryonic phase, following which the shape of the embryo changes markedly; the major external features are recognizable by the end of the second month.
Inside-out development
Primitive neural tissue arises from the ectoderm. Maturation of the primitive pit leads to the neural plate and neural folds. This is the basis of the nervous system. The neural folds become elevated and grow towards the midline to fuse and thus form the neural tube. The process of fusion begins in the cervical region and progresses both in the cephalic and caudal directions, with full closure being achieved at 25–27 days. The caudal end of the neural tube is composed of neuroepithelial cells, which give rise to primitive nerve cells known as neuroblasts, which following maturation form the grey and white matter of the future spinal cord. The cephalic end progresses through a process of folding to form the primitive hindbrain, midbrain, and forebrain.
The hindbrain, midbrain, and forebrain have distinct but interlinked functions, ranging from the hindbrain, which controls basic processes necessary to sustain life, to the intricacies of cerebral cortex activity in higher functions, such as completing a jigsaw puzzle or playing a musical instrument. Development in general begins with the ‘primitive’ brain and works upwards to the cortex. Therefore, it progresses first in the hindbrain, then midbrain, then forebrain. This is logical given that the need to eat and breathe is more important for survival than the ability to read and understand a newspaper.
Neural tube defects
Failure of the neural tube to form correctly leads to a variety of congenital defects:
• Midline defects – failure of fusion, e.g. of skull in encephaloceles, most often occipital, with herniation of meninges and may include neural tissue
• Spina bifida – by definition involves splitting of the vertebral arch, and may involve the spinal cord.
Spina bifida
A combination of genetic and environmental factors contribute to spina bifida. After having an affected infant, the risk of a second is 3–5%, and after two affected children have been born to a mother, the risk to the third is approximately 5–10%.
Drugs are known to have an effect. There is a 10–20 times increased risk in mothers taking valproate. The biological availability of folate to the fetus appears pivotal to the observed risk. The prevalence is reduced by maternal folic acid supplementation before and during early pregnancy, although in most people, the maximum effect is probably reached at small daily doses.
Cereal grain products are fortified with folic acid in the United States but not in the UK or most countries in Europe. There has been a marked decline in the prevalence of neural tube defects in the UK, due to improved maternal nutrition, folic acid supplementation and antenatal screening, mainly with ultrasound, and the option of termination of pregnancy. In the UK, 1.2 per 1000 pregnancies are affected; the birth prevalence is 0.2 per 1000 live births.
Genetic polymorphisms in genes that are involved in folate metabolism such as methylenetetrahydrofolate reductase (MHTFR) show strong associations with the risk of spina bifida and anencephaly. Polymorphisms (variations) in this gene are common in the general population, though, and most do not lead to abnormalities in the fetus. The exact mechanism of risk is not known but is likely to be related to the folate–homocysteine–methionine pathway.
Spina bifida comprises a spectrum of disorders:
• Spina bifida occulta – a defect in development of the vertebral arch, which is covered by intact skin and does not involve the spinal cord. The lumbar spine is the most frequent location and occurs in up to 10% of healthy people. In asymptomatic people, it is usually identified on incidental X-rays. If there is an overlying patch of hair or naevus or other skin abnormality, tethering of the spinal cord may occur during childhood. An ultrasound or MRI scan of the spine should be performed and a neurosurgical opinion obtained.
• Spina bifida cystica – more severe neural tube defect in which spinal cord tissue and/or meninges protrude through the skin. Most cases involve the lumbar spine. Includes meningocele and myelomeningocele:
– Meningocele – the fluid filled meninges, but not the spinal cord, protrude through the defect
– Myelomeningocele – neural tissue is involved and protrudes.
These lesions are often associated with downward displacement of the cerebellar tonsils through the foramen magnum, called the Chiari malformation (also known as Arnold–Chiari malformation), which may cause non-communicating hydrocephalus.
Cell differentiation
Once the basic three-region structure of the brain is completed, it must be populated with active brain cells. Precursors of brain cells are the pluripotent neural stem cells. These differentiate into neurons, astrocytes, and oligodendrocyte lineages:
– comprise a cell body (soma), dendrites and a single axon that terminates in one or more synapses
– rely on metabolically active ion channel pumps to maintain voltage gradients
– action potentials arise via voltage-gated ion channels
– dominant cell line in the brain and spinal cord
– maintain extracellular ion balance
– provide nutrients to neural cells
– support and insulate the axon
– permit saltatory conduction of nerve impulses (propagation of action potentials along myelinated axons from one node of Ranvier to the next)
– increase conduction velocity along neurons
Cell migration
Neural cells migrate to their correct locations along specially created ‘highways’ of glial cells, known as radial glia. Each layer of brain cells is laid down, and then forms a scaffold upon which the next layer can be built on top – in other words, the brain develops ‘inside out’. When this process is complete, the cortex has six distinct layers of organization. This amazingly complex system can, and does, go wrong (Table 28.1). Neuronal migration disorders can be visible on MRI scan (for example, lissencephaly – lack of development of brain folds) and are associated with a wide spectrum of clinical difficulties, including some forms of epilepsy.
Synaptogenesis
Synaptogenesis is the creation of functional signalling mechanisms between neurons. It ‘explodes’ at around 20 weeks’ gestation, and continues for the first couple of years after birth. In the central nervous system, a projection from a dendrite (a filopodium) makes contact with an axon. This contact triggers the axon to recruit synaptic vesicles and active proteins to the area, which now forms the presynaptic membrane. Neurotransmitter receptors gather in the membrane of the dendrite, i.e. the postsynaptic membrane. Both cells now express a protein called N-cadherin, which stabilizes the synapse. This now matures into the familiar arrangement, with a presynaptic (axonal) and postsynaptic (dendritic) membrane, separated by the synaptic cleft. A myelin sheath forms around thestructures, and the synapse is complete.
Myelination
Neuronal cells consist of the cell body, branching dendrites that receive information from surrounding cells, and long axons to carry information in the form of electrical impulses. Many axons are covered in myelin, produced by glial cells. Myelin can be thought of as being like insulation on an electrical wire; it increases the speed and accuracy of the transmission of information in the form of action potentials that propagate along neurons. The process of myelination begins in utero, and between 36 weeks and term myelin increases from 1% to 5% of total brain volume.
Normal myelination progresses in a set sequence, beginning around birth and infancy with the basic senses of sight, smell, taste, touch and sound. From then onwards into adulthood, myelination continues in areas of the brain linked to thought, emotion, executive planning, and conceptualization. Just like other aspects of neuronal maturation, a child’s experiences and learning influence the rate and growth of myelination.
Ion channels and the action potential
As nerve cells rely on voltage gradients to produce an action potential, the nerve cell must maintain a membrane potential by modulating the concentration of intracellular ions. In a resting state, a nerve cell has high intracellular potassium and low intracellular sodium. The voltage gradient is maintained by two mechanisms:
2. An adenosine triphosphate-dependent (ATP) pump actively transports three positively charged sodium ions (Na+) out of the cell in exchange for two positively charged potassium ions (K+). This energy-dependent process leads to a voltage differential across the cell membrane of around −70 mV, and a high potassium concentration inside the cell relative to outside.
In order to depolarize, ion channels in the cell membrane must activate and trigger ion redistribution. When neurotransmitter molecules bind to receptors located on a neuron’s dendrites, voltage-gated ion channels open. Positive ions enter the target neuron and depolarize the membrane, decreasing the difference in voltage between the inside and outside of the neuron. When this reaches the threshold potential (−55 mV), Na+ channels open. Na+ rapidly enters the cell and the neuron completely depolarizes to a positive membrane potential of about +40 mV. The action potential travels down the neuron as more Na+ channels open.
Once an action potential has been generated, the Na+ ion channels close, and the cell is refractory to any further stimulus. Voltage-gated K+ channels open, and K+ ions leave the cell. This takes the membrane potential down below the original −70 mV (hyperpolarization), resetting the Na+ channels ready for the next signal. The cell then ‘resets’ itself by once again pumping out Na+ in exchange for K+ and returns to a voltage differential of around −70 mV.
Ion channels are of clinical relevance in certain diseases and also as targets for therapeutic drugs. As well as the voltage-gated ion channels mentioned above, channels may be ligand-gated (activated by ligand binding), mechanosensitive (activated by stretch and mechanical forces), cyclic-nucleotide-gated (activated by substances such as cAMP), light-gated (in the eye), and temperature-gated.
Conditions known to be caused by ion channel malfunction include: many of the epilepsies, including generalized epilepsy with febrile seizures plus (GEFS+), Dravet syndrome (SCN1A mutation), familial hemiplegic migraine, and some of the ataxias. Drugs affecting ion channels include lidocaine (a local anaesthetic, blocks sodium channels), phenytoin and carbamazepine (antiepileptics, block sodium channels), ondansetron (an antiemetic, ligand binding to 5-HT3 receptors), lamotrigine (an antiepileptic, ligand-gated channels) and gabapentin (an antiepileptic, ligand binding to inhibit calcium channels).
Cell growth and apoptosis
Neuronal cell growth depends on many things, including the hormone nerve growth factor (NGF). This protein, and other cell-signalling protein known collectively as ‘neurotrophins’, are responsible for the growth, maintenance, and survival of neurons. NGF has a role in myelin formation and also inhibits cell apoptosis. The precursor of NGF (pro-NGF) is also important in its own right. Although not biologically active in itself, in combination with tumour necrosis factor type receptors it can either promote cell growth or conversely promote apoptosis.
Cell apoptosis or ‘programmed cell death’ is an essential part of the reorganization of the brain that occurs during growth and development of the child (see Neuronal plasticity, below). NGF is thought to have a role in many neurological conditions, including psychiatric and neurodevelopmental disorders, and to be a potential therapeutic agent for neurodegenerative conditions and multiple sclerosis.
Craniocaudal and centrifugal development
Once the basic structure of the nervous system is formed, the development of skills and abilities follows a craniocaudal (head to tail) and centrifugal (central to peripheral) sequence. This can be clearly seen in the way infants gain motor skills. First to emerge are essential skills, such as coordinated suck and swallow, then head control, then shoulder and upper trunk stability to reach for toys, followed by hip stability and sitting, then lower limb control for standing and walking. Peripheral skills, such as fine motor coordination to use a tripod grasp and draw pictures, come later than the central skills, such as stabilizing the shoulders to allow the arm to push their dinner plate off the high chair.
Neuronal plasticity
When first developing, the human brain divides into two distinct hemispheres, linked via a large bundle of fibres called the corpus callosum. Initially, the two halves are mirror images of each other, and share functions. Over time, functions begin to lateralize to one side or the other, and ‘handedness’ develops. The ability to change and strengthen neuronal pathways is known as ‘plasticity’. Put simply, pathways that are used grow stronger, and those that are not used will diminish and may disappear altogether.
Figure 28.2 illustrates lateralization relating to hand function. Initially, the right hand is supplied by motor fibres from both hemispheres. Around the time of birth and shortly afterwards, contralateral fibres from the left cerebral hemisphere begin to dominate, and the ipsilateral pathway from the right hemisphere diminishes. This explains why brain damage at different stages of development produces different clinical outcomes. If, for example, an infant suffers a left hemispheric stroke early in utero, the right hand is still supplied by both sides of the brain at that time. The left side simply takes over control of both hands, and the infant retains bimanual function. If the same injury occurs in the postnatal period, there may still be some capacity for ipsilateral pathways to develop. The right hand will be weak, but may have some function. In a young adult, the same stroke will eliminate the contralateral innervation of the right hand, and with no remaining ipsilateral fibres to utilize, function is lost. Fortunately, even adults may still have some residual ipsilateral fibres; people who have less hand preference or who have bilateral innervation show greater scope for recovery of function.
When developing, neurons have a wealth of synaptic connections. By age 3, the brain has around 1000 trillion synapses. Synaptic connectivity is maximal in early childhood and it is at this time that the brain is most receptive to the accumulation of new skills and knowledge, and maximum learning is possible.
Neuroplasticity is of utmost importance in visual development. As demonstrated by the experiments of Hubel and Wiesel (who famously studied visual cortex firing in cats): without binocular visual stimulation, anatomical abnormalities develop in the ocular dominance columns of the lateral geniculate nucleus and visual cortex. Visual deprivation in the first 3 months of life in infants (the critical period of visual neuroplasticity) may result in permanent visual impairment (amblyopia). The neonatal (performed within 72 hours after birth) and GP (at 6–8 weeks) eye screening checks should detect and enable the management of ocular abnormalities, such as cataract, within this period. The higher visual pathway continues to develop and differentiate over the first eight years of life (the sensitive period of visual neuroplasticity); visual deprivation, strabismus (squint) or uncorrected refractive error during this period will result in amblyopia.
Young children have more capacity for plasticity than adults, and can make remarkable recovery from significant brain injuries. However, the true extent of the damage may not become known for many years, when ongoing maturation ‘unmasks’ a neurological deficit. An example is traumatic brain injury, when the child may seem to make a full recovery in terms of returning to previous level of function, only to show significant cognitive difficulties when reaching adolescence. Similarly, it is easier to regain a function lost as a teenager than develop it from scratch as a toddler; for example, a concert pianist who has had a stroke will find it easier to learn to play again than a similar stroke sufferer who has never touched a piano.
Although synaptic pruning means that connections are lost throughout puberty and is complete soon after the end of puberty, learning and therefore a degree of plasticity is possible throughout life and into old age. Furthermore, an older person has the benefit of experience and has pre-established pathways that can augment both learning and speed of cognition.
The blood–brain barrier
The brain and spinal cord are uniquely protected by a physical barrier that isolates them from biochemical changes in the rest of the body. This shield is known as the blood–brain barrier (BBB). Not to be confused with the interface between cerebrospinal fluid (CSF) and the brain, the BBB lies along the individual capillaries that feed deep into the brain tissue itself. It is formed by closely sealed endothelial cells in cooperation with brain astrocytes, and regulates and adjusts transfer of nutrients, ions, proteins, and components of the immune system. It achieves this by highly selective permeability and use of specialized transporters. Glutamine differs from other amino acids in that it passes across the BBB through such a mechanism. Facilitative carriers for glutamine and glutamate at the luminal membrane may provide a mechanism for removing nitrogen and nitrogen-rich amino acids from brain.
Some molecules are able to freely cross the BBB: water, gases, and also some lipid-soluble substances. Increased BBB permeability for ammonia is considered to be an integral part of the pathophysiology of hepatic encephalopathy. This permeability to lipid-soluble agents is clinically important. In the jaundiced neonate, unconjugated bilirubin is highly lipid-soluble. As such, it passes readily across the BBB and into the brain, leading to neurological damage and kernicterus. Exposure to light of a specific range of wavelengths converts the bilirubin to a water-soluble state, by converting insoluble bilirubin into water-soluble structural isomers. It also generates bilirubin molecules in an excited state, and these react with oxygen to produce colourless oxidation products. Once the molecules are water-soluble they can no longer cross the BBB, and thus no longer damage the brain. Lipid solubility is also clinically relevant when prescribing. Agents that are lipid soluble are more likely to cross the BBB and alter CNS function. This may be desirable, for example when giving a general anaesthetic, but may lead to unwanted effects of sedation or brain disturbance with other drugs.
The BBB is susceptible to damage, in particular from inflammation in conditions such as meningitis. This is mediated by pro-inflammatory cytokines, and leads to opening up of spaces between endothelial cells and loss of the tight regulation of transport across the BBB. This can be advantageous (for example, allowing white blood cells and immune components to enter the brain), but can also lead to chemical imbalances and cell damage. In meningitis, however, we capitalize on the fact that the BBB is no longer preventing entry of chemicals into the brain, as it permits a far greater penetration of prescribed antibiotics (including the water-soluble amoxicillin) than would ordinarily be possible.
Anatomy and physiology of the nervous system
Knowledge of the structure and physiology of the nervous system allows understanding of how damage to different areas may present. The general structure of the brain is shown in the sagittal view in Figure 28.3.
At the spinal level, 31 pairs of mixed motor and sensory nerve bundles emerge (Fig. 28.4):
These nerves further combine to produce nervous plexi:
• Cervical plexus (C1–C4): muscles of the neck, shoulder and skin, phrenic nerve (diaphragm)
• Brachial plexus (C5–C8 + T1): muscles from the base of the neck to the fingertips and skin
• Lumbar plexus (L1–L3 + part of L4): skin and muscles of the lower abdomen, thighs and groin
• Sacral plexus (L4–L5 + S1–S4): muscles and skin of the pelvic area and legs
• Coccygeal plexus: muscles and skin of pelvic area and sphincters
Cerebrospinal fluid
Distinct from the BBB, the CSF–brain barrier relates to the extracerebral fluid that is found within the ventricles and around the brain. It has two main categories of function: physical and biochemical. From a physical standpoint, it acts to cushion and protect the brain from shear forces and impact, and plays a role in regulating intracerebral blood pressure and thus prevents ischaemia. Biochemically it serves to remove waste and toxins from the CNS, and helps regulate levels of hormones and neurologically active substances.
CSF is produced by a type of glial cell called an ependymal cell. It is chiefly produced in the choroid plexi in the lateral ventricles of the brain, and exits through the intraventricular foramen of Munro, into the third ventricle, through the aqueduct of Sylvius into the fourth ventricle, then down the spinal cord and over the cerebral hemispheres. It is reabsorbed into the circulation via arachnoid villi. CSF is produced at a rate of around 30 mL/hour. The volume after the age of 2 years is around 150 mL, with about 35 mL in the ventricular system. Abnormalities of the CSF circulation may result in hydrocephalus:
• Communicating hydrocephalus – no obstruction between ventricles and subarachnoid space. Caused by:
– Excessive CSF production (rare, e.g. choroid plexus tumour)
– Impaired CSF resorption (e.g. blockage of arachnoid granulations by debris after meningitis or haemorrhage)
• Non-communicating hydrocephalus – physical obstruction between ventricles and subarachnoid space. Caused by:
– Congenital malformation (e.g. aqueduct stenosis, Arnold–Chiari malformation)
Idiopathic intracranial hypertension is a special case where the CSF is elevated in the absence of hydrocephalus or intracranial mass lesion, and is described later in this chapter.
Treatment of hydrocephalus depends on the cause. It may be via resection of an intracranial obstruction, placing of a stent in a stenosed aqueduct, or by removal of excess CSF. In many children this requires insertion of a one-way valved ventriculoperitoneal shunt, which forms a direct drainage route for CSF from the cranial vault to the low pressure of the peritoneal cavity.
Clinical features of hydrocephalus depend on the site of the obstruction and also on the capacity of the cranial vault to expand if the sutures are not yet fused. Symptoms of hydrocephalus may be acute (vomiting, irritability, headache, change in consciousness) or chronic (visual disturbance, ‘sunsetting eyes’, early morning vomiting, pressure headache, deterioration in school performance). Untreated, acute hydrocephalus (for example, in a child with a blocked ventriculoperitoneal shunt) can lead to brainstem herniation and death. It is a neurosurgical emergency.
CSF analysis is of value in many disorders, the most common of which is meningitis (Table 28.2). It is also of value in diagnosis and management of metabolic disorders, leukaemias, neurodegenerative conditions and autoimmune disorders.
Table 28.2
CSF analysis values in diagnosis of meningitis*
White cell count | Biochemistry | |||
Neutrophils (× 106/L) | Lymphocytes (× 106/L) | Protein (g/L) | Glucose (CSF : blood ratio) | |
Normal (>1 month of age) | 0 | ≤5 | <0.4 | ≥0.6 (or ≥2.5 mmol/L) |
Normal term neonate | <5 | <20 | <1.0 | ≥0.6 (or ≥2.5 mmol/L) |
Bacterial meningitis | 100–10,000 (but may be normal) | Usually <100 | >1.0 (but may be normal) | <0.4 (but may be normal) |
Viral meningitis | Usually <100 | 10–1000 (but may be normal) | 0.4–1 (but may be normal) | Usually normal |
TB meningitis | Usually <100 | 50–1000 (but may be normal) | 1–5 (but may be normal) | <0.3 (but may be normal) |
Meningitis
CSF microscopy (see Table 28.2) may initially be normal in meningitis, so clinical impression is of prime importance. It is unusual to find neutrophils in CSF beyond the neonatal period, so this should raise the possibility of bacterial infection. CSF white cell count and protein level are higher at birth than in later infancy. In the first week, 90% of well babies have a white cell count less than 20, and a protein level <1.0 g/L. Although lymphocytes are more characteristic, neutrophils may predominate in viral meningitis even after the first 24 hours, which may make it difficult to distinguish between viral and bacterial meningitis. Antibiotics do not significantly change the CSF cell count or biochemistry in samples taken within 24 hours of the initial dose. Meningococcal PCR testing on CSF samples is particularly useful in patients with a clinical picture consistent with meningococcal meningitis but who have received prior antibiotics.
CNS inflammatory diseases
CSF IgG may be raised in patients with central nervous inflammatory diseases (e.g. multiple sclerosis, subacute sclerosing panencephalitis). The most commonly used diagnostic tests for multiple sclerosis are a raised CSF index (the ratio of CSF IgG to CSF albumin compared to the serum IgG to serum albumin ratio) and oligoclonal band detection.
CSF neurotransmitter disorders
The monoamine neurotransmitter disorders are caused by defects in the synthesis, degradation, or transport of dopamine, noradrenaline (norepinephrine), adrenaline (epinephrine), and serotonin. They are implicated in a varied group of conditions including mitochondrial disorders, Rett’s syndrome and leukodystrophies. They usually present with abnormal neurological features (encephalopathy, epilepsy, and pyramidal and extrapyramidal motor disorders). Their diagnosis may include analysis of neurotransmitters in the CSF, which involves a precise protocol for collection and rapid freezing of samples. Specialized laboratory analysis is required.
History and examination
History
History is the cornerstone of neurological diagnosis. Particular attention should be paid to the age at onset, developmental history including age of milestones, any loss of skills or deterioration of school performance, detailed family history, and the impact of the problem on family life and functioning. Typically, presentation falls into one of the following categories:
• Developmental delay – single domain or multi-domain
• Developmental regression – e.g. Rett’s syndrome
• Weakness – e.g. muscular dystrophy
• Abnormal movements – e.g. ataxia
• Change in level of consciousness – e.g. encephalopathy
• Paroxysmal events – e.g. epilepsy
Examination
A full neurological examination is a skilled and lengthy procedure, and is not required unless a neurological disorder is suspected. In those who do have a neurological symptom, your history will allow you to focus your attention on a specific part of the neurological system, with a more generalized exam of the rest of the child. Detailed neurological examination can be tricky in children, as you need a cooperative patient! However, it is usually possible to gain a good assessment of ability through a combination of observation of play and use of ‘games’ to encourage the child to do what you need. For example: a child may not cooperate with a ‘finger–nose pointing’ test, but is likely to join in with a ‘sweetie–mouth’ test if you hold out a pretend sweet for them to grasp. A child may not want to show you their gait or to demonstrate shoulder abduction for you, but is likely to join in a ‘race’ or to ‘flap your elbows like a chicken’.
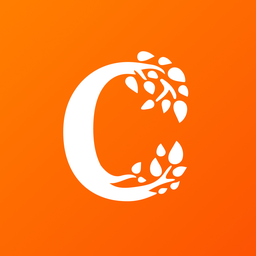
Full access? Get Clinical Tree
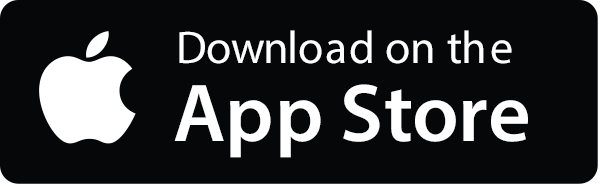
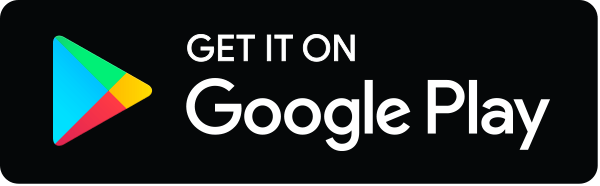