-
Chapter Outline
-
Part 1: Examination of the nervous system 1067
-
Introduction 1067
-
Examination systems 1068
-
Scheme of neonatal neurological examination 1068
-
Neonatal neurological alarm signals 1075
-
Special senses 1075
-
Imaging 1076
-
Examination of cerebrospinal fluid and intracranial pressure 1076
-
Electroencephalography 1076
-
Maturation of EEG 1076
-
-
Neurological examination before discharge, and follow-up 1076
-
-
Part 2: Neonatal seizures 1080
-
Introduction 1080
-
Pathophysiology 1081
-
Incidence 1081
-
Time of onset 1082
-
Diagnosis and classification 1082
-
Physiological changes during seizures 1084
-
EEG diagnosis of seizures 1084
-
Electrographic seizures 1087
-
Electroclinical dissociation 1087
-
Aetiology 1087
-
Metabolic causes 1089
-
Congenital malformations of the brain 1089
-
Inborn errors of metabolism 1089
-
Syndromic neonatal seizures 1090
-
Epileptic encephalopathies 1091
-
Ohtahara syndrome (early infantile epileptic encephalopathy) 1091
-
-
Early myoclonic epilepsy (EME) 1091
-
-
Part 3: Intracranial haemorrhage and perinatal stroke (arterial and venous) at term 1099
-
Introduction/background 1099
-
Incidence/prevalence of intracranial haemorrhage 1099
-
Risk factors for intracranial haemorrhage 1100
-
Clinical presentations of intracranial haemorrhage 1100
-
Investigations 1101
-
Pathogenesis of subdural haemorrhage 1102
-
Mechanisms of subdural haemorrhage 1102
-
Clinical features of subdural haemorrhage 1103
-
Clinical management following intracranial and subdural haemorrhage 1103
-
Extradural haemorrhage 1103
-
Subarachnoid haemorrhage 1104
-
Intraventricular, intraparenchymal, thalamic and cerebellar haemorrhage 1105
-
Subgaleal (subaponeurotic) haemorrhage 1106
-
Perinatal stroke 1107
-
Thrombophilic state and the newborn 1108
-
Perinatal arterial ischaemic stroke 1108
-
Cerebral sinus venous thrombosis 1109
-
-
Part 4: Hypoxic–ischaemic brain injury 1114
-
Part 5: Preterm brain injury 1156
-
Part 6: Hereditary and degenerative central nervous system disease 1182
-
Part 7: Muscle disease in the newborn 1189
-
Introduction 1189
-
Clinical presentation 1189
-
Obstetric history 1189
-
Clinical investigations 1192
-
Congenital myotonic dystrophy 1193
-
Congenital muscular dystrophy 1193
-
Forms of congenital muscular dystrophy with structural brain changes and eye involvement 1193
-
Motor neuron disorders 1195
-
Congenital myopathies 1195
-
Myasthenia gravis 1197
-
-
Part 8: Central nervous system malformations 1200
-
Introduction 1200
-
Epidemiology of CNS malformations 1200
-
Cephaloceles 1200
-
Neural tube defects 1201
-
Disorders of prosencephalic development 1205
-
Malformation of cortical development 1207
-
Ventriculomegaly 1213
-
Posterior fossa abnormalities 1214
-
Arachnoid cysts 1218
-
Cerebrovascular malformations 1218
-
Vein of Galen malformation 1219
-
-
Examination of the nervous system
- Janet M Rennie
- Angela Huertas-Ceballos
Introduction
Suspicion of neurological illness in the neonatal period is always important. As in any organ system disease, diagnosis and prognosis must be based on the findings of a full examination together with the results of appropriate investigation. Neonatal neurological assessment should include sequential careful examinations, which of necessity are dominated by examination of the baby’s state of alertness and the motor system. Neurological examination of a baby receiving intensive care is difficult, but not impossible, and can be supplemented by a wide range of diagnostic tests. Cranial ultrasound scanning is now standard in neonatal units, and atlases have been published which can help clinicians interpret the findings ( ; Govaert and de Vries 2010). Much valuable experience has been gained with magnetic resonance imaging scanning of the neonatal brain ( ; ). Normal ranges exist for somatosensory, auditory and visual evoked potentials, and recording the electroencephalogram (EEG) can prove extremely helpful. The information in this short chapter can be supplemented by further reading from more detailed texts ( ; ; ; ). Much of the modern work on examination of the neonatal nervous system has built on that of the earlier teaching of , and . For advice on general examination of the newborn, see Chapter 14 .
Examination systems
The endless fascination which observing babies provides is reflected in the number of schemes which have been developed for assessment of the neonatal nervous system. reviewed nine different systems, many of which require special training and take up to an hour to carry out. This is not practical in clinical practice, or possible in sick babies. The recommendations vary from a detailed ‘cut-down’ version of an adult-style neurological examination ( ) to a more general approach involving scoring of videos of general spontaneous movement ( ; ) or scoring of behavioural states ( ). We agree with that the allocation of a numbered score implies a degree of standardisation and accuracy which does not exist.
Our own current system is illustrated in Figure 40.1 , and can be carried out quickly and adapted for babies in intensive care. This scheme includes many of the items from the original Dubowitz system ( ) updated by Eugenio , which has been developed after a great deal of careful research, and was designed to be taught to junior resident staff and to be reproducible and quick to carry out. The updated scheme takes into account the newer emphasis on the importance of hand posture and presence or absence of individual finger movements, and has reduced duplication. In addition to completing a suitable proforma, there is no substitute for a short and pithy narrative note. For example:


At the start of the examination, Tom was lying prone in his incubator in a flexed posture with his knees tucked up under his abdomen, eyes closed and breathing regularly. His head circumference was 35 cm and his fontanelle felt normal. When stroked lightly on his back he awoke and began fussing, with normal facial and body movements and a normal cry, but he soon calmed when stroked again. He sucked vigorously on a finger with a stripping action of his tongue, made eye contact readily and followed a target horizontally through 90° in each direction with ease. When observed lying on his back he kicked with both legs, and his truncal and limb tone and reflexes were normal. His hands were relaxed and open and he made individual movements of his fingers. He could not be taken out of the incubator to test stepping or ventral suspension today but his Moro response appeared full and symmetrical, although it was not possible to test head lag. As far as I can assess, Tom’s neurological state was normal today. Alice’s head circumference is small for a term baby at 32 cm, and her ears appear prominent – they are not low-set. Her skull is a normal shape and, although her fontanelle was small (little-fingertip size), the tension felt normal. When observed at the start, Alice was lying on her back, with outstretched arms and extended legs; she had a high-pitched scream and was arching her back at times, digging her heels into the cot mattress. Her hands were fisted with the thumbs in both palms all the time and I did not observe her to open them. She could be consoled by swaddling and rocking but this took patience, and when she was handled her tone was high in both the trunk and the limbs. After some persuasion Alice fixed on a red woolly ball but did not track it consistently or through a wide range of arc. Alice did not make eye contact with me; she chomped on my finger and I note that the nurses are tube-feeding her at the moment. Tendon reflexes were easy to obtain but I could not elicit a stepping or root reflex and her Moro was incomplete with a brisk adduction phase. I am concerned about Alice, whose neurological assessment was not normal today, and in my view a re-examination should be carried out before she is discharged home.
Scheme of neonatal neurological examination
General observations
Head size and shape
The head circumference should be measured as part of routine examination of the newborn ( Ch. 14 ), and the importance of documenting head size and head growth as part of neurological assessment cannot be overstressed. Fontanelle size and tension must be recorded.
The newborn skull is not fused, and it is possible to palpate the cranial sutures which should not be ridged or separated too far ( Fig. 40.2 ). Observe any superficial swellings such as cephalhaematoma or subgaleal collections. Fused sutures (craniosynostosis) may need treatment, or may be a clue to an underlying disorder such as Apert’s syndrome. Advice may need to be obtained from a neurosurgeon.

Orientation and behaviour
Behavioural states
The initial assessment of the baby’s nervous system should involve evaluation of state of alertness, a sensitive indicator of neural integrity. Alertness or behavioural states can be described on the basis of four features ( Table 40.1 and Fig. 40.1 ) ( ; ; ).
STATE | EYES OPEN | RESPIRATION REGULAR | GROSS MOVEMENTS | VOCALISATION |
---|---|---|---|---|
1 | −1 | +1 | −1 | −1 |
2 | −1 | −1 | 0 | −1 |
3 | +1 | +1 | −1 | −1 |
4 | +1 | −1 | +1 | −1 |
5 | 0 | −1 | +1 | +1 |
Term babies spend about 50 minutes of each hour asleep and about 50% of the time in quiet sleep (state 1). Babies usually cycle between states, and failure to do so is abnormal; examples of conditions that cause this are drug withdrawal and hypoxic–ischaemic encephalopathy.
State 1: deep or quiet sleep
Quiet sleep begins when a baby who keeps his eyes closed begins to breathe regularly. Eye movements under the closed eyelids are not present. The baby is generally still and not moving. Rhythmical mouthing movements can appear, lasting a few seconds up to a maximum of a few minutes. Although gross motor movements are rare, startles do occur in this phase of sleep.
State 2: light or rapid eye movement sleep
In this phase of sleep respirations are irregular. The transition from state 1 into state 2 is frequently marked with a startle, gross movements or a sigh. Under the closed eyelids, slow eye movements can be observed. Small twitches are common in state 2 and are visible in the face, hands and feet. Grimaces, smiles and rhythmical mouthing are observable at times. The many movements result in frequently changing postures.
State 3: awake, drowsy, dozing
In state 3, the baby lies still and keeps his eyes open, although they may be heavy-lidded. Although there may be moments of staring, most of the time the baby seems to scan the environment with rapid eye movements. A stable posture and regular respiration are usual. Babies have a dazed look in this half-awake state. The longest such periods occur after a feed.
State 4: quiet alertness
In state 4, the baby is alert with eyes open and minimal motor activity; there can be a glazed look that is easily changed into a brighter look with appropriate visual or auditory stimulation.
State 5: alert
The baby is alert and content with bright open eyes. Posture changes frequently and sequential, fluent movements of all four limbs (also known as writhing movements) are seen.
State 6: crying
Crying is a communication signal, and it is clear that parents and nurses can often discriminate between cries of discomfort, hunger and pain.
Behavioural state of the neonate less mature than 36 weeks’ gestation
Babies born before 36 weeks’ gestation spend a great deal of time asleep, but their sleep states cannot be classified so easily. , on the basis of a longitudinal study of very low-risk preterm infants, came to the following conclusion: ‘Cycles of rest and activity, regular and irregular breathing, and epochs with and without eye movements may alternate independently and may accidentally overlap, but often do not coincide at all before 35–37 weeks’.
Abnormal states, abnormal state cycling and coma
Nearly every disorder that affects the central nervous system disturbs the level of alertness at some time. Babies are usually arousable, that is, they will awaken to the sound of a bell or a bright light. Failure to arouse means that a baby is stuporose or comatose. Both in utero ( ) and after birth stereotyped movements are an indicator of disease, and a rich variety of movement is an indicator of health.
In conclusion, in healthy newborns, both full-term and preterm, cycles of activity should be present. After 36 weeks these are recognised as distinct behavioural state cycles as described above; before 36 weeks these consist of more or less independently occurring cycles of activity and quiescence, of regular and irregular breathing, and of periods with and without rapid eye movements. Recognition of these cycles can be helped by looking at the electrocardiogram, respiratory pattern and Tc P o 2 . Attention to assessment of behavioural state and behavioural state cycling may be the single most sensitive way to assess the integrity of the neonatal central nervous system, and should be the starting point for examination of the nervous system.
Consolability/cuddliness
During an examination, or when they are cold or hungry, babies often cry but are usually consolable. stress that normal babies are ‘cuddly’ and will mould into the crook of an arm or nestle in the examiner’s neck. Persistent, high-pitched crying is abnormal, as is the stiff ‘uncuddly’ behaviour of a baby with encephalopathy.
Posture, spontaneous movement and tone
Active tone and movements
Normal muscle offers a resistance to stretch which is felt by the examiner as tone. Active flexor tone appears between 28 and 34 weeks and matures from the feet and legs upwards ( ). This is clearly seen by watching the posture adopted by term babies, who lie with their limbs flexed and adducted, unlike preterm babies who adopt an extended posture. Asymmetrical tone does not always indicate asymmetrical pathology in the newborn period. A pattern of persistent mixed change in tone with hypertonia in the limbs and hypotonia in the trunk is abnormal, as is arching of the trunk at any age. When the whole body is arched, the term ‘opisthotonos’ is used. Tone can alter considerably in relation to medication, feeds and sleep state, and sequential examinations are required to confirm physical signs.
A term newborn makes smooth, varied, spontaneous and symmetrical limb movements which stop when the baby’s attention is diverted ( ). Finger movements are elegant and varied, involving the thumb, which can be abducted away from the palm by term ( ). A persistently adducted thumb (cortical thumb) in a tightly fisted hand is abnormal ( ; ), and babies with brain injury often have this sign, with a paucity of fine finger movements.
Facial expression
Spontaneous facial movements are frequently seen in the normal newborn, although bilateral facial paralysis (as in Moebius syndrome) is much more difficult to diagnose than the more common unilateral facial palsy. Normal facial expression in an otherwise hypotonic and flaccid newborn baby suggests a spinal cord problem. Babies of just a few days old will often imitate facial gestures, for example putting out their tongues.
Limb movement, passive tone and power
Before passive movements are used to assess tone it may be possible to observe spontaneous movements of the limbs against gravity. Failure to move part or all of a limb may be due to pain or paralysis. Limb tone is influenced by the tonic neck reflex in newborns which means it is important to have the head in the midline before beginning to elicit passive movements. These involve gentle flexion of the upper and lower limbs, then rapid extension and observation of recoil. A summary is contained within the protocol suggested by . This examination system, like that of , involves assessing angles made by bending and manipulating the limbs and trunk. These include the popliteal angle ( Fig. 40.3 ), the foot dorsiflexion angle and the scarf sign. A reduced popliteal angle and clusters of abnormal signs are sensitive indicators of later outcome ( ).

Jitteriness
The normal term newborn is in a state of relative hypertonicity, with brisk reflexes tending to clonus. This high tone can lead to the clinical sign of jittering. Jittering is a high-frequency, generalised, symmetrical tremor of the limbs which is stilled by flexion or by inducing the baby to suck on a finger. Jittering is common in the first 2 or 3 days in term babies and is generally benign ( ) but if it is excessive or persistent it deserves investigation (see seizures, p. 1080 ). Repetitive chewing movements or tongue thrusting are not part of jitteriness and imply seizures. Jitteriness is stimulus-sensitive, whereas seizures are not. In seizure the movement has a fast and slow component whereas in jittering the tremor is symmetrical. Jittering is never accompanied by physiological changes due to the activation of the autonomic nervous system such as tachycardia, hypertension or apnoea.
Trunk and neck tone and power
Normal term babies have sufficient power in their neck muscles to lift their heads slightly when prone or supine. Preterm babies can manage to turn their heads from side to side but have much less power, with complete head lag when pulled to sit. In order to judge tone in the neck and trunk, babies should be pulled to sit by holding them at the shoulders ( Fig. 40.4 ). The pull-to-sit manoeuvre elicits an attempt to raise the head in a normal term newborn ( Fig. 40.4a ). If the head is unsupported it will gradually fall forwards or backwards: normal term babies will be able to raise their heads to the vertical again from either direction ( Fig. 40.4b ), but it is normal for the head of a term baby to wobble. Truncal tone can be assessed by placing the baby on his side, with one hand on the back and the other manipulating the legs. It is normally easier to flex a baby’s trunk than to extend it.


Reflexes
Tendon and Babinski reflexes
Eliciting tendon reflexes is of less value in the newborn period than later in childhood. Knee and biceps jerks can usually be obtained. Reflexes at term are very brisk due to the high tone, and a few beats of clonus at the ankle are usual. Very brisk reflexes and clonus are not reliable indicators of an upper motor neurone lesion until about 6 months of age ( ). A crossed adductor response to the knee jerk (see below) is also usual in the first months of life whereas the sign is abnormal later on. The plantar reflex of Babinski is always extensor in babies, and this test is best omitted as the stimulus is painful and often results in a withdrawal response.
Primary neonatal reflexes
Whilst these responses are intriguing to doctors and parents alike it is necessary to have a working knowledge of only a few. Primitive reflexes normally habituate after repeated performance. Persistence of primitive reflexes can inhibit normal movement in children with cerebral palsy.
Moro reflex
This reflex is usually elicited by allowing the previously supported head of a baby to fall backwards slightly, whereupon the baby extends and adducts both upper limbs, opening the hands ( Fig. 40.5 ). Babies of greater than 33 weeks’ gestation subsequently adduct their arms. The Moro response is present from 28 weeks of gestation and usually disappears by 4 months. Persistence beyond 6 months is always abnormal.

Asymmetric tonic neck reflex
Starting with the baby supine and the head in the midline the head is slowly turned to one side. This results in increased extensor tone in the arm on the side to which the head is turned and increased flexor tone in the arm on the opposite side (fencing posture). The reflex appears by 35 weeks’ gestation, is very prominent by about 1 month of age and disappears by about 7 months.
Crossed extension (adduction) reflex
One leg is held in extension and the sole of the foot is rubbed. The other leg first withdraws and then extends with fanning of the toes. The third and final component of the fully developed reflex brings the other foot towards the side which was stimulated. Eliciting the knee jerk often produces this reflex in the neonatal period, which should not persist after 8 months of age.
Placing and stepping
By stimulating the dorsum of the foot, usually by bringing it into contact with the edge of the couch, a mature baby can be induced to ‘step’ over the edge ( Fig. 40.6 ). The baby’s toes fan out and he lifts his foot up and then places it on the surface. Babies will extend their legs on to a flat surface and ‘support’ their weight when held under the arms. With the feet in contact with a solid surface and the body tilted forwards the baby will ‘walk’.

Rooting, sucking and swallowing
Stroking the upper lip of a baby of 28 weeks’ gestation and above results in the baby searching for the nipple and opening the mouth. This reflex tests the sensation in the distribution of the fifth cranial nerve and the motor pathways of cranial nerves V, VII, XII. Swallowing also involves cranial nerves IX and X. The sensory input for the sucking reflex comes from the hard palate, not the tongue or cheek. Sucking begins during the 11th week of intrauterine life. Coordination between sucking and swallowing exists from 28 weeks’ gestation but the strength to sustain it and to synchronise the process with breathing is only adequate after 32–34 weeks’ gestation. Sucking gradually builds up from bursts of three sucks at a time to eight or more, with a reduction in the interburst interval. If sucking is absent, test the gag reflex by gently stroking the soft palate with a cotton bud.
Palmar and plantar
The palmar reflex results from stroking the palmar surface of the hand, eliciting a grasp that is often strong enough to lift the baby from the crib ( Fig. 40.7 ). It is present from 26 weeks’ gestation and persists up to 4 months. Stroking the plantar surface of the foot results in curling of the toes in a similar manner to the palmar response ( Fig. 40.8 ).


Pupillary reflex
The pupils respond to light only after 30 weeks’ gestation, and the response was present in all infants after 35 weeks ( ). Babies of less than 30 weeks’ gestation do respond to a bright light, by blinking or averting their eyes. The size of the pupil gradually decreases after the development of the light reflex. The amplitude of the response, that is, the difference in size of the pupil before and after the light exposure, increases up to term. A small pupil on the side of an Erb’s palsy suggests Horner’s sydrome due to involvement of C8 and T1 nerve roots. A large pupil can indicate a congenital or acquired third-nerve palsy.
Neonatal neurological alarm signals
Certain neonatal neurological signs are generally recognised as potential indicators of serious disease. These ‘alarm signs’, derived from the work of many authors ( ; ; ; ; ; ; ; ), are:
- •
persistent irritability
- •
difficulty in feeding
- •
persistent deviation of head and/or eyes
- •
persistent asymmetry in posture and movements
- •
persistently adducted thumbs in a fisted hand
- •
opisthotonos
- •
persistent posture of flexed arms and extended legs
- •
apathy and immobility
- •
floppiness, severe generalised hypotonia
- •
convulsions
- •
abnormal cry
- •
the combination of setting-sun sign, vomiting, wide sutures and/or abnormal increase in skull circumference.
Respiratory difficulties and apnoea can be signs of neurological dysfunction, although apnoea of prematurity is the commonest cause on a modern neonatal unit ( Ch. 27 , part 4). In one large study, recurrent apnoea (i.e. three or more episodes of apnoea of longer than 20 seconds’ duration) occurred in 1% of 25 154 babies evaluated between 1974 and 1979 ( ). Of the affected babies, apnoea commenced in the first 2 days of life in 77% and was unlikely to commence after 7 days ( ). The gestational age at birth had a major impact with respect to the postnatal age when the last apnoea was detected.
Special senses
Examination of vision, visual evoked potentials
The assessment of visual function in newborn babies includes behavioural and electrophysiological techniques.
Behavioural techniques are based on the observation of spontaneous or elicited visual behaviours in the baby. The 26-week gestation preterm baby blinks in response to light; by 32 weeks there is eye closure; by 34 weeks a baby is able to fix and track a bright object briefly. By 37 weeks a baby will turn to soft light, and can track reliably ( Fig. 40.9 ). Optokinetic horizontal nystagmus is present when a term baby looks at a striped rotating drum or a striped tape is moved in front of the baby’s eyes. Changing the width of the stripes or forced-choice preferential looking at striped grids can be used to test visual acuity, which is equivalent to 20/150 vision at term. Vertical or asymmetrical nystagmus should always be investigated ( ).

The interpretation of deficient neonatal visual responses, however, is much more difficult. Dubowitz stresses the importance of the loss of previously good visual performance as a disturbing sign. Babies’ eyes are usually in alignment, although a slight horizontal divergence is normal until 6 weeks of age, particularly in preterm babies. Vertical or skew deviation is always abnormal and has been seen in association with germinal matrix haemorrhage/intraventricular haemorrhage ( ). For more information on abnormal and delayed visual development, and squint, see Chapter 33 .
Electrophysiological techniques measure the visual evoked potentials which are produced within the occipital cortex as a result of repeatedly applying an appropriate visual stimulus so that the minute electrical response to it, which will be identical each time, can be extracted from the random background electrical noise (EEG) by computerised averaging. Strobosocopic or flashing red lights are used which can penetrate closed eyelids. The electrical response ‘matures’ with advancing gestation and can be detected from 25 weeks. Study of visual evoked potentials has been found to be of value in predicting outcome after birth asphyxia ( ), and is a sensitive test for the integrity of the visual pathway. Absent visual evoked potentials predicts cortical blindness in preterm infants with extensive cystic leukomalacia ( ), and visual impairment is the most important variable in determining the neurodevelopmental scores in these infants ( ).
Auditory testing in the neonatal period
The fetus responds to sound from 19 weeks of gestation ( ). Babies from 28 weeks of gestation respond to noise by turning their heads, arousing from sleep or increasing their body movements. Bilateral sensorineural deafness occurs in about 1.5 per 1000 children. Successful universal neonatal screening programmes developed for use in the UK ( ; ) have been rolled out gradually since 2000. Universal neonatal hearing screening is now established in the UK ( www.hearing.screening.nhs.uk ). Universal neonatal hearing screening is more cost-effective than the infant distraction test and children treated early have better speech and language skills, although debate continues about the strength of the evidence ( ).
Otoacoustic emissions
Otoacoustic emissions were discovered in 1978. Otoacoustic emissions are low-amplitude sound waves which are produced by the inner ear; they occur spontaneously as well as in response to a click stimulus. The automated method depends on the fact that a click stimulus, when presented to an intact hearing ear, evokes an otoacoustic emission which can be detected by a probe lying in the ear canal. Testing with otoacoustic emissions is quicker to administer than with an automated evoked brainstem response method but there are more false-positive results.
Brainstem auditory evoked potential
These indicate electrical events generated in the brainstem auditory pathway in response to sound (usually a click) presented at the ear. The electrical signals are recorded with EEG electrodes on the scalp. The results of many click stimulations are summed by a computer which uses coherent averaging to eliminate the background noise generated by the local EEG signal. The mature pattern consists of seven waves, but these are poorly developed with increased latency and require a larger stimulus in order to elicit them in babies, in whom the response is present from 24 weeks. Automated brainstem response equipment eliminates the need for extensive operator training and is a widely used method of hearing screening.
Imaging
For advice on the best imaging modality to choose when investigating the central nervous system, see Chapter 43 .
Examination of cerebrospinal fluid and intracranial pressure
There should be a low threshold for performing lumbar puncture in babies because the signs of meningitis are subtle. For practical advice on how to perform the procedure, see Chapter 44 . In the neonatal period cerebrospinal fluid may be xanthochromic because of jaundice or old germinal matrix haemorrhage/intraventricular haemorrhage. The mean and median white cell count is less than 10 cells per mm 3 and the upper limit of normal is around 21 ( Appendix 1 ). Red cell counts of around 100 per mm 3 in the preterm baby are considered normal and counts more than 1000 per mm 3 make the interpretation of cerebrospinal fluid results very difficult: applying correction factors using the ratio of white cells to red cells has been shown to be inaccurate. The only course of action is to repeat the lumbar puncture after 24 hours.
Measured accurately with pressure transducers at lumbar puncture, the intracranial pressure was 0–5.5 mmHg ( ) and 2 mmHg ( ).
Electroencephalography
Conventional multichannel EEG recordings are difficult to obtain in newborn babies, but the results can provide very valuable information ( ; ). Short recordings are of less value than prolonged ones as the EEG shows wide variability and changes not only with sleep state but also with the length of the preceding sleep epoch and the degree of maturity of the baby ( ; ; ). Continuous monitoring is possible with a cerebral function monitor, which displays the amplitude of one or more channels of processed EEG, and modern equipment offers the opportunity to obtain multiple channels of raw EEG and a digital video signal in addition to a compressed and filtered amplitude-integrated EEG ( Fig. 40.10 ). Cerebral function monitoring has drawbacks, but the advantages include continuous monitoring and relative ease of interpretation ( ).

Maturation of EEG
The EEG of very preterm babies is markedly discontinuous with a pattern of high-voltage slow activity with suppressed EEG activity, termed ‘tracé discontinu’ ( ). Preterm babies also exhibit a pattern, called ‘delta brush’, of fast waves superimposed on delta waves, which can be misinterpreted as convulsive activity. Delta brush is most abundant at 32 weeks’ gestation, and is very rarely seen after term. With increasing gestation the interburst intervals decrease and the record becomes more continuous ( Fig. 40.11 ). Abnormal background EEG activity, such as severe amplitude depression or burst suppression, correlates well with later adverse outcome in both preterm and asphyxiated term babies ( ; ; ; ; ). See part 2 and part 4 of this chapter for the role of the EEG in neonatal encephalopathy and page 1084 for its use in seizure detection.




Neurological examination before discharge, and follow-up
A complete neurological examination including assessment of movements is the best way of assessing the neurological status of a high-risk infant as long as is performed in a sequential way because it is the pattern of change that gives away the diagnosis and improves the accuracy of prediction. Therefore, good practice demands that high-risk babies should be closely monitored after discharge and during infancy. In high-risk preterm infants found a high correlation between the neonatal and follow-up examinations during infancy. Cramped synchronised movements on video assessments at term followed by absent or abnormal fidgety movements at 3 months of age are known to be extremely sensitive and specific for early diagnosis of cerebral palsy ( ). The neonatal hemisyndrome and hypertonia syndrome have distinct prognostic value, whereas hypotonia does not. With asphyxia, however, hypotonia which evolves to hypertonia correlates significantly with subsequent neurological disorders ( ). The neonatal hyperexcitability syndrome appears to correlate with subsequent developmental difficulties only when it persists for more than 6 weeks. If, upon discharge from the neonatal unit, it is concluded that the baby is definitely neurologically abnormal, on the basis of established abnormalities such as microcephaly or seizures, a nearly 100-fold increased risk of cerebral palsy does exist ( ).
Follow-up of high-risk infants is an important part of neonatal intensive care provision ( Ch. 19 ). Monitoring of head growth and neurodevelopment over the first months can give early warning of neurodevelopmental problems. A failure to achieve a normal pattern of head growth is an ominous sign.
Neonatal seizures
- Geraldine Boylan
- Janet M Rennie
Introduction
Seizures are the most common neurological emergency in the neonatal period and present a diagnostic and therapeutic challenge to clinicians worldwide. The neonatal brain is uniquely vulnerable to seizures and, as a result, seizures are more common in the neonatal period than at any other time of life. There is now convincing evidence that seizure can damage the brain and exacerbate pre-existing injury. Therefore it is imperative that seizures are identified and treated as soon as possible. However neonatal seizures differ in etiology, semiology, and electroencephalographic (EEG) characteristics to seizures in older children and adults and antiepileptic drugs (AEDs) do not work very well. There is also concern about the potential adverse effects of currently used AEDs on brain development.
All those who care for the newborn need a working knowledge of the likely causes and a management plan for this important emergency. Prompt diagnosis, investigation and treatment are vital as delayed recognition of a treatable cause can have a significant impact on the child’s subsequent neurological outcome. In neonates seizures are associated with conditions such as periventricular haemorrhage, cerebral infarction (stroke), hypoglycaemia, infection, cerebral malformations and hypoxic–ischaemic encephalopathy.
There is increasing evidence that neonatal seizures have an adverse effect on neurodevelopmental outcome and predispose to cognitive, behavioural or epileptic complications in later life ( ). Seizures cause synaptic reorganisation with aberrant growth (mossy fibres), and may interfere with the normal synaptic pruning which takes place during development ( ). If seizures are not controlled the electrical activity can continue to circulate, a phenomenon known as kindling. Prolonged seizures cause progressive cerebral hypoxia, changes in cerebral blood flow, cerebral oedema and lactic acidosis; changes have been shown in human babies with Doppler ultrasound and using magnetic resonance spectroscopy ( ; ). Status epilepticus worsened the outcome for neonatal rats with hypoxic ischaemia ( ). Some clinical studies have shown a correlation between the number of seizures and outcome, but others have not, although very few neonatal studies have been done using EEG quantification of the seizure burden ( ).
Pathophysiology
There have been substantial recent advances in our understanding of the pathophysiology of neonatal seizures and more specifically in the developmental age-specific factors that influence mechanisms of seizure generation, response to AEDs and neurodevelopmental outcome. The neonatal period is also a period of intense physiological synaptic excitability as the balance between excitatory versus inhibitory synapses is tipped in the favour of excitation to permit robust activity-dependent synaptic formation, plasticity and remodelling ( ) ( Fig. 40.12 ). Glutamate receptors are essential for plasticity and are transiently overexpressed in the neonatal period. In addition both N -methyl- d -aspartate (NMDA) and alpha-amino 3-hydroxy-5-methyl-4-isoxazole propionate (AMPA) receptors are developmentally regulated and are expressed at levels and with specific subunit composition that enhances excitability in neuronal networks in the neonatal brain ( ). AMPA receptors are not calcium-permeable in the adult; in the newborn the receptors are deficient in the Glu-R2 subunit which renders them calcium-permeable and enhances excitation. Gamma-aminobutyric acid (GABA) is excitatory rather than inhibitory in perinatal neurons because of elevated neuronal chloride. This in turn is due to the presence of the chloride channel NKCCl which mediates chloride influx. The expression of the NKCCl cotransporter is high in the perinatal period and the high levels of neuronal chloride lead to an efflux of chloride when GABA-A receptors are activated ( ). Hypoxic ischaemia leads to a further upregulation of the NKCCl transporter and hence the response to GABA agonist drugs such as phenobarbital and the benzodiazepines is particularly poor in hypoxic–ischaemic encephalopathy. Ion channels also regulate neuronal excitability and mutations and low expression of certain channels such as K + and HCN lead to enhanced excitability. Neuropeptide systems are also implicated in enhanced neuronal excitation; for example, corticotrophin-releasing hormone and its receptors are expressed at higher levels in the postnatal period and are increased during stress.

Basic science research has shown that, in animals, status epilepticus causes neuronal loss in the hippocampus. Cell death occurs from excessive excitatory neurotransmitter release, which allows calcium to enter the cell and trigger a cascade of biochemical changes, including activation of nitric oxide synthase, and enzyme activation. Seizures also activate genes that can lead to abnormal axon growth and synaptic reorganisation. This has been seen as ‘mossy fibre sprouting’ in the hippocampus, and sprouting and neosynapse formation have been seen in other areas of the brain ( ). Holmes’ group has also shown alterations of neural pathways in animals subjected to repeated seizures, with early gene activation of c-fos ( ), and suggested that seizures in early life may modify a wide range of essential processes such as neuronal migration, aborisation and synaptogenesis, leading to permanent effects on seizure susceptibility, learning and memory ( ). This fundamental work has helped to explain why anticonvulsant drugs which are effective in older individuals do not work well in babies ( Fig. 40.13 ).

Incidence
There is now clear evidence that there is both under- and overreporting of neonatal seizures in the neonatal intensive care unit (NICU) when EEG monitoring is not available for confirmation ( ; ; ). All of the epidemiological studies to date report the incidence of clinical seizures: an epidemiological study is impossible without current EEG monitoring technology. Clinical neonatal seizures occur in 5–13% of very-low-birthweight infants, and in 1–3 per 1000 of infants born at term ( ; ; ; ; ; ; ; ; ; ). In a recent study of over 70 preterm babies monitored with amplitude-integrated EEG (aEEG) who had a formal EEG within 2–48 hours of birth, five were found to have definite seizures ( ).
Many of the cases of clinical seizure reported in older studies were due to late-onset hypocalcaemia, which probably explains the higher incidence. The incidence of early (<48 hours) seizures in term infants has been proposed as an indicator of the quality of perinatal care because the most common cause in this group is hypoxic–ischaemic encephalopathy. The incidence of early seizures varies, being 0.87 per 1000 in Dublin between 1980 and 1984 ( ), 1.3 per 1000 in Cardiff during 1970–1979 ( ), 2.8 per 1000 in Fayette county, Kentucky in 1985–1989 ( ) and, in the most recent study, 1 per 1000 in California (Glass et al. 2008). There are many problems in the definition of neonatal seizures which will become apparent to the reader. When clinical signs of seizures are present they are commonly subtle in nature, particularly in premature infants, being present in 75% of the cases described by .
Time of onset
Most neonatal seizures start between 12 and 48 hours after birth; babies rarely present with seizures in the delivery room. In our experience, full-term babies who are thought to be seizing on admission to the neonatal unit after a difficult delivery often have abnormal jittery movements without electrographic seizures. This is particularly common after moderate or severe hypoxic–ischaemic injury.
With the advent of moderate hypothermia treatment for hypoxic–ischaemic encephalopathy, EEG monitoring generally commences earlier and it is now often possible to capture the true onset of electrographic seizure in neonates. In a study by , electrographic seizures began at a mean time of 9 hours and 30 minutes in full-term neonates treated with hypothermia for hypoxic–ischaemic encephalopathy. In a study by the onset of electrographic seizures in the majority of neonates with hypoxic–ischaemic encephalopathy, also treated with hypothermia, was within 18 hours of birth. compared the seizure characteristics of two full-term cohorts of neonates with hypoxic–ischaemic encephalopathy: one group had received therapeutic hypothermia and the other group did not. The onset of electrographic seizures in both groups was not significantly different, with a mean of 18 hours in the non-cooled group and 13 hours in the cooled group. A study by in non-cooled term neonates with hypoxic–ischaemic encephalopathy showed electrographic seizure onset before 20 hours.
Animal work shows that seizures emerge 7–13 hours after a hypoxic–ischaemic insult ( ), and this fits with what is known about glutamate release and damage during the secondary reperfusion phase in this situation. Late-onset seizures suggest meningitis, benign familial seizures or hypocalcaemia . Figure 40.14 shows the time of onset recorded in 277 neonatal cases ( ). A study carried out 17 years later shows a very similar pattern ( ).

Diagnosis and classification
In 1870 J Hughlings Jackson described a seizure as ‘an excessive discharge of nerve tissue on muscle’. Today this definition is expanded to include the effects on the sensory and autonomic nervous systems, including paroxysmal alterations in behavioural state. Four main seizure types are recognised ( Table 40.2 ) and within each type the seizures can be unifocal, multifocal or generalised. Classic tonic-clonic seizures are very rare in babies, in whom there is the additional problem of electroclinical dissociation ( ).
TYPE | CLINICAL MANIFESTATION | FREQUENCY AND EEG CORRELATE |
---|---|---|
Subtle | Eye signs: eyelid fluttering, eye deviation, fixed open stare, blinking. Apnoea. Cycling, boxing, stepping, swimming movements of limbs. Mouthing, chewing, lip smacking, smiling | About 50% of neonatal seizures; EEG correlation variable. EEG changes most likely with ocular manifestations |
Tonic | Stiffening. decerebrate posturing | About 5% of neonatal seizures. EEG correlation variable |
Clonic | Repetitive jerking, distinct from jittering. Can be unifocal or multifocal | 25–30% of neonatal seizures. EEG correlation highly likely, especially if focal |
Myoclonic | Myoclonic jerks; sleep myoclonus can be benign | 15–20% of neonatal seizures. EEG normal if focal, or sleep myoclonus |
Subtle
The clinical manifestations of seizures in infants can be extremely subtle. They can be divided into orofacial manifestations, including fixed eye opening, eye deviation, eyelid blinking, sucking, chewing and lip smacking, and limb movements (often described as swimming, boxing or cycling). In addition, apnoeic episodes can be due to seizure and this diagnosis should be considered if there is slow response to bag-and-mask ventilation, particularly in a preterm neonate with an intracranial lesion. Another clue may be associated movements such as eyelid or mouth opening and a bradycardia which starts soon after the collapse. Autonomic changes, such as a change in blood pressure or increased salivation, often accompany subtle seizures and these may provide a clue to the correct diagnosis in the absence of EEG confirmation.
Clonic
Clonic seizures usually involve one limb or one side of the face or body jerking rhythmically, usually at a frequency of 1–4 times per second. This type of seizure is associated with a characteristic EEG discharge consisting of runs of sharp slow-wave complexes which spread ipsilaterally from the hemisphere in which they originate. Clonic seizures in the neonate can have more than one focus or migrate in a non-jacksonian fashion; for example, jerking of one leg can be followed by similar movements in the opposite hand. Clonic seizures are often a clue to an underlying focal lesion such as a cortical infarction and are frequently associated with neonatal stroke ( ). They may also occur in association with a metabolic cause and occasionally herpes encephalitis. Infants are not usually unconscious during clonic seizures.
Tonic
Tonic seizures are much less common than either subtle or clonic seizures in the newborn. Sustained posturing of the limbs or trunk or deviation of the head or eyes are the usual manifestations of tonic seizures in the newborn.
Myoclonic
Myoclonus is characterised by sudden brief and jerky movements which tend to occur in the flexor muscle groups. It can be localised to one muscle group or it may be generalised. It is irregular and arrhythmic and is of high amplitude. Generalised myoclonic seizures resemble salaam spasms and are the type most likely to be associated with EEG change. Myoclonic seizures are most frequently noted in preterm infants with major cerebral pathology and the background EEG pattern is usually abnormal. Myoclonic jerks can also occur in babies who are receiving midazolam for sedation, particularly during weaning ( ). Some babies with serious underlying disorders such as glycine encephalopathy suffer from very troublesome repetitive myoclonic jerking as their only seizure manifestation ( ).
Jitteriness
Jitteriness is an extremely common phenomenon in normal newborns, being observed in 44% of a sample of 936 babies ( ). It is defined as involuntary rhythmic oscillatory movement around a fixed axis that can be either fine (fast (>6 Hz) and of low amplitude) or coarse (slow and of high amplitude) ( ). Fine jittery movements are usually benign or associated with a metabolic disturbance such as hypoglycaemia. Coarse jittery movements are more commonly associated with hypoxic–ischaemic encephalopathy or intracranial haemorrhage. Excessive jitteriness has also been reported in infants born to mothers who use marijuana, and can be a sign of neonatal abstinence syndrome ( Ch. 26 ). Jittering is a symmetrical tremor, without the fast and slow component of a clonic or myoclonic seizure, and occurs at a faster rate of 5–6 times per second. Jittering does not involve the face (unlike subtle seizures), is markedly stimulus-sensitive, and ceases when the limb is held. The autonomic nervous system changes of a seizure, such as tachycardia or hypertension, are never seen in jittering. The EEG does not display electrographic seizure activity and fast-frequency movement artefact is common, synchronous with jittering movements.
Hyperekplexia
Hyperekplexia is a rare disorder caused by autosomal-dominant or recessive modes of inheritance and characterised by generalised muscle rigidity, nocturnal myoclonus and a very exaggerated startle response to auditory, visual and tactile stimuli ( ; ). The startles can look like myoclonic jerks, and the high tone, hyperreflexia and jitteriness can lead to an erroneous diagnosis of seizure ( ). Hyperekplexia is probably the same condition previously known as hereditary stiff-baby syndrome ( ). Although the EEG is normal, the tonic spasms can be dangerous. Severe cases can lead to neonatal cardiac arrest and treatment is warranted. Treatment with clonazepam (0.05–0.2 mg/kg/day) or low-dose clobazam (0.25–0.3 mg/kg/day) usually results in marked improvement, and the episodes usually disappear by the age of 2 years ( ). The disorder is caused by mutations in the alpha subunit of the inhibitory glycine receptor ( ) and has been mapped to chromosome 5 (de Koning-Tijssen and Rees 2007); it can be inherited in an autosomal-dominant fashion, although some forms are autosomal-recessive ( ).
Physiological changes during seizures
Neonatal seizures cause an increase in metabolic rate, a decline in energy reserves, including brain glucose, and an increase in lactate ( ). This implies that brain transport mechanisms are unable to match the increased demand. The demand for oxygen is also increased, and cerebral blood flow rises to try to meet the need for oxygen and glucose. That metabolic demand outstrips supply in the newborn is supported by magnetic resonance spectroscopic data showing a shift in spectra from the high-energy phosphate compounds towards inorganic phosphate ( ). The changes in the brain are similar to those seen in hypoxic–ischaemic injury. Glucose pretreatment is effective in reducing the high mortality of status epilepticus in rats, a benefit which is not seen with ketone body supplementation, although the neonatal brain is known be able to utilise alternative fuels. Lactate accumulates during seizure, and the brain (and often the arterial) pH falls. Systemic blood pressure increases, and cerebral blood flow rises ( ). These dramatic short-term effects are followed by the changes in cell structure and synaptic linkages referred to earlier.
EEG diagnosis of seizures
Normal neonatal EEG
The EEG of the normal full-term baby is continuous, contains moderate-voltage mixed-frequency activity, shows fully developed sleep cycles and is reactive to stimuli even within the first 12 hours of birth ( ). The type of continuous activity present depends on whether the baby is awake or in active or quiet sleep (this chapter, part 1). During quiet sleep the EEG may show a pattern called high-voltage slow-wave sleep that consists of continuous medium- to high-voltage delta waves. As quiet sleep continues this pattern may become somewhat discontinuous with periods of low-amplitude beta and theta activity, alternating with 3–5-second bursts of higher-voltage 1–3 Hz activity occurring at 3–10-second intervals. This pattern is called ‘tracé alternant’. In active sleep, the EEG is continuous, of lower voltage and contains mixed-frequency activity. In wakefulness the EEG also shows a lower-voltage mixed-frequency pattern referred to as ‘activité moyenne’. The EEG of a full-term baby is illustrated in Figure 40.15a . Specific normal maturational features that may be present in the full-term EEG are anterior slow dysrhythmia (first apparent from approximately 35 weeks) and frontal sharp transients.


The most striking feature of the very preterm EEG is long periods of quiescence interrupted only by bursts of high-voltage mixed-frequency waves ( Fig. 40.15b ). The length of quiescent periods (also referred to as interburst intervals) is directly proportional to the degree of prematurity and this normal background pattern is referred to as ‘tracé discontinu’ or discontinuous pattern. This pattern is different to the tracé alternant pattern seen in full-term babies because the periods of quiescence can be completely flat. True quiescence is always less than 15 µV. In tracé alternant the discontinuous pattern is characterised by periods of high-amplitude, mixed-frequency activity alternating with periods of lower-amplitude activity at 25–50 µV (it is never completely flat). The degree of discontinuity seen in the preterm EEG would be very abnormal at term ( ). The maximum interburst interval falls from 60 seconds at 24–26 weeks, to 40 seconds at 27–29 weeks, and 20 seconds at 30–32 weeks ( ; ; ; ). After 33–34 weeks and up to 37 weeks the EEG is discontinuous only in quiet sleep and the interburst interval should be no longer than 10 seconds ( ). After 37 weeks, the EEG is continuous in wakefulness and active sleep, and tracé alternant replaces tracé discontinu in quiet sleep. Specific normal maturational features of the preterm EEG include premature temporal theta activity, which is sharp activity with a characteristic ‘saw-toothed’ appearance seen from approximately 27 weeks, and delta brush activity, which is a combination of very slow delta activity with superimposed fast components, present from approximately 30 weeks.
The abnormal neonatal EEG
The neonatal EEG may be abnormal in a number of ways:
- •
disturbances of continuity, amplitude or frequency
- •
interhemispheric asymmetry or asynchrony
- •
abnormal waveforms may be present
- •
disturbances of sleep state
- •
seizure activity may be present.
Repetitive periods of true quiescence in the term newborn are abnormal. However anticonvulsants and sedation may induce some periods of quiescence in the term newborn and prolong periods in the preterm baby ( ). characterised the neonatal EEG mainly on the basis of the interburst interval, categorising the EEG of infants <30 weeks as abnormal if the interval was >60 seconds, using a figure of 30 seconds at term. confirmed that an interburst interval of more than 30 seconds was abnormal at term, and presaged a poor outcome.
Electrographic seizures
Electrographic seizures are typically defined as repetitive, rhythmic, stereotypic activity lasting at least 10 seconds that evolve in amplitude frequency and morphology ( ). Both term and preterm infants have the ability to generate a multitude of ictal events but generally seizures originate in the central and temporal regions ( ; ).
Few studies have quantified electrographic seizure duration in the newborn ( ; ; ). used an arbitrary cut-off of 10 seconds as a minimum duration and this definition was also adopted by . Others have used 5 seconds ( ). Very short bursts of abnormal rhythmic electrical activity have also been termed brief intermittent rhythmic discharges (BIRDS). Electrographic seizures characteristically consist of monophasic repetitive discharges or spike-and-wave activity ( Fig. 40.15c and d ) ( ), but there is a rich variety in the onset, morphology and propogation patterns of neonatal seizures, which do not always correlate with the underlying pathology. An electrographic seizure should have a clear onset and conclusion, but these can be difficult to identify. Electrographic seizures may or may not be accompanied by sterotyped movements (see next section). found that the mean duration of electrographic seizures in the neonate was 2–3 minutes, with 97% of all seizures lasting 9 minutes or less and only 0.4% lasting 30 minutes or longer.
Electroclinical dissociation
There is asynchrony between the clinical and electrical representation of neonatal seizures: in only one-third of cases studied with video surveillance were the clinical and electrical manifestations simultaneous ( ; ). have shown that, on careful review of video EEG in neonates with electrographic seizures due to hypoxic–ischaemic encephalopathy, only 34% of seizures had concomitant clinical manifestations. When the medical charts of these neonates were reviewed, only 9% of seizures were actually documented during NICU stay, representing a significant gap between the electrographic, clinical and observed seizure burden.
Subtle stereotyped behaviour may or may not be associated with characteristic EEG changes, and continuous electrical monitoring detects many clinically silent seizures. One explanation is that the motor manifestations arise because of discharges from the brainstem and spinal cord which are ‘released’ because of lack of inhibition from higher centres ( ). An alternative explanation is that scalp electrodes are incapable of recording from every part of the brain; depth electrodes reveal an otherwise unsuspected electrical focus in 10% of adult patients. The neurological effects of clinically silent (electrographic) seizures are not known, nor is it certain that treatment of clinically manifest seizures to electrical quiescence is required. This is an important question because phenobarbital treatment frequently abolishes the clinical manifestations whilst the electrical paroxysms continue ( ; ).
Current clinical practice is to commence anticonvulsant treatment without obtaining an EEG in the newborn. A study by has shown that there is both over- and underdiagnosis of neonatal seizures if EEG monitoring is not used, and we would advocate using EEG if at all possible to confirm the presence of true neonatal seizures. aEEG is now widely used in NICUs, especially since the introduction of therapeutic hypothermia for hypoxic–ischaemic encephalopathy. aEEG does have limitations, however, and short seizures, especially those less than 1 minute in duration, are missed, as are low-voltage seizures and seizures which remain localised ( ; ). aEEG will usually detect seizures which are of high amplitude, generalised over a wide area and which are of long duration.
The lack of staff training and experience in aEEG interpretation is emerging as an important barrier to the effective adoption of aEEG monitoring in the NICU ( ). Nevertheless, the technique remains popular because it is easy to use and is useful for monitoring the response to antiepileptic treatment.
Many groups are now examining automated seizure detection algorithms for use in the NICU and promising results are emerging ( ; ).
Evidence is scarce on the clinical benefits of treating electrographic seizures in neonates and, with the current state of knowledge, it is acceptable practice to aim to control clinically apparent seizures. In any case, it is virtually impossible to achieve electrical control in all cases with the anticonvulsants currently recommended for newborn use ( ).
Evidence is emerging from a small number of studies designed to measure the effect of treating electrographic seizures which does suggest a reduction in the severity of magnetic resonance imaging (MRI) injury when efforts are made to treat all such seizures ( ). Evidence from large multicentre trials is essential before this practice can be recommended ( ), and a number of such studies are currently under consideration.
Aetiology
Causes of neonatal seizures in current order of importance are shown in Table 40.3 .
1 | 2 | 3 | 4 | 5* | 6 † | 7 | 8 | |
---|---|---|---|---|---|---|---|---|
Number of cases | 71 | 131 | 100 | 40 | 90 | 81 | ||
Hypoxic–ischaemic encephalopathy | 53% | 16% | 49% | 30% | 49% | 37% | 40% | 37% |
Cerebral infarction (stroke) | 12% | 17% | 1% | 11% | ||||
Intracranial haemorrhage (includes intraventricular haemorrhage and subdural haematomas) | 17% | 14% | 7% | 12% | 15% | 9% | ||
Meningitis | 8% | 3% | 2% | 7% | 5% | 5% | 20% ~ | 9% |
Maternal drug withdrawal | 4% | |||||||
Hypoglycaemia | 3% | 2% | 0.1% | 5% | 3% | 3% | ||
Hypocalcaemia, hypomagnasaemia | 22% | 5% | ||||||
Rapidly changing serum sodium | ||||||||
Congenitally abnormal brain | 8% | 4% | 3% | 17% | 10% | 6% | ||
Fifth-day fits (benign non-familial) | 52% | |||||||
Benign familial neonatal seizures | 6% | 1% | ||||||
Pyridoxine-dependent seizures | ||||||||
Hypertension | 1.4% | |||||||
Kernicterus | 1% | |||||||
Inborn errors of metabolism | 3% |
Hypoxic–ischaemic encephalopathy
This remains the most common cause of neonatal seizures at term, contributing over half the cases to most series. The characteristic time of onset is within 24 hours of birth, and seizures often begin in the first 12–18 hours. Seizures are rare in the first 6 hours unless there has been an antenatal insult ( ). For more information on the management and prognosis of this condition, see pp. 1114–1150 .
In moderate and severe hypoxic–ischaemic encephalopathy, the background EEG pattern can evolve over the first few days after birth. Very suppressed EEG activity is seen for a number of hours after birth and is often followed by a period of seizure activity that, depending on the severity of the insult, can be very difficult to control with anticonvulsants. The seizures also evolve in a characteristic manner in hypoxic–ischaemic encephalopathy over a number of days ( ). When seizures resolve, a burst suppression pattern can be seen for a number of days. Our group have reported delayed lactate clearance in neonates with hypoxic–ischaemic encephalopathy and a high EEG seizure burden ( .
Intracranial haemorrhage
Intraventricular haemorrhage is the most important cause of seizures in preterm babies. A large parenchymal haemorrhage which causes seizures is associated with a poor outcome ( ) (this chapter, part 5). Neonates of any gestation can have seizures because of bleeding into the subarachnoid space, the dural space, the cerebellum or cortex ( ). A diagnosis of intracranial bleeding in a term baby should lead to a search for a coagulation disorder, including vitamin K deficiency, and testing for haemophilia in boys. MRI has shown that a small amount of subarachnoid or subdural bleeding is common in the newborn period, and these collections usually resolve without sequelae.
Focal cerebral infarction (stroke)
Seizures in term infants with normal Apgar scores who remain alert between spasms are likely to be due to focal lesions, most commonly middle cerebral artery infarction. Ten to 15% of seizures in full-term neonates are due to stroke (p. 1108) . On EEG, the area of infarction is the focus of electrographic seizures that generally manifest as clonic seizures on the contralateral side. Seizures often require MRI for identification, and the prognosis is better than when the underlying cause is hypoxic–ischaemic encephalopathy.
The background EEG may be normal or show only mild focal abnormalities, though quiet sleep may enhance background abnormalities ( ). Diagnosis of arterial or venous occlusion in the newborn period should trigger investigations to rule out an underlying thrombotic tendency. EEG is useful for diagnosis and prognosis and should ideally be performed within 24 hours of signs; some neonates will have seizures early in the postnatal period ( ).
Seizures are also the most common presenting sign in cerebral venous sinus thrombosis (p. 1109) . Associated lesions include thalamic haemorrhage, intraventricular haemorrhage and parenchymal haemorrhagic infarction ( ). Ultrasound imaging is not reliable for the detection of stroke or sinus venous thrombosis, which often requires MRI for identification.
Meningitis
Intracranial infections, whether bacterial, non-bacterial or congenital, cause neonatal seizures usually after the first week of life. In a recent French study group B streptococci remained the most common cause of neonatal bacterial meningitis, causing 77% of early-onset and 50% of late-onset cases ( ). Escherichia coli was the most common cause of meningitis in preterm infants.
Aciclovir treatment should be started if the lumbar puncture reveals a high white-cell count in the cerebrospinal fluid (CSF), yet no organisms are seen on Gram stain in a baby who has not been treated with antibiotics, whilst awaiting the results of polymerase chain reaction for herpesvirus. The risks of starting treatment in this situation are minimal, whereas delay will worsen the prognosis. More information on the diagnosis and treatment of neonatal central nervous system infections can be found in Chapter 39 , part 2.
Neonatal abstinence syndrome and other drug-related causes of neonatal seizure ( Ch. 26 )
Withdrawal seizures can occur for the first time at any age up to 3 weeks, with a median time of onset of 10 days. EEG abnormalities are present in 50% of cocaine-exposed neonates, persist up to 1 year, and are associated with an adverse neurodevelopmental outcome. Maternal methadone addiction is more likely to be associated with neonatal withdrawal seizures than heroin ( ). Tremors have been noted in children who received prolonged infusions of narcotics for analgesia ( ), and there has been concern about the effects of midazolam for sedation in preterm babies ( ). A high incidence of benign neonatal sleep myoclonus (BNSM) has been reported in the babies of opioid-dependent mothers ( ).
Metabolic causes
Hypoglycaemia
Hypoglycaemia can be the sole cause of neonatal seizures and other neurological signs such as apnoea, lethargy and jitteriness. Often hypoglycaemia complicates hypoxic–ischaemic encephalopathy or infection, and hypoglycaemia is common in infants who are small for gestational age ( Ch. 10 ). The adverse outcome associated with the underlying cause makes it difficult to determine the prognosis of uncomplicated hypoglycaemia. However, animal evidence does show that if hypoglycaemia is present during seizures, the risk of brain injury is even higher ( ).There is no doubt that the finding of a low glucose level in a baby who is seizing is an indication for urgent intravenous treatment, and every effort should be made to normalise the glucose level as soon as possible.
Hypocalcaemia
Up until the late 1970s the high phosphate content in cow’s milk-based formula for babies resulted in a high incidence of neonatal seizures due to hypocalaemia ( ). Half the babies in the series of were hypocalcaemic (total serum calcium less than 1.75 mmol/L). Similarly high incidences of late hypocalcaemic seizures were reported prior to the introduction of modern low-phosphate milks; in the late 1960s infants were consuming doorstep cow’s milk or a high-phosphate formula. A higher than usual level of hypocalcaemia has been found as the cause for neonatal seizures in an Indian population in more recent years ( ).
A diagnosis of hypocalcaemia should lead to consideration of CATCH-22 (22q11.2 deletion syndrome), which can be diagnosed with fluorescent in situ hybridization genetic studies. Hypocalcaemic seizures occasionally occur secondary to maternal hypercalcaemia (prolonged intrauterine exposure to high levels) or maternal vitamin D deficiency and the neonatal diagnosis should prompt estimation of the maternal serum calcium. Low magnesium levels frequently accompany hypocalcaemia and require correction before the seizures will respond. Hypocalcaemia is common in ill very-low-birthweight babies and in babies with hypoxic–ischaemic encephalopathy and is probably not causally related to the seizures in these cases. Less often there is congenital hypoparathyroidism or calcium-sensing receptor defects.
Hypernatraemia and hyponatraemia
The most common cause of hypernatraemia is breast milk insufficiency and seizures can occur when the serum sodium level is very high or falls very rapidly. It is recommended that the level be reduced at a rate not exceeding 0.5 mmol/l/h when rehydrating hypernatraemic babies. Babies have also developed hyponatraemic seizures after being given excess solute-free water in intravenous solutions, or orally in the form of cheap alternatives to correctly balanced oral rehydration solutions. A very high, very low or rapidly changing serum sodium occurring in conditions such as the syndrome of inappropriate antidiuretic hormone secretion, Bartter’s syndrome or severe dehydration can cause seizures.
Congenital malformations of the brain
Disorders of neuronal migration such as lissencephaly or schizencephaly can present with neonatal seizures. Diagnosis has been facilitated with the advent of MRI but is sometimes possible with ultrasound ( pp. 1207–1212 ).
Inborn errors of metabolism
Pyridoxine-dependent seizures
The first case of an infant with intractable seizures controlled by pyridoxine was reported by . It is a rare disorder and seizures can begin during intrauterine life (mothers describe ‘hammering’ movements lasting 15–20 minutes several times a day). Seizures are very resistant to conventional anticonvulsant treatments yet cease within minutes of parenteral pyridoxine (50–100 mg) and return within days of withdrawal. This therapeutic trial can cause hypotonia requiring ventilatory support and should be carried out in an intensive care unit ( ). There is no other way to make the diagnosis, although characteristic EEG abnormalities have been recognised ( ; ). A more recent study has shown that some of the EEG changes seen after pyridoxine administration to neonates with pyridoxine-dependent seizures are also present in other treatment-resistant seizure disorders ( ). Atypical cases that respond more slowly and that show late-onset seizures requiring unusually high doses of pyridoxine (up to 500 mg) have been described ( ; ), and yet others respond to very small doses. Gospe suggests that a trial of pyridoxine should involve 100 mg intravenously every 10 minutes until the seizures stop or a total of 500 mg is reached.
The condition is caused by an inherited metabolic disorder of lysine degradation, resulting in increased urinary alpha-aminoadipic semialdehyde (α-AASA) excretion ( ). In addition to increased urinary α-AASA concentrations, mutations in the ALDH7A1 (antiquitin) gene can also identify pyridoxine-resistant seizures ( ; ). The increased a-AASA concentrations in body fluids result in pyridoxal-5-phosphate deficiency. Pyridoxal-5-phosphate is responsible for converting glutamate into the inhibitory GABA. The condition is autosomal-recessive. Supplementation of the diet with pyridoxine (vitamin B 6 ) 20–100 mg bd is required for life, and the dose may need to be increased with age ( ). Unfortunately, many of these children have severe cognitive disabilities despite early diagnosis and treatment. A better outcome has been achieved with higher doses of pyridoxine, leading to the suggestion that sufficient pyridoxine should be given to restore the CSF glutamate levels to normal.
Diagnostic tests such as measurements of urinary AASA and pipecolic acid, as well as gene testing for mutations in the antiquitin gene encoding for the AASA dehydrogenase enzyme, are now proving very useful for this disorder ( ; ).
Glycine encephalopathy
Non-ketotic hyperglycinaemia is an inborn error of glycine metabolism in which large quantities of glycine accumulate in all body tissues, including the brain. Neonates exhibit lethargy, hypotonia, apnoea, characteristic hiccups and intractable epileptic seizures that are not specific to this disease. Rapid progression can lead to intractable seizures, coma and respiratory failure. The outcome is invariably poor, and many die before the age of 1 year. Levels of glycine in blood, urine and CSF are very high. The EEG shows a generalised burst suppression pattern characterised by periodic high-amplitude bursts of activity on a near-silent background ( ; ). Dextromethorphan monotherapy (35 mg/kg/day) was associated with cessation of seizures and normalisation of the EEG in a single case, but this regimen is not always successful ( ). A recent report has shown an increase in seizure frequency following valproate treatment ( ).
Glucose transport across the blood–brain barrier is mediated by the facilitative glucose transporter isoform 1 (GLUT-1). A deficiency of this transporter results in impaired energy supply to the brain, and was recognised by . This rare disorder is important because treatment has the potential to lead to a normal neurological outcome, and because the inheritance is autosomal-dominant ( ). The interictal EEG is normal in this disorder, with spike-and-wave discharges generally only emerging during seizures ( ). Babies have a low CSF glucose concentration, with low to normal CSF lactate levels despite normal blood glucose concentrations. Elevated lactate levels would suggest a mitochondrial disorder. The diagnosis should be suspected if the CSF glucose is less than a third of the blood glucose level, and GLUT-1 deficiency can be confirmed with an assay that measures the uptake of 14 C- O -methyl- d -glucose in erythrocytes. Treatment is with a ketogenic diet, which is usually successful in controlling the seizures but does not always prevent the microcephaly, developmental delay and ataxia. There may be a transient form of the disorder which does not require long-term treatment, but the three cases so far described may have had alternative explanations for the low CSF glucose levels, such as low-grade meningitis or subarachnoid haemorrhage ( ).
Biotinidase deficiency
This is one of the few treatable causes of resistant neonatal seizures, hence the importance of considering this rare autosomal-recessive condition. Biotinidase is responsible for biotin recycling and biotin is an essential cofactor for activation of the carboxylase enzymes. If biotinidase is absent, infantile or early-childhood encephalopathy, seizure disorder, dermatitis, alopecia, neural deafness and optic atrophy develop. In the neonatal period there is usually a skin rash, similar in appearance to seborrhoeic dermatitis. The disease can be diagnosed by simple fluorometric enzyme assay and treatment with biotin is cheap. The EEG shows a burst suppression pattern consistent with encephalopathy ( ).
Syndromic neonatal seizures
Benign familial neonatal seizures
This fascinating autosomal-dominant condition was first recognised in 1964 ( ). The onset is within the first week of life and seizures are dramatic, with 80% beginning on the second or third day of life. The seizures ceased after 6 weeks of age in 68% of the largest kindred reported, and rarely persist beyond 6 months ( ).
Seizures show an initial tonic phase with cyanosis followed by clonic movements of the whole body ( ). There is usually a family history of neonatal seizures but they are associated with normal psychomotor development, a normal interictal EEG and a favourable outcome. This rare condition has an autosomal-dominant inheritance with 85% penetrance, and mutations have been found in the genes situated on chromosomes 20q and 8q which code for a family of voltage-gated potassium channels (M (for muscarine) channels). Benign familial neonatal convulsions (BFNC) are thus an example of a ‘channelopathy’. M channels can be kept open with a new AED, retigabine, and it has been suggested that this drug might hold promise for other types of neonatal seizure apart from those in BFNC.
In contrast to pyridoxine-dependent seizures, these fits can be controlled by conventional medication and the prognosis for development is excellent.
Benign non-familial neonatal seizures (fifth-day fits)
This benign self-limiting condition reached epidemic proportions in some Australian maternity units in the late 1970s ( ). Reports also came from France, but only two cases have been seen in Nancy since 1985 and the diagnosis has not been made in Camperdown since 1989 ( ; ). Seizures occur between day 1 and day 7 after birth and are usually partial clonic, rarely generalise and apnoea is common ( ). Seizures last for 1–3 minutes but can become frequent, leading to status epilepticus. The neurological state is usually normal at the onset of seizures and there is no family history of seizures. The interictal EEG may be normal, show generalised or multifocal discharges or show characteristic bursts of sharp waves, sometimes called ‘theta pointu alternant’.
The cause remains a mystery, although low CSF zinc was found in a few cases ( ). A few years ago we cared for a baby with this condition who had marked tonic-clonic seizures which are otherwise very unusual in the newborn period; she has thrived ( ).
Benign neonatal sleep myoclonus
Neurologically normal term neonates can present with BNSM, which is characterised by recurrent rhythmic jerks during sleep and a normal EEG. Jerks disappear during wakefulness and resolve within 3 months. A recent review of the literature which included 164 cases of BNSM found that repetitive jerks were located primarily in the distal part of the extremities, usually the arms but also the legs and very occasionally the face and abdomen ( ). Myoclonus was seen in quiet sleep predominantly but some reports did describe jerks during active sleep. There was also some evidence that an exacerbation of myoclonic jerks occurred when some infants were erroneously treated with AEDs. An interesting finding in this series was that the repetitive jerks did not always stop with gentle restraint and had not resolved by 3 months in one-third of cases. No treatment is required for BNSM and parents should be reassured that the jerks will cease eventually.
Epileptic encephalopathies
Ohtahara syndrome (early infantile epileptic encephalopathy)
This syndrome was first described by Ohtahara and colleagues in 1976 and is one of the age-dependent epileptic encephalopathies (the others being West’s syndrome and Lennox–Gastaut syndrome). Seizures usually develop within the first 10 days of life and may occur as early as the first hour after delivery. The seizure types in Ohtahara syndrome are variable, with the most frequent type being spasms, which may be either generalised and symmetrical or lateralised. Spasms may occur singly or in clusters in both awake and asleep states. Generalised tonic-clonic seizures can develop later in the syndrome. Seizures are usually accompanied by a severe encephalopathy, and are resistant to treatment. Soon after the onset of seizures, the infants become inactive and hypotonic. The syndrome is now believed to be mainly attributable to a cerebral malformation ( ).
The EEG shows a characteristic burst suppression pattern and can be distinguished from the pattern seen in other encephalopathies ( ). According to the Japanese group that has published most of the seminal works on this condition, the bursts must consist of high-amplitude non-synchronised paroxysms lasting 2–6 seconds and the suppression phase must show less than 10 µV or a flat tracing and continue for 3–5 seconds ( ). Suppression and burst phases must appear alternately and regularly every 5 seconds or more and should be seen in both sleep and wake states. During seizures, desynchronisation of the burst suppression pattern is seen. The prognosis is very poor: psychomotor development is arrested and severe neurological abnormalities such as spastic diplegia, hemiplegia, tetraplegia, ataxia, or dystonia develop ( ). Vigabatrin has been tried in a few cases ( ).
Early myoclonic epilepsy (EME)
This rare syndrome, originally described by Aicardi, presents with erratic, fragmentary myoclonus in the first month of life, evolving into focal seizures and infantile spasms. Seizure manifestations include partial or fragmented myoclonus, massive myoclonias, partial motor seizures and tonic spasms. Myoclonias may shift from one part of the body to another and usually persist in sleep. Normal background activity is absent. The background EEG shows a burst suppression pattern with complex bursts of spikes, sharp waves and slow waves lasting for 1–5 seconds in both waking and sleep. The burst suppression pattern seen in EME is similar to that in Ohtahara syndrome but the burst phase is shorter with longer periods of suppression than Ohtahara syndrome ( ). The fragmented myoclonias usually have no EEG correlate, whereas massive myoclonias may be synchronous with the bursts. The clinical course of EME is severe and antiepileptic agents and corticosteroids or adrenocorticotrophic hormone have not been effective. Metabolic aetiologies are predominant in EME, whereas malformative aetiologies predominate in Ohtahara syndrome.
Investigation
Essential laboratory investigations include:
- •
blood glucose
- •
serum calcium, ionised calcium if possible
- •
serum magnesium
- •
arterial pH, blood gas
- •
lactate
- •
serum sodium
- •
serum urea and creatinine
- •
lumbar puncture
- •
blood culture
- •
cranial ultrasound scan.
If the cause is not revealed, second-line investigations are suggested in Table 40.4 and include specimens for virology and a congenital infection screen, MRI, samples such as hair or urine to look for maternal ‘street’ drugs, urinary and blood amino acid estimation, chromosomal analysis, blood ammonia and measurement of urinary organic acids. Consideration should be given to a trial of pyridoxine in resistant cases. The value of an EEG examination has already been discussed and an EEG should be obtained if at all possible, and certainly should be done in difficult cases. MRI is superior to computed tomography for most purposes, and should be considered in all cases, and certainly if the cause is not revealed by first-line investigations. Even if the cause is found, the information obtained from MRI can give valuable information about the prognosis.
|
Treatment
Indications for treatment
Most babies are still treated on the basis of a clinical diagnosis alone, and treatment is also monitored this way. Continuous EEG studies show that a considerable electrographic seizure burden often remains after anticonvulsant treatment begins, due to electroclinical dissociation ( ). Whether or not treating to electrical quiescence can improve the outcome is not known, and as yet there is no anticonvulsant regimen that will achieve this in all cases ( ). In the current state of knowledge most neonatologists would treat a baby who had more than three clinical seizures in an hour, or a single clinical seizure lasting more than 3 minutes; this remains reasonable practice, although every attempt should be made to obtain an EEG.
General guidelines
Treatment is best started intravenously as absorption is erratic from intramuscular or enteral administration, and the neonate has little muscle mass. Facilities to site and maintain intravenous lines and to institute artificial ventilation are necessary before treating seizures, as most of the available drugs depress respiration and ventilation can become inadequate due to frequent convulsions or the effects of treatment. The high total body water of the neonate means there is a large volume of distribution, hence the relatively large loading doses suggested in Table 40.4 . Many of the drugs are protein-bound and can interact with other drugs and bilirubin. Therapeutic hypothermia does prolong the half-life of many drugs, including phenobarbital, and appropriate doses are still being worked out. In cooled babies we give a loading dose of 20 mg/kg phenobarbital with a maximum of two further doses of 10 mg/kg/dose and then check a level.
Initiating treatment
Phenobarbital remains the current first-line treatment of neonatal seizures worldwide, in spite of evidence that it is effective in only about a third of cases and there is concern about the effects of this drug on brain development, including apoptosis ( ; ; ; ; ). Recently published surveys from Israel, Australia and the USA indicate that there are large variations in practice, both between neonatologists and neurologists and between countries ( ; ). Off-label use of AEDs is not uncommon ( ). In a recent European survey, showed almost unanimous use of phenobarbital as a first-line drug, followed by midazolam, phenytoin and lidocaine as second- and third-line drugs.
Phenobarbital enhances GABA actions. The qualitatively distinct action of GABA on the neuronal membrane in early development may explain why phenobarbital is often ineffective in newborns: it facilitates the passive outflow of chloride down its electrochemical gradient, depolarising and exciting neurons (as compared with its actions on mature neurons, which have low Cl − concentrations).
Phenytoin is our current second-line choice, although this drug needs to be used with caution (and given slowly intravenously) in babies with hypoxic ischaemia who often have cardiac depression. About a third of babies fail to respond to a combination of phenobarbital and phenytoin: they are usually suffering from severe hypoxic–ischaemic encephalopathy and their prognosis is poor. The choice of third-line anticonvulsant varies, but one of the benzodiazepines is often chosen. Doses of commonly used anticonvulsant drugs are given in Table 40.5 . Midazolam is effective for the control of status epilepticus in adults and children, but has shown varying results in babies ( ; ; Sirsi et al. 2008). Recent evidence from a number of small studies does seem to indicate that midazolam may be a useful treatment for seizures in babies, but the exact dose and dosing regimen have not been established ( ; Sirsi et al. 2008). In addition, there are concerns about safety: midazolam can cause myoclonus in preterms ( ).
DRUG | INITIAL DOSE | ROUTE | MAINTENANCE DOSE | ROUTE | HALF-LIFE | MODE OF EXCRETION | Notes | Therapeutic level |
---|---|---|---|---|---|---|---|---|
Phenobarbital | 20–40 mg/kg | IV | 4–5 mg/kg/24 h | O | 100–200 h | Hepatic P 450 cytochrome oxidase | Slow oral absorption: liver enzyme inducer | 20–40 mg/l 90–180 µmol/l |
Phenytoin | 20 mg/kg slowly (1 mg/kg/min) | IV | 5 mg/kg/24 h in two doses | IV/O | 20 h (75 prems) | Liver glucuronidation | Vitamin K antagonist; risk of extravasation, cardiac toxicity and purple-glove syndrome | 10–20 mg/l 40–80 µmol/l |
Paraldehyde | 0.3 ml/kg (0.6 ml/kg mixture) PR | rectal | For rectal use dilute 1 : 1 with arachis oil, give no more than t.d.s. | Rectal | 7–27 h | Mainly liver, some lungs | Protect from light and plastic | |
Diazepam | 0.3 mg/kg | IV | 0.3 mg/kg | IV | 20–60 h | Liver glucuronidation | Flumazenil is an antidote to diazepam and midazolam; rapid clearance from the brain limits value | |
Clonazepam | 100 µg/kg | IV | 4 µg/kg/h | IV | 30 h | Liver glucuronidation | Tends to increase salivation and bronchial secretions | 30–100 mg/l |
Midazolam | 60 µg/kg | IV | 150 µg/kg/h | IV | 6–12 h | Liver glucuronidation | Reports of myoclonic jerks in preterm babies | |
Valproate | 20 mg/kg | IV | 10 mg/kg/12-hourly | O | 26–47 h | Hepatic | GABA modifier. Increased ammonia levels. Hepatotoxicity may be a problem but not yet described in babies | 40–50 mg/l 275–350 µmol/l |
Lidocaine | 2–4 mg/kg | IV | 2 mg/kg/h maximum 6 mg/kg/h and for no more than 48 hours | IV | 200 min | Liver and kidney | Toxic metabolites accumulate in 24 hours | 2.4–6 mg/l in adults; little known about therapeutic levels in babies |
Pyridoxine | 50–100 mg | IV | 5–10 mg/kg/day | O | EEG monitoring in ICU for the initial dose | |||
Pyridoxal phosphate | 30 mg/kg/day | |||||||
Folinic acid | 4 mg/kg/day | Folinic acid-responsive Seizures are very rare |
Midazolam has been evaluated in an open comparison with lidocaine as a second-line treatment in babies whose seizures failed to respond to phenobarbital: seizures were monitored with continuous video-EEG ( ). Six babies received either clonazepam or midazolam, but none responded. Others have had better success with midazolam, one study using very high doses of 1000 µg/kg/h ( ; ). An adequately powered randomised controlled trial of midazolam is clearly warranted.
Lorazepam is more popular in the USA ( ; ). Paraldehyde, formerly given rectally, can work well intravenously but is currently difficult to obtain in Europe and is not available in the USA. Those who have used intravenous paraldehyde as a third-line anticonvulsant report success in over 80% of cases with 200 mg/kg given intravenously as a single slow infusion repeated 12-hourly if required ( ). The problem with many of the studies which evaluate AED treatments in babies is that the outcome measure is clinical seizure control, which can be very misleading.
Lidocaine has a very narrow therapeutic range and accumulates in the blood, so that it can only be given as an infusion for 48 hours. There are reports of success with this agent, mainly from Scandinavia ( ; ). Much more information is required before this drug can be recommended for routine use. A baby who has been given phenytoin already should not receive lidocaine because of the risk of cardiac toxicity: the drugs act on the same sodium channels ( Table 40.5 ). Newer AEDs such as topiramate and levetiracetam have been anecdotally reported to improve acute neonatal seizures ( ; ; ). It is also not known how long to continue treatment for neonatal seizures (see below), and how the length of treatment impacts outcome.
An age-dependent high expression of a chloride cotransporter is thought to be responsible for the high incidence of seizure in the newborn period and the lack of response to conventional drugs used in older children and adults ( ). Bumetanide, a commonly used loop diuretic, blocks this specific age-dependent cotransporter and has been shown to reduce seizure burden significantly in immature animals. More recently, animal experiments suggest that bumetanide in combination with phenobarbital may be even more effective in suppressing seizures in the immature brain ( ).
Bumetanide has been used as a diuretic in term and preterm babies for around 30 years and several studies have validated its efficacy and safety, including pharmacokinetics and dose-finding studies ( ). It is considered a safe drug in the neonatal period, even in critically ill infants ( ; ; ; ; ; ; ; ; ; ; ). Studies in animals have suggested 0.1–0.2 mg/kg as the optimal dose to block the NKCC1 cotransporter, which is at the upper range of the dose used as a diuretic. Two randomised studies of bumetanide for neonatal seizure control are underway in the USA and Europe.
Duration of treatment
Concern about the effects of anticonvulsant treatment on the developing brain means that most British neonatologists would only discharge a baby on maintenance phenobarbital if the neurological examination was abnormal, although only 3% of US neonatologists discontinued treatment prior to discharge ( ). Only two of 55 Swedish infants discharged without medication relapsed ( ). Some perform an EEG at a month or prior to discharge, and discontinue anticonvulsants if this was normal. If babies are discharged on anticonvulsants, consider discontinuation of treatment if they remain seizure-free at 9 months. Babies can be allowed to ‘grow out of’ their dose, gradually reducing drug levels.
Maintenance anticonvulsants
Phenobarbital in a dose of 5 mg/kg/day is the usual maintenance anticonvulsant chosen for the newborn. There is very little experience with alternative maintenance therapy at the present time and combinations are best avoided. Phenytoin is not a good choice for long-term therapy. Resistant cases should be treated with a combination of phenobarbital and carbamazepine, although sodium valproate can be successful in some cases.
Prognosis
The prognosis depends largely on the cause of the seizures, being worse for those with hypoxic–ischaemic encephalopathy, meningitis and cerebral malformations than hypocalcaemia, benign familial neonatal seizures, subarachnoid haemorrhage or stroke ( ) ( Table 40.6 ). Mortality and morbidity are greater in preterm babies ( ; ). There is some evidence suggesting that seizures are independently associated with a poor outcome but recent evidence is conflicting ( ; ). The background EEG can be helpful, and a normal background EEG with well-organised sleep stages has consistently been shown to be associated with an 80% chance of normal development ( ; ; ). The background EEG features of encephalopathy evolve over time, and it is important not to prognosticate from a recording made too early; both we and others have shown that a low-voltage EEG seen in the first 6 hours of life can recover ( ; ; ).
DEAD | HANDICAP | NORMAL | REFERENCE | |
---|---|---|---|---|
HIE grade II, III | 50% | 25% | 25% | |
Preterm | 58% | 23% | 18% | Scher et al. (1993a); Watkins et al. (1988); van Zeben-van der Aa et al. (1990) |
Meningitis | 20% | 40% | 40% | |
Malformations | 60% | 40% | ||
Late-onset hypocalcaemia | 100% | |||
Hypoglycaemia | 50% | 50% | Koivisto et al. (1972) |
This evolutionary pattern may have changed somewhat with the advent of therapeutic hypothermia and two studies using continuous aEEG monitoring have suggested that recovery of the EEG is delayed when babies with hypoxic–ischaemic encephalopathy are cooled ( ; ). Rewarming seizures are occasionally seen ( ). The number of electrographic seizures is not in general an indicator of prognosis, nor is the clinical seizure type. Some have suggested that the outcome is worse for babies with a large number of independent electrographic seizure foci, but this work requires confirmation ( ). There is increasing consensus that seizures (including electrographic seizures which are clinically silent) which persist despite third-line AEDs carry a poor prognosis ( ). Adverse outcomes include cerebral palsy and microcephaly with significant learning difficulties.
The risk of subsequent epilepsy after neonatal seizures also depends on the aetiology, and is more likely if spike and sharp-wave activity persists on the EEG at 3 months ( ). The later seizure type includes infantile spasms, minor motor seizures, complex partial and tonic-clonic seizures, which often only emerge after a year or so. In a Dutch study, the incidence of postneonatal epilepsy after treatment of clinical and subclinical neonatal seizures detected with continuous aEEG was 9.4% ( ).
Intracranial haemorrhage and perinatal stroke (arterial and venous) at term
- Divyen K Shah
- Janet M Rennie
Introduction
Intracranial haemorrhage (ICH) and stroke in term newborn represent an important and probably underrecognised group of conditions which require a high index of suspicion for diagnosis. The diagnosis should be considered in babies exhibiting neurological dysfunction such as seizures and encephalopathy. Symptomatic ICH can coexist with other pathologies such as hypoxic–ischaemic encephalopathy (HIE) or sepsis, and may be associated with serious consequences for the infant. Serious ICH following a difficult delivery is well recognised, and, although obstetric trauma is much less common than in the past, cases still occur, with disimpaction of the fetal head at caesarean section in the late second stage emerging as an increasing problem for the newborn, in our experience. With improved neuroimaging techniques, we now know that asymptomatic ICH is quite common and is generally associated with good outcome.
ICH may be classified according to the intracranial compartment in which the blood is found on imaging into extra-axial bleeds (outside the brain) which include subgaleal (subaponeurotic), extradural, subdural and subarachnoid, or intra-axial bleeds, including intraventricular and intraparenchymal bleeding ( Fig. 40.16 ). Many babies will have haemorrhage in more than one location or compartment of the brain. ICH has also been categorised according to its position with respect to the tentorium cerebelli, an almost horizontal fold of dura, which separates the occipital cerebral lobes above from the cerebellum below into supratentorial and infratentorial compartments. Although subgaleal or subaponeurotic haemorrhage is extracranial, it will also be considered in this chapter because of its potentially serious nature.

Clinically, the subtype of ICH can be recognised by the site and pattern of distribution of the collection of blood on imaging. The subtypes of ICH will be considered together as a group initially because they may share similar aetiologies such as birth trauma and bleeding diatheses, they are often found to coexist and they all have the potential to cause permanent neurodisability.
Incidence and prevalence of intracranial haemorrhage
The true incidence of ICH is difficult to ascertain. Serious ICH related to traumatic birth is much less common with improved obstetric care ( ). The prevalence of ICH revealed using magnetic resonance imaging (MRI) is much greater than ultrasound studies would suggest ( ). Recent MRI studies confirm the early pilot MRI results ( ), showing that asymptomatic ICH is quite common. In an MRI study of 111 asymptomatic newborns in Sheffield, used an 0.2 T magnet to detect subdural haemorrhage (SDH) in nine (8%) in the first 48 hours of life. In an American MRI study using a 1.5 T magnet in which 101 newborns were imaged in the first week of life, found 46 (46%) had a supratentorial SDH; 20 infants also had an additional infratentorial SDH. In both studies, all haemorrhages had resolved by 1–3 months’ age. At 2 years of age, no child followed up by Rooks and colleagues had a motor deficit, and the incidence of speech and language delay (6%) was no greater than expected. More recently. using a 3 T magnet, found 16 subdural, two subarachnoid and six parenchymal haemorrhages in 88 babies, giving a prevalence of 26%.
The incidence of symptomatic ICH is lower, and has been estimated at around 3 per 10 000 live births ( ). A large epidemiologic study of neonatal intracranial injury related to the mode of delivery in almost 600 000 women from California suggested that the incidence of ICH varied from 1 in 664 infants delivered by forceps to 1 in 2750 delivered by caesarean section without labour ( ). Subgaleal haemorrhage was not included in this study.
Risk factors for intracranial haemorrhage
Difficult delivery
The main risk factor for perinatal ICH is dystocia (difficult child birth: Table 40.7 ). Disimpaction of the fetal skull at caesarean section has become a more common cause of ICH due to trauma in our experience, and that of others, as more caesarean sections are performed in the second stage of labour when the head is deeply engaged ( ). There may be an associated skull fracture ( Fig. 40.17 ). From an epidemiologic study from California, ICH occurred in 1 of 2750 infants delivered by caesarean section without labour, 1 in 1900 infants delivered by normal vaginal delivery, 1 in 907 infants delivered by caesarean section during labour, 1 in 860 infants delivered by vacuum extraction and 1 in 664 infants delivered by forceps, in nulliparous women ( ). In a Sheffield study of asymptomatic infants ( ), five of nine infants with SDH were delivered by forceps after failed ventouse, one had had a traumatic ventouse delivery and the other three were born by normal vaginal delivery. Studies show that ICH is commoner after forceps delivery ( ) when compared with vaginal delivery, and it is also commoner after a trial of forceps following a failed ventouse ( ) when compared with delivery by ventouse alone, forceps alone or a normal vaginal birth.
Maternal |
|
Mode of delivery |
|
Infant |
|




Maternal associations include primiparity, grand multiparity, pre-eclampsia and placental abruption. Neonatal factors include HIE, low Apgar scores and any bleeding disorder, including thrombocytopenia and haemophilia.
Neonatal bleeding disorders
ICH in babies with HIE or sepsis complicated by a coagulopathy is not uncommon. A study of 66 newborns with ICH showed that at least 12 (19%) had platelet counts of less than 50 × 10 9 /L within the first 48 hours of life and were specifically associated with intraparenchymal haemorrhage and a more severe grade ( ).
Neonatal alloimmune thrombocytopenia ( Ch. 30 ) carries a substantial risk of ICH in the newborn, probably around 1 in 10, many of which occur during intrauterine life ( ; ). These babies are prone to all types of extra-axial and intra-axial haemorrhage ( ).
Primary inherited coagulation disorders are rare but may present with ICH in the newborn. Haemophilia A and B are the commonest of these ( ; ; ). Recent studies suggest that 3.5–4% of all haemophilic boys develop ICH in the newborn period, a figure which represents a significantly increased risk compared to the normal population. Despite the poor outcome, the debate about prophylactic factor VIII still rages ( Ch. 30 ) and of course many are first-born male babies, and the diagnosis is not suspected until the ICH is already very large. Very rarely, deficiency of factors X, VII, XIII and fibrinogen presents in the newborn period. These deficiencies are autosomal-recessive traits and as such are commoner in communities with consanguinity. Ineffective vitamin K prophylaxis, parental refusal of vitamin K and deficiency remain important causes of ICH. Babies with liver disease often present with vitamin K deficiency bleeding, usually intracranial ( ; ).
Structural anomalies
Arteriovenous malformations, aneurysms and tumours are rare causes of ICH at term.
Extracorporeal membrane oxygenation (ECMO)
The need for ECMO or cardiac surgery increases the risk that a term baby will develop symptomatic intraventricular haemorrhage (IVH) ( ; ).There are probably many reasons for this, including anticoagulation, sepsis and sinus thrombosis. Some forms of ECMO require ligation of one carotid artery.
Haemorrhage into an area of venous infarction
It is now appreciated that many parenchymal haemorrhages at term are due to bleeding into an area of venous infarction ( ). This is particularly likely in thalamic haemorrhage (see below) ( ). Hence, bleeding into the deep grey matter at term is often the first sign of a cerebral sinovenous thrombosis (CSVT: see below).
Clinical presentation of intracranial haemorrhage
When signs of ICH occur, the most commonly reported are seizures and apnoeas, but subtle clues such as poor temperature regulation or reluctance to feed can be present. Babies with focal lesions may be well at birth and present with focal seizures on the postnatal ward over the next 24–48 hours. All babies with neonatal encephalopathy should have a careful clinical neurologic examination; pupils and tone should be checked, paying particular attention to symmetry and focal signs.
Investigations
A full blood count may reveal anaemia and thrombocytopenia; coagulation screen may be deranged.
Lumbar puncture
Lumbar puncture is not recommended to assist in the diagnosis of ICH. Clearly, in a sick infant with accompanying signs of neurological dysfunction such as encephalopathy and raised intracranial pressure, lumbar puncture is contraindicated.
If uniformly blood-stained cerebrospinal fluid (CSF) is obtained when a diagnostic lumbar puncture is undertaken it should raise a suspicion of ICH, particularly subarachnoid haemorrhage (SAH).
Electrophysiology
Digital bedside cerebral function monitoring carried out while a conventional multichannel electroencephalogram (EEG) is awaited may reveal electrographic seizures ( p. 1084 ). Conventional multichannel EEG may show focal seizures localizing to the focus of the lesion.
Neuroimaging
Cranial ultrasound (CUS) remains the main modality of imaging of the newborn brain at the bedside and is generally a good technique for imaging intraventricular and parenchymal bleeds. In experienced hands, CUS allows visualisation of bleeds into the cerebellum as well as the basal ganglia and thalami. CUS is of limited value for the detection of subdural haemorrhage and SAH unless there is obvious midline shift.
Computed tomography (CT) scanning is easily available in most centres, where access to MRI may be more limited. CT entails a significant dose of X-ray irradiation, but provides images with a short acquisition time, albeit with low resolution. It is good for visualising skull fractures and will adequately detect modest-sized extra-axial bleeds, although MRI is likely to detect smaller bleeds. The radiation exposure of CT is justified when significant ICH is suspected, if MRI is not available.
MRI, where available, is the imaging modality of choice. A combination of MRI sequences such as T1, T2 and diffusion weighting will allow detection of most intra- and extra-axial bleeds at various stages of evolution. MR angiography, including the neck vessels, should be performed when stroke is suspected (see below).
Pathogenesis of subdural haemorrhage
Since SDH is a common finding after non-accidental injury, an understanding of SDH following birth trauma and its natural history is important ( Table 40.8 ). SDH represents a collection of blood in the dural border cell layer adjacent to the arachnoid membrane ( ). Small veins from the dorsal convexity of the cerebral hemisphere drain into superficial cerebral veins, which in turn coalesce to form 10–18 bridging veins which cross the subarachnoid space through the arachnoid membrane into the dura and drain into the superior sagittal sinus. Traditionally, the mechanism ascribed to haemorrhagic collections on the surface of the brain such as subdural haemorrhage as well as SAH has been a tear in these bridging veins due to distorting forces.
|
Mechanism of subdural haemorrhage
Serious SDHs have been related to:
- •
tentorial and falx tears
- •
occipital diastasis
- •
convexity collections.
Tentorial and falx tears
Prolonged and difficult labour caused by cephalopelvic disproportion as the baby’s head passes through the birth canal has been postulated to cause stretching of the dura and tears to the falx and tentorium ( Fig. 40.18 ). The tentorium cerebelli has the transverse sinus running in the horizontal plane and the straight sinus running in the vertical plane (both posteriorly). The superior and inferior sagittal sinuses both meet the straight sinus within the tentorium. A severe tentorial tear can disrupt these large veins, causing haemorrhage into the posterior fossa. A tear to the falx is less common, causing damage to the inferior sagittal sinus, with blood seen above the corpus callosum on imaging. Most perinatal SDHs are peritentorial ( ).


Occipital osteodiastasis
This is the result of serious trauma, and was often found in term infants who died after breech extraction, which is now rarely seen. The occipital bone has four parts which are not completely ossified at birth, and are separated by cartilage. Hyperextension of the head during breech extraction can exert enough pressure on the occipital bone to cause traumatic separation at the cartilaginous joint at the junction of the squamous and lateral or condylar parts, with injury to the occipital sinus causing haemorrhage into the posterior fossa ( ). suggests that this lesion may be more common than has been recognised because it is easily missed at postmortem, in the absence of imaging.
Convexity haemorrhage
These are typically crescentic-shaped supratentorial collections lying on the convex surface of the brain which have traditionally been thought to be caused by ruptured bridging veins. Hence depending on the site of the rupture of the vessel, they may also be associated with SAH.
Asymptomatic subdural haemorrhage
In view of the fact that asymptomatic SDH is common, propose that the relatively large inner vascular plexus in the dura membrane of the newborn may be the origin of many SDHs, as opposed to the bridging veins.
Clinical features of subdural haemorrhage
Convexity haemorrhage
Most SDH is asymptomatic. Clinical signs may be non-specific and a high degree of vigilance and index of suspicion is important after a difficult delivery. The most common clinical features are seizures and apnoea. Seizures may be focal, particularly in cases of a supratentorial convexity haematoma overlying a cerebral contusion. Careful examination in such a baby may reveal neurological signs. A major convexity SDH may cause herniation of the temporal lobe through the tentorial notch and a distinct palsy of the third cranial nerve, with a dilated pupil that is poorly reactive to light.
Babies are often well in the first few hours of life but then go on to develop signs of raised intracranial pressure, including bulging fontanelle, poor feeding, irritability and seizures.
Infratentorial haemorrhage
Major infratentorial haemorrhage is now quite rare, although the MR studies mentioned above show that most subdural bleeding is peritentorial. Babies may be born with depressed Apgar scores or deteriorate later due to infratentorial clot swelling and compression of the brainstem. The clot may block free flow of CSF, causing hydrocephalus. These infants become obtunded, with opisthotonic posturing, bradycardia, loss of respiratory drive and dilated, poorly reactive pupils. Death may ensue over a matter of hours unless the pressure is relieved.
Clinical management and prognosis following subdural and intracranial haemorrhage
Medical management
Most ICHs can be managed conservatively. Seizures should be treated. Thrombocytopenia should be corrected with platelet transfusion and deranged coagulation should be treated with fresh frozen plasma, vitamin K and cryoprecipitate appropriately. Remember that a first-born male may be the first in his family with haemophilia, and if the diagnosis is missed and the baby dies, the family cannot benefit from antenatal diagnosis in future pregnancies. The baby may require respiratory and haemodynamic support.
Neurosurgical management
Neurosurgical input may be appropriate in cases of symptomatic ICH, particularly in the presence of an associated skull fracture, midline shift, signs of raised intracranial pressure and focal neurological signs. Sixteen of 53 infants with ICH in Holland ( ) developed hydrocephalus due to obstruction of free flow of CSF. Imaging revealed midline shift in 21/53 infants, three of whom were treated with craniotomy.
The place of surgical intervention, particularly in infratentorial bleeds, is a subject for debate. The consensus view seems to be that, in the absence of brainstem compression or hydrocephalus, conservative management is appropriate. Indications for neurosurgery include the following ( ):
- •
signs of life-threatening brainstem compression such as apnoea, bradycardia and hypotension
- •
acute obstructive hydrocephalus with raised intracranial pressure
- •
a very large clot in the posterior fossa.
Neurosurgical procedures used in these circumstances include aspiration of clot using needle aspiration techniques or burrhole trephine, external ventricular drain for obstructive hydrocephalus and posterior fossa craniectomy. In a series of 15 infants with infratentorial SDH ( ) surgical evacuation was performed in eight. Outcomes were similar for both the conservatively managed and surgical intervention groups. Five of the 15 infants had moderate or profound delay.
Prognosis
reported cerebral palsy in four of 34 survivors of their series of 53 ICHs. Adverse outcome was associated with intraparenchymal bleeds and associated HIE. Other studies also show that coexisting HIE in infants with ICH puts them at increased risk of adverse outcome compared to infants with ICH alone ( ). Some children develop epilepsy.
Extradural haemorrhage
Extradural haemorrhage is rare in the newborn. It is typically a biconvex collection between the skull bone and the dura ( Fig. 40.19 ). There may be a skull fracture immediately adjacent to the collection. On high-resolution imaging, the dura may be seen between the brain and such a collection. These collections do not cross suture lines, as dura attaches to the skull at these sites.


reported a series of 15 newborn infants with extradural haematoma. Eight had been delivered by instrumental delivery and five by caesarean section following a trial of vaginal delivery. Most presented within 24 hours with seizures (7/15) or hypotonia. Ten of the 15 had a skull fracture, most commonly of the parietal bone. All infants also had retinal haemorrhages. Seven infants were treated with craniotomy and two had ventriculoperitoneal shunts.
Subarachnoid haemorrhage
The pathogenesis of primary SAH is not well understood, but it probably represents bleeding into the subarachnoid space caused by ruptured blood vessels, including bridging veins, and may occur with convexity SDH. The incidence of SAH in the newborn is not known but is commonly noted at postmortem in babies who have died from unrelated causes.
Babies may be asymptomatic. Seizures may be the main presenting feature and the infants may be well between seizures. Rarely, infants may die from a catastrophic SAH. SAH is recognised from CT and MR images as the blood fills CSF cavities, including sulci, cisterns and fissures. The diagnosis should be considered if blood-stained CSF is obtained from a lumbar puncture in an infant with cerebral dysfunction.
Intraventricular, intraparenchymal, thalamic and cerebellar haemorrhage
Intraventricular, intraparenchymal and cerebellar bleeds are commoner in preterm infants. In term infants the mechanism of the intraventricular and intraparenchymal bleeds is less well understood. IVH in term infants may occur in association with hypoxia–ischaemia, birth trauma, ECMO therapy, congenital heart disease, disseminated intravascular coagulation (DIC), sepsis and thrombophilic disorders. Intraventricular and parenchymal bleeds in term infants may occur with arterial and venous infarcts.
Thalamic haemorrhage at term typically presents with seizures, and unusual eye signs may be present ( ; ). Babies who were apparently well at birth can become lethargic, feed poorly and develop seizures ( ). Figure 40.20 shows the characteristic imaging findings in a term baby with thalamic haemorrhage. From an early series of 19 term infants diagnosed with IVH on CT scan prior to 1 month of age, 12 were found to have thalamic haemorrhage, leading the authors to conclude that the thalamic haemorrhage was the source of the IVH ( ). Bleeds into the choroid plexus are also thought to be a source of IVH in the term infant. A more recent case series of 29 term infants with IVH detected using CT and MRI associated the cause of IVH with sinus venous thrombosis in nine infants ( ). Thalamic haemorrhage is probably due to sinovenous thrombosis in the majority of cases. As yet, there is little experience with anticoagulation in thalamic haemorrhage due to thrombosis of the deep venous system, or CSVT (see below) ( ).


IVH in term infants from sources such as the thalami, choroid plexus and sinus venous thrombosis lead us to conclude that IVH in term infants represents a different disease process to the common IVH in the preterm population, which is thought to arise mostly from the highly vascular germinal matrix near the subventricular zone.
In a series by , 14/29 (48%) had had perinatal complications such as fetal distress, low Apgar scores and HIE, 14/29 neonates had had major medical conditions such as congenital heart defects, the need for ECMO and DIC, and three neonates had thrombophilic disorders. In a third of the cases (9/29) there were no such associated perinatal or neonatal factors.
In the presence of HIE, term infants with IVH have a worse outcome than infants with IVH alone. Intraparenchymal haemorrhage is associated with a high risk of cerebral palsy ( ). Our understanding of the role of the cerebellum for neurodevelopment has increased considerably over recent years, and explains why term infants with cerebellar bleeds have a guarded prognosis.
Subgaleal (subaponeurotic) haemorrhage
Subgaleal haemorrhage is rare, but a massive and expanding collection represents an emergency in the newborn, and can be fatal. It is thought to be caused by the rupture of emissary veins, which connect scalp veins to the dural sinuses, causing bleeding into the subaponeurotic space between the outer periosteum of the skull and the epicranial aponeurosis which extends from the orbital ridges anteriorly to the nuchal ridge posteriorly. This space can hold up to 260 ml of blood ( ), which is almost as much as the total circulating volume of an average term newborn ( Fig. 40.21 ).

Subgaleal haematomas are commoner in infants delivered by instrumental delivery, particularly ventouse ( ). In a case series of 34 infants from St Louis, Missouri, 31 had been delivered by instruments – 21 vacuum, eight vacuum followed by forceps and two forceps ( ). Seventeen of the infants also had associated intracranial bleeds and six infants had skull fractures. The four (12%) infants who died had required large volumes of blood and blood products for treatment of coagulopathy and shock. In a prospective series from Malaysia, describe an incidence of 71/338 (21%) in babies born using the ventouse. These authors carefully examined all babies born by ventouse, checking head circumference and looking for fluctuant swellings several times over the first 24 hours of life. Two babies died, one from severe hypovolaemic shock and one from HIE. However, 58 (81%) developed some complication such as a coagulopathy, hyperbilirubinaemia or anaemia. The good outcome in this study was probably due to the active surveillance and increased awareness of the condition, which is often missed or appreciated too late.
Clinical presentation
A fluctuant swelling that crosses suture lines develops on the scalp after birth. Fluctuance, size and position help to distinguish subgaleal haemorrhage from cephalohaematoma, which does not cross the suture lines and does not become fluctuant until 48 hours of age. Some infants may deteriorate rapidly, sometimes when they are still in the labour ward. The head size may visibly increase and the infant becomes pale, sweaty and tachycardic. Some authors suggest that each centimetre increase in head circumference corresponds with a loss of 40 ml of blood into the subaponeurotic space ( ). The baby may develop systemic shock with DIC and coagulopathy.
Investigations
The haematocrit may drop rapidly. The baby may develop a metabolic acidosis with a rising lactate. The infant may be anaemic and thrombocytopenic and have deranged coagulation. Measuring the head circumference at frequent intervals can help.
Management
The key to management of this condition is a high index of suspicion and prompt and early recognition ( ; ). Delay in recognition of the condition may worsen outcome. recommends at least 8 hours of observations for all babies following a difficult instrumental delivery regardless of the need for resuscitation or Apgar scores. Aggressive management of the blood loss with blood and blood products is essential to avoid death from hypovolaemic shock and DIC, and secondary hypoxic–ischaemic brain damage.
Prognosis
A study of 42 cases from Taiwan found that 13 (31%) had a poor outcome: five died, four had epilepsy, three had severe auditory impairment and two had cerebral palsy. Outcome is related to the size of the haemorrhage and hence the degree of supportive treatment required. Infants who need mechanical ventilation, pressor support and large volumes of blood products, all reflecting the severity of haemorrhage, are at risk of worse outcome, as are factors such as coagulopathy, metabolic acidosis and renal impairment ( ). Good outcomes have been reported, as described above, following early recognition and aggressive replacement of blood and coagulation factors.
Perinatal stroke
Perinatal stroke can be defined as an area of damaged cerebral tissue resulting from focal disruption to cerebral blood flow secondary to arterial (perinatal arterial ischaemic stroke (PAIS): see below) or cerebral venous thrombosis (CSVT: see below) or embolisation, between 20 weeks of fetal life through the 28th postnatal day, confirmed by neuroimaging or neuropathological studies ( ). The growing importance of the topic was recognised by a recent National Institutes of Health workshop. Perinatal stroke is important because, apart from in later adult life, stroke is at no time more common than during this early period of development ( ). Stroke is also thought to account for up to 30% of hemiplegic cerebral palsy in term-born infants ( ). Haemorrhagic transformation is common in CSVT and rare in PAIS.
In this chapter we discuss only PAIS and CSVT. Borderzone, or ‘watershed’, infarction is usually secondary to prolonged partial hypoxic ischaemia, is associated with HIE, and is covered in this chapter section 4. Central to both conditions is the thrombophilic state of the circulating blood of the infant. The most common site for neonatal stroke is in the territory of the left middle cerebral artery. Perhaps half the children with neonatal stroke present in the first 3 days with focal seizures. Others are diagnosed later (outside the neonatal period), presenting with asymmetric neurologic findings or early hand preference. This latter group is labelled as ‘presumed perinatal stroke’, presenting later in infancy with asymmetric neurologic examination and early hand preference, and a higher proportion of this group develop hemiplegic cerebral palsy compared to infants diagnosed in the neonatal period.
Depending on the age of presentation, neonatal stroke may be classified as follows ( ):
- •
fetal ischaemic stroke – diagnosed antenatally or at autopsy in a stillborn fetus
- •
neonatal ischaemic stroke – diagnosed after birth or during the first 28 days of postnatal life, including in preterm infants
- •
presumed perinatal ischaemic stroke – diagnosed after 28 days, in infants in whom the ischaemia is thought to have occurred between 20 weeks’ gestation and 28 days’ postnatal age
- •
sinovenous thrombosis.
Thrombophilic state and the newborn
The newborn, like the pregnant woman, is in a thrombophilic state, as demonstrated in an elegant study that measured paired maternal and umbilical venous coagulation and anticoagulation factors ( ). Protein C, protein S, antithrombin III, fibrinogen and plasminogen levels were all significantly lower in the cord specimens.
In the newborn, acquired thrombophilic risk factors include transplacental acquisition of maternal antiphospholipid antibodies and inherited thrombophilic tendencies. Prothrombotic coagulation factors are present in half of neonates with perinatal stroke ( ). A meta-analysis of 22 cohort studies, with almost 2000 patients and 3000 controls, showed a statistically significant association between the thrombophilic traits of antithrombin deficiency, proteins C and S deficiency, factor V Leiden deficiency, factor II, methyltetrahydrofolate reductase (MTHFR) C677T mutation, antiphospholipid antibodies and elevated lipoprotein a with first stroke in childhood, with no difference found between PAIS and CSVT ( ). Children with combined thrombophilic disorders had the highest risk with an odds ratio of 11.86 (95% confidence interval 5.93–23.73).
Interestingly, in a study including 23 PAIS cases from the Israeli Paediatric Stroke registry in which the infants and parents had been screened for thrombophilic tendency, a risk factor was present in 18 mother–infant pairs but there was a mismatch in 15 cases so that the mother and the infant did not share one or more of the risk factors ( ). The most frequent risk factor in the study of was hetero- or homozygosity of the MTHFR C677T mutation, but this may only increase thrombogenesis when homocysteine levels are high. Inherited and acquired thrombophilic tendency is almost certainly important in neonatal stroke, but the whole story is, as yet, far from clear.
Perinatal arterial ischaemic stroke
PAIS is an increasingly recognised entity, largely due to increased use of MRI. PAIS is probably underrecognised, with incidences as high as 1 in 2300 live births quoted by centres with easy access to and frequent use of neonatal brain MRI, while other centres report lower incidences, of around 1 in 4000–5000 live births ( ; ; ). PAIS can affect both term and preterm babies ( ).
Aetiology and pathogenesis
The cause of PAIS is not always clear. The most commonly cited hypothesis is that of a placental embolus travelling into the arterial tree as a paradoxical embolus, because of the frequency with which the left middle cerebral artery territory is involved and because of the presence of a patent foramen ovale in fetal and early neonatal life. There is an association with persistent pulmonary hypertension which tends to support this mechanism ( ). Other associations include meningitis ( ), congenital heart disease, particularly if surgical repair is required ( ), intravascular catheters and ECMO ( Tables 40.9 and 40.10 ). Older studies report an association with perinatal hypoxic ischaemia, but these data are from the pre-MRI era and there were no cases of PAIS in the TOBY trial, which had strict entry criteria for the definition of HIE ( ). The previous association probably arose from the misclassification of babies with seizures; further, some babies with well-established infarction have developed acute neonatal signs. However, it remains the case that babies with stroke often have a complicated perinatal history ( ; ). Dissection of the internal carotid artery or its branches is very rare ( ): one of 215 German cases had arterial dissection demonstrated ( ). There is a single case report of a 5-month-old child who presented with a left hemiplegia and a right Horner’s syndrome. MRI revealed reduced blood flow in the internal carotid artery ( ). The cause was ascribed to a whiplash injury to the artery during fetal life, when the mother was involved in a road traffic accident. Congenital abnormalities of the neck vessels are also possible causes of PAIS.
|
Maternal risk factors |
|
Intrapartum factors |
|
Neonatal factors |
|
Clinical presentation
Commoner in males, the commonest clinical feature of PAIS in the neonatal period is seizures, particularly focal seizures in the first 3 days of life. Babies with PAIS have usually been well before presentation, and often remain well between seizures. Infants diagnosed outside the neonatal period commonly present with abnormal hand preference or evolving hemiparesis.
Investigations
A full blood count and haematocrit may reveal polycythaemia. Conventional or amplitude-integrated EEG (aEEG) may identify focal or generalised seizures; the background activity is often normal, and can be a useful guide to prognosis ( ). Echocardiography is indicated. An initial thrombophilia screen consisting of protein C, protein S, fibrinogen, antithrombin, lipoprotein a, anticardiolipin antibodies, lupus anticoagulant, partial thromboplastin time and international normalised ratio should be carried out, with a follow-up screen at 3–6 months (activated protein C resistance, factor V Leiden, prothrombin gene mutation, MTHFR, lipoprotein a, homocysteine, factors VIII/IX/X) ( ).
Imaging
In experienced hands, CUS may reveal echogenic foci at sites of infarction ( ), but the gold-standard investigation for PAIS is MRI ( ) ( Fig. 40.22 ). MR is preferable to CT, because of the radiation dosage associated with CT. CT is justified in an emergency, when it is important to determine whether or not there is a space-occupying subdural haematoma that might need neurosurgical intervention, or when there is thought to be significant ICH or trauma (bony windows can be obtained with CT). CT acquisition times are shorter and this can be a factor in critically ill babies.





Diffusion-weighted sequences may reveal restriction at affected territories of recent infarction ( Fig. 40.22d ) and sequences using MR angiography may reveal sites of arterial narrowing. studied conventional T1 and T2 images and diffusion-weighted images (DWI) that were first imaged in the neonatal period, from 21 infants with unilateral PAIS. All DWI images showed high signal intensity in the affected region until day 4 (similar findings to Lequin’s group in France). The cortex showed high signal intensity on T2 and low signal intensity on T1 until day 6, when the signal intensities reversed. Secondary changes in the thalamus and brainstem were seen in the first week of life and atrophic changes were seen after 4 weeks. These authors reported that the pattern of changes in signal intensities on conventional MRI and DWI were remarkably consistent in their patient group, leading them to conclude that PAIS in symptomatic term-born infants occurs within a very limited timeframe around birth. has provided helpful anatomical templates with which to identify the affected artery.
Management
During the acute phase of PAIS supportive measures such as adequate hydration, maintenance of normal glucose, pH and oxygen levels and anticonvulsants for seizures and antibiotics/antivirals for infection are important. Routine thrombolysis is not recommended as there are not sufficient data regarding its effectiveness or safety. In cases of PAIS with an ongoing documented cardioembolic source treatment with unfractionated heparin (UFH) or low-molecular-weight heparin (LMWH) may be appropriate ( ), but specialist advice should be sought.
Outcome
PAIS in the middle cerebral artery territory may result in hemiplegia in up to 50% of children, the likelihood being predictable from neonatal MRI ( ). Three-site involvement of the hemisphere, basal ganglia and posterior limb of the internal capsule is strongly associated with later contralateral hemiplegia irrespective of the size of the infarct ( ; ). The presence of pre-Wallerian degeneration in the cerebral peduncles also helps to predict the outcome ( Fig. 40.22e ) ( ). Later cognitive impairments, epilepsy and behaviour problems remain relatively common adverse outcomes ( ), with language delay in 25% ( ). In one group of 63 term and preterm children with PAIS, 54% developed epilepsy in childhood ( ), and cognitive difficulty is often present in those with epilepsy. Most children will walk, and recurrence is very rare in children without underlying cardiac disease.
Cerebral sinovenous thrombosis
Neonatal CSVT is rare, with a reported incidence of around 2.6 per 100 000 live births ( ). This is probably an underestimate, and the diagnosis is often missed due to the non-specific symptoms at presentation and the lack of sophisticated imaging in many centres ( ). CSVT can coexist with sepsis, meconium aspiration syndrome, meningitis and HIE. The commonest sinus to be involved is the superior sagittal sinus, but the transverse (lateral) or the straight sinus is also often thrombosed and many babies have more than one sinus involved ( ). CSVT propagation has been recognised with repeated MRI, and some would say that was an indication to consider anticoagulation (see below). Seizures are less commonly associated than with PAIS, and respiratory signs and hypotonia can be the clue to this diagnosis ( ).
Aetiology and pathogenesis
Risk factors are similar to that for PAIS, with haemoconcentration and dehydration particularly important ( ). Following CSVT venous pressure escalates in the occluded vessels and haemorrhagic infarction can develop in the region of brain drained by the sinus, because of venous congestion, vasogenic oedema, and increased capillary hydrostatic pressure ( ). The resulting brain injury may vary from venous congestion to the recognised ischaemic infarction involving the cortex, subcortical white matter and the deep nuclear grey matter. Haemorrhagic transformation of any infarcted cerebral tissue is the norm.
CSVT is frequently associated with secondary intraventricular, intraparenchymal and basal ganglia and thalami haemorrhage in term infants, and is thought to result from back pressure to distal draining veins. A 10-year review of patients from centres in the Netherlands ( ) identified 52 neonates with CSVT, of whom half had associated thalamic haemorrhage, half had associated IVH and 79% had intraparenchymal haemorrhage. As discussed above, and as demonstrated in Figure 40.20 , most term babies with thalamic haemorrhage have underlying CSVT.
Clinical features
In the Dutch study of 52 neonates with CSVT there was a male preponderance (75%) ( ). The commonest symptoms were seizures (81%), focal in a fifth of patients, followed by apnoeas. Fifteen per cent of infants had suffered sepsis or meningitis and 13% were born to mothers with pregnancy-related hypertension.
Investigations
The key to diagnosis of CSVT (arguably even more so than with PAIS) is a high index of suspicion so that appropriate and specific investigation to demonstrate diminished blood flow through cerebral venous sinuses can be carried out. Colour Doppler flow studies at the bedside may demonstrate reduced flow in affected venous sinuses. MR may show the clot, or venogram studies may identify regions of decreased flow-related enhancement in venous sinuses ( Fig. 40.23 ). In the Dutch study ( ), half of the infants had multiple sinus involvement, superior sagital sinus was affected in 23% and straight sinus in 15%. In addition to the associated haemorrhage, infarcts may be seen in the parasagittal white matter and the basal ganglia. All other investigations are as for PAIS.


Management
Rehydration is necessary in a dehydrated newborn. In an infant with primary polycythaemia dilutional exchange transfusion may be needed. Seizures and infections will require treatment with anticonvulsants and antibiotics respectively.
At present there are few data on therapeutic efficacy and safety of antithrombotic treatment in newborns with CSVT ( ), and international practice is hugely variable ( ). In the absence of significant ICH, particularly if the thrombus is noted to propagate, anticoagulation with UFH or LMWH may be used with specialist haematology input and close monitoring of anti-Xa, heparin and activated partial thromboplastin time levels. The aim should be rapidly to achieve anti-Xa levels of 0.5–1.0 U/ml ( ). Once commenced, treatment may need to be continued for several weeks until improved flow through the cerebral venous sinuses is established.
Outcome
Perinatal stroke is the leading known cause of hemiplegic cerebral palsy ( ). In all, 30–50% of infants with CSVT will go on to have a motor deficit ( ; ). Other common deficits resulting from perinatal stroke include epilepsy, deficits in language, vision and cognitive outcomes.
Hypoxic–ischaemic brain injury
- Nicola J Robertson
- Floris Groenendaal
Introduction
Definitions
Hypoxic–ischaemic brain injury of the term and near-term infant remains a significant problem throughout the world. Hypoxaemia is defined as the ‘diminished amount of oxygen in the blood supply’ and cerebral ischaemia as the ‘diminished amount of blood perfusing the brain’; ischaemia is the more important of the two forms of oxygen deprivation since it also results in deprivation of glucose which is also crucial in the genesis of neuronal injury ( ). Asphyxia is the result of an impairment of exchange of the respiratory gases oxygen and carbon dioxide. Thus, in addition to hypoxia, asphyxia has the important additional feature of producing elevated levels of carbon dioxide, which results in a number of additional metabolic and physiological features which include acidosis and increased cerebral blood flow (CBF) ( ). Hypoxia–ischaemia may occur acutely or chronically and is most commonly associated with maternal factors (hypotension, severe hypoxia), cord factors (prolapse, occlusion), placental factors (insufficiency and abruption) and uterine factors (rupture). Neonatal postnatal events such as shock, respiratory or cardiac arrest can also lead to hypoxic–ischaemic injury.
Neonatal encephalopathy (NE) is the clinical manifestation of disordered neonatal brain function in the term infant in the early neonatal period, manifested by respiratory difficulties, depression of tone and reflexes, subnormal level of consciousness and often seizures ( ). The aetiology of NE is varied, with many genetic, metabolic and infective conditions presenting with similar clinical signs (Hankins et al. 2003) ( Tables 40.11 and 40.12 ). The disorder is termed hypoxic–ischaemic encephalopathy (HIE) if there is evidence that intrapartum asphyxia is the cause of the encephalopathy resulting in neurologic depression or seizures. An important concept that is recurrent in many studies is that the human injury of HIE does not follow a wholly reproducible pattern: the etiology, extent of hypoxia or ischaemia, maturational stage of the brain, regional CBF and general health of the infant prior to the injury can all impact on the extent of brain injury as well as the outcome following injury.
CONDITION | EXAMPLE |
---|---|
Neonatal sepsis | Group B streptococcal septicaemia |
Congenital infections | Viral, toxoplasmosis |
Neuronal migration disorders | |
Congenital myotonic disorders | Congenital and transient myasthenia gravis, nemaline myopathy, Prader–Willi syndrome, peroxisomal disorders |
Lung or airway disorders | Pneumothorax sustained during birth or early resuscitation, congenital airway problem, congenital diaphragmatic hernia |
Metabolic conditions | Non-ketotic hyperglycinaemia, mitochondrial myopathies, methylmalonic and propionic acidaemia, maple syrup urine disease |
Extracranial trauma causing significant blood loss and/or pressure | Subgaleal, extradural and subdural haematomas |
Genetic disorders associated with thrombotic or thrombophilic abnormalities | Protein C and protein S deficiencies, factor V Leiden deficiency, anticardiolipin antibodies |
|
The term HIE is often incorrectly used synonymously with NE. The term NE is a clinical description of disordered neurological function that does not require assumptions about pathogenesis or aetiology. The diverse risk factors, causal pathways and aetiologies, which may lead to NE in a developed country such as Australia, are listed in Table 40.13 . The case-control study of NE in Western Australia showed that many antecedents of NE are present before the onset of labour. Intrapartum risk factors included maternal pyrexia, persistent occipitoposterior position and acute intrapartum events; however in over 70% of cases of NE there was no evidence of intrapartum hypoxia ( ). This is very different to the situation in many low-income countries where mothers are stunted, do not access antenatal care, have high stillbirth rates and receive poor obstetric care. Under these conditions intrapartum factors probably remain more important in the causation of NE ( ). For example, in a case-controlled study in Kathmandu, there was evidence of intrapartum hypoxia–ischaemia in 60% of encephalopathic infants. Independent risk factors for NE included short maternal stature, high maternal age, lack of antenatal care and multiple birth ( ); intrapartum risk factors included non-cephalic presentation, prolonged rupture of membranes, cord prolapse and uterine rupture.
ANTENATAL RISK FACTORS FOR NEONATAL ENCEPHALOPATHY | ADJUSTED ODDS RATIO (95% CONFIDENCE INTERVAL) ALL INCREASED RISK EXCEPT MARKED * | |
---|---|---|
Maternal | History of seizures | 2.6 (1.3–4.9) |
Older mother | 6.0 (1.3–28.2) | |
Decreased parity | 1.8 (0.9–3.7) | |
Infertility treatment | 4.4 (1.1–17.6) | |
Thyroid disease | 9.7 (2–48) | |
Bleeding | 3.6 91.3–9.9) | |
Viral illness during pregnancy | 2.9 (1.5–5.8) | |
Abnormal placenta at delivery | 2.1 (1.2–3.7) | |
Infant | Weight < 3 rd centile | 38.2 (9.4–154.8) |
Intrapartum risk factors | ||
Maternal pyrexia | 3.8 (1.4–10.1) | |
Persistent occipitoposterior position | 4.3 (1.7–10.5) | |
Acute intrapartum event | 4.4 (1.3–15.2) | |
Instrumental vaginal delivery or emergency caesarean section | 2.3 (1.2–4.7) 2.2 (1.0–4.6) | |
Elective caesarean section * |
Since 1996 there have been three consensus statements addressing the diagnosis of intrapartum asphyxia ( ; ; ). These consensus statements emphasise the use of multiple markers for the diagnosis – some signs are considered essential and others supportive. With advances in cerebral imaging technology, the two most recent statements take the findings from cerebral imaging into consideration as supportive evidence of intrapartum asphyxia ( ; ). This may cause problems, particularly in countries with poor access to this advanced technology. The diagnosis of intrapartum asphyxia has been restricted to certain types of cerebral palsy in these two most recent statements ( Table 40.14 ). As cerebral palsy is an eventual outcome, which may take several years to be confirmed, it is difficult for this to be used as an essential diagnostic criterion for an acute condition such as intrapartum asphyxia.
AMERICAN ACADEMY OF PEDIATRICS/AMERICAN COLLEGE OF OBSTETRICS AND GYNECOLOGY (1996) * | INTERNATIONAL CEREBRAL PALSY TASK FORCE (1999) † | AMERICAN COLLEGE OF OBSTETRICS AND GYNECOLOGY (2003) ‡ |
---|---|---|
|
|
|
* Committee of Fetus and Newborn (1996).
‡ American College of Obstetricians and Gynecologists Taskforce (2003).
Epidemiology
Industrialised countries
The lack of universal agreed definitions of NE and the subgroup with HIE make the estimation of the incidence and identification of risk factors problematic. Very few investigators use the same case definition for either NE or HIE. The reported incidence of NE ranges from 2 to 6/1000 live births ( ; ) and that of HIE ranges from 1 to 8/1000 live births ( ; ; ; ). This wide range reflects a mix of population-based and hospital-based incidence figures (hospital-based figures tend to be higher as such studies tend to be conducted in referral centres and therefore referral bias is introduced) as well as different time periods when the data were collected. There is evidence of a reduction in the incidence from the late 1970s (7.6 per 1000 live births) ( ) through to the late 1980s (4.6) ( ) to the early 1990s (1.9) ( ). Therefore the interpretation of older data in relation to the current situation is problematic. Not all investigators have access to population data by gestational age and the rates of HIE and NE may be quoted per 1000 births rather than 1000 term births, thus the denominator may differ between studies.
Based on population studies with similar inclusion and denominator criteria Kurinczuk et al. (2010) estimated the incidence of NE to be 3.0/1000 live births (95% confidence interval (CI) 2.7–3.3) ( ) and HIE to be 1.5 per 1000 live births (95% CI 1.3–1.7) ( ; ; ).
Role of hypoxia–ischaemia in the causation of NE in industrialised countries
Population-based studies have found intrapartum hypoxia–ischaemia to be present in a small percentage of term children with cerebral palsy, ranging from 8% in Australia ( ) to 28% in western Sweden ( ). The most recent Swedish cohort suggests that an increasing number of term infants now fulfil the American College of Obstetricians and Gynecologists’ criteria of intrapartum events severe enough to cause cerebral palsy ( ). However, a systematic review of the English-language literature on the association between intrapartum hypoxia–ischaemia and NE at term reported the proportion of cerebral palsy associated with intrapartum hypoxia–ischaemia was 14.5%, suggesting that most cases of cerebral palsy in term infants are not associated with intrapartum hypoxia–ischaemia ( ). The incidence of an umbilical arterial pH <7.0 at birth was 3.7 per 1000 births, of which 17.2% survived with neonatal neurologic morbidity, 16.3% had seizures and 24 of 6% died during the neonatal period ( ). In this meta-analysis the incidence of HIE was 2.5 of 1000 live births.
Conversely, although not population-based, a study of 351 term infants who presented within 72 hours of birth with NE or seizures or both at two tertiary referral intensive care units ( ) defined intrapartum asphyxia as the presence of at least three of the following: (1) late decelerations on fetal monitoring or meconium staining; (2) delayed onset of respiration; (3) arterial cord blood pH <7.1; (4) Apgar score <7 at 5 minutes; and (5) multiorgan damage ( ). On the basis of magnetic resonance imaging (MRI) within 2 weeks of birth or postmortem examination, 80% of the neonates with NE and asphyxia had lesions of the deep grey matter, cortex or white matter consistent with an evolving hypoxic–ischaemic insult and 69% of neonates with only seizures within 3 days of birth had acute ischaemic or haemorrhagic lesions. The authors concluded that events in the immediate perinatal period are most important in neonatal brain injury.
Mid- and low-resource settings
Infants born in the world’s least developed countries have a high risk of intrapartum-related injury and of intrapartum stillbirth ( ). Almost one-quarter of the world’s 4 million annual neonatal deaths are caused by perinatal asphyxia; 22% and 99% of these deaths occur in low- and mid-resource settings respectively ( Fig. 40.24 ). Severe infections are responsible for a further 26% of total estimated deaths ( ) and at times it is difficult to differentiate between the two conditions ( Fig. 40.25 ). The estimated that an annual 1 million survivors of ‘birth asphyxia’ may develop cerebral palsy, learning difficulties or other difficulties, although these numbers are difficult to estimate.


Precise estimates of NE and HIE incidence are uncertain as there is a lack of data from low- and mid-income countries and a complete absence of data from community-based settings where most of the burden of perinatal hypoxia–ischaemia falls. In 2006, an admission audit to the special care baby unit at Mulago Hospital, Kampala, Uganda revealed a moderate to severe NE incidence of 17.9/1000 term live births (M Nakakeeto, personal communication). In the Indian subcontinent, the Indian National Neonatal–Perinatal Database suggests an overall NE incidence of 14 per 1000 live births. As there is such a wide range in neonatal mortality rate (NMR) across countries throughout the world, a recent systematic review for the Global Burden of Disease Project estimated the incidence of NE by NMR category. In very-low-mortality settings (NMR< 5) ( Fig. 40.25 and Table 40.15 ), the median incidence of NE is 1.9 per 1000 live births (range 0.7–6.0) compared with 26.5 per 1000 live births in the highest mortality settings (based on a single study) – a 14-fold disparity. Countries with NMR 6–15, 16–30 and 31–45 have an estimated median (range) incidence of NE of 6.7 (4.7–8.7), 9.8 (3.6–10.2) and 13.4 (5.5–22.2) ( ). South Asia and Africa, with large numbers of births and deaths, account for 73% of all intrapartum-related neonatal deaths worldwide.
CATEGORY 1 VERY LOW MORTALITY NMR <5 | CATEGORY 2 LOW MORTALITY NMR 6–15 | CATEGORY 3 MODERATE MORTALITY | CATEGORY 4 HIGH MORTALITY NMR 31–45 | CATEGORY 5 VERY HIGH MORTALITY NMR >45 | |
---|---|---|---|---|---|
Incidence of NE median (range) | 1.9 (0.7–6.0) | 6.7 (4.7–8.7) | 9.8 (3.6–10.2) | 13.4 (5.5–22.2) | 26.5 (26.5) |
NE case-fatality median (range) | 21% (17–37) | 12% (12%) | 19% (10–28%) | 31% (20–33%) | No data |
Sensitising factors
Maternal pyrexia and maternal/fetal infection
Maternal intrapartum fever of >37.5°C has been shown to increase the risk of perinatal brain injury independently of infection ( ) and increase the risk of early-onset neonatal seizures at term ( ). There is also substantial experimental evidence that pre-existing intrauterine inflammation can exacerbate hypoxic–ischaemic injury ( ; ). A maternal intrapartum fever of > 38°C persisting >1 hour is usually considered a clinical indicator of chorioamnionitis; epidemiological studies suggest that chorioamnionitis is an independent risk factor for cerebral palsy among term and near-term infants ( ). Indeed, term infants exposed to maternal infection are predisposed to delivery-room depression and NE ( ). Convincing experimental ( ; ) and epidemiological evidence suggests that the ‘dual hit’ of combined infection and hypoxia–ischaemia results in more severe brain injury and increase in the risk of cerebral palsy ( ). It is likely that this dual hit may be one of the factors responsible for the worse neurological outcome even with mild or moderate NE reported from low and mid income countries ( ).
Cytokines are responsible for many normal cellular processes: the family of cytokines includes interleukins, interferons and the proinflammatory cytokines interleukin (IL)-1, 6, IL-8 and tumour necrosis factor-alpha (TNF-α). However, proinflammatory cyokines mediate a cascade of destructive cellular responses and higher levels of IL-6 and IL-8 were seen in the cerebrospinal fluid (CSF) of infants with HIE than control infants ( ). The levels of cytokines correlated with the NE stage and IL-6 correlated with outcome ( ). Other studies have shown that IL-1 correlated best with outcome ( ). Higher levels of proinflammatory cytokines were seen in neonatal blood samples of infants with cerebral palsy compared to those without ( ). Cytokines may therefore be acting as the final common pathway initiated by a variety of insults, including infection, hypoxic–ischaemic injury and reperfusion injury.
Genetic factors
Common genetic variation has been implicated as a risk modulator for perinatal brain injury: specifically, minor changes in the DNA code for a gene single-nucleotide polymorphism that alters the function or activity of the gene product are reported in genes that encode putative mediators of brain injury. These include regulators of endothelial function, inflammation and thrombosis/thrombolysis pathways ( ). One example is the single-nucleotide polymorphism in the IL-6 gene promoter region: this is an independent predictor of CP in term and near-term infants ( ). This finding suggests that the fetal inflammatory response observed in neonates with brain injury may be more than a downstream result of maternal infection and brain injury, and may instead represent an important pathogenetic factor. Neonatal studies of the IL-6-174 polymorphism suggest that the C allele is associated with either enhanced IL-6 production or adverse outcome. Term neonates with the CC genotype demonstrated increased IL-6 levels in cord blood and increased monocyte production of IL-6 in vitro, in response to lipopolysaccharide administration ( ).
In severe global brain injury in infants with NE, an association was shown with homozygosity for the 677C>T allele ( ). The prevalence of the 677C>T allele was studied in 11 children with HIE, their mothers and 85 healthy individuals. Seven mothers were homozygous and four heterozygous for the 677C>T allele. Five of the children were homozygous and six heterozygous for this polymorphism. The variant allele frequency was higher in the group of mothers with affected children than in the controls and was associated with an increase in plasma homocysteine after methionine loading. The 677C>T mutation in mothers, either in a homozygous or heterozygous state, together with poor nutritional status (probable folate deficiency) may represent a risk factor for irreversible brain injury in the offspring ( ).
Impairment of fetal growth potential
Some epidemiological studies suggest that a significant proportion of neonates with NE demonstrate signs of antepartum injury, reflected in the impairment of their growth ( ). In a case-controlled study to determine the frequency of growth impairment in NE, encephalopathic infants meeting criteria for an acute intrapartum hypoxic event (IHE, n = 21) and those who did not meet these criteria ( n = 20) were compared with controls ( ). More neonates with NE with and without IHE were below the 10th percentile of growth potential compared with controls. This association was similar in the presence or absence of IHE, which may suggest that antepartum injury has a causative rather than predisposing character in many cases of IHE.
Pathophysiology of hypoxia–ischaemia
Fetal responses to hypoxia–ischaemia
Animal models of acute, near-total hypoxia–ischaemia have demonstrated that fetal arterial P o 2 drops during acutely induced hypoxia–ischaemia from 25 mmHg to levels below 5 mmHg within 5 minutes, whereas arterial P co 2 rises from 45 to 100 mmHg within 10 minutes, and pH drops from 7.30 to 6.80 within 10 minutes ( ; ). The fetus will respond to acute hypoxia–ischaemia in a number of ways, aiming to reduce energy consumption and to maintain cerebral oxygenation as much as possible. Body movements will be reduced, which is sometimes the first sign of fetal compromise reported by pregnant women. However, this is a very non-specific finding in term fetuses. With a lowering of Pa o 2 , oxygen extraction from the blood will increase.
In the experimental animal, the response to acute fetal hypoxia includes three phases ( ). First, a redistribution of blood flow directs a greater fraction of cardiac output to the heart and central nervous system without creating metabolic acidosis. The initial rapid fall in fetal heart rate and redistribution of blood flow away from peripheral organs during hypoxia or asphyxia are key fetal adaptations generally believed to help maintain perfusion of vital organs and reduce myocardial work ( ). More profound hypoxia results in metabolic acidosis as the oxygen supply becomes inadequate to the skeletal muscles and some viscera with increasing diversion of cardiac output to the heart and brain. The final stage of fetal hypoxia corresponds to an inadequate oxygen supply to all organs, including the heart and brain. The fetus and the newborn infant both respond initially to acute hypoxia with shallow breathing followed by cessation of respirations, termed primary apnoea. After the period of primary apnoea, gasping (deep irregular respirations) develops, then respirations gradually become weaker until the onset of secondary or terminal apnoea when all respiratory effort ceases. Heart rate decreases from baseline during primary apnoea, decreases even lower during gasping and eventually ceases after several minutes of secondary apnoea. Blood pressure initially increases during primary apnoea and gasping, but then rapidly decreases during secondary apnoea ( Fig. 40.26 ) ( ). Primary and secondary apnoea is not precisely distinguishable at birth – both present as a newborn who is not breathing and whose heart rate is slow. Tactile stimulation during primary apnoea can restore spontaneous respirations. Secondary apnoea requires assisted ventilation to restore spontaneous breathing ( ).

Production of meconium can be induced by severe fetal hypoxia–ischaemia. In combination with gasping this may lead to meconium aspiration, and development of the meconium aspiration syndrome after birth ( Ch. 27 , part 2). In chronic fetal hypoxia, which may be the result of several maternal diseases such as severe anaemia, pregnancy-induced hypertension or pre-eclampsia, the fetus will produce erythropoietin to augment erythrocyte production through extramedullar haematopiesis. Increased numbers of erythroblasts have been reported to be a sign of chronic fetal hypoxia–ischaemia ( ).
Mechanisms of brain injury
Initiating role of energy failure
Severe hypoxia–ischaemia rapidly results in cessation of cerebral oxidative metabolism, accumulation of cerebral lactate and rapid depletion of adenosine triphosphate (ATP). This initial decrease in ATP is capable of triggering a series of additional mechanisms that begin with failure of the ATP-dependent Na + ,K + pump ( Fig. 40.27 ) ( ). If the initial insult is very severe, the acute result is Na + influx followed by Cl − and water influx, cell swelling, cell lysis and early death by necrosis. In the more usual less severe insult, membrane depolarisation occurs and is followed by extracellular accumulation of glutamate, increased cytosolic Ca 2+ and a cascade of events leading to a more delayed cell death, principally apoptotic, although necrosis may also occur. Cell swelling can be demonstrated in vivo within 30 minutes using diffusion-weighted MRI techniques ( ). The details of the activated cascade of events are discussed below.

The accumulation of lactate and H + is initially beneficial as it leads to the generation of ATP from phosphocreatine (PCr) (due to the shift in creatine phosphokinase reaction) and an increase in CBF (due to the effect of acidosis on vascular smooth muscle). However, as severe tissue acidosis develops, detrimental effects of increased lactate and H + develop: autoregulation is lost, phosphofructokinase activity is inhibited, leading to reduced ATP production, and acidosis itself leads to neuronal injury and necrosis ( ).
Release of excitatory amino acids
Excitotoxicity is an important mechanism of injury in the brain ( ). Glutamate is the predominant excitatory amino acid neurotransmitter in the brain, and most neurons and many glia possess receptors for glutamate ( ). Neuronal pathways mediating vision, hearing, somatosensory function, learning and memory use glutamate as their neurotransmitter ( ). There are three major groups of glutamate receptors: (1) N -methyl- d -aspartate (NMDA); (2) α-amino-3-hydroxy-5-methylisoazole-4-propionic acid (AMPA); and (3) kainic acid. Normally, glutamate is contained within the presynaptic nerve terminal until release is stimulated by neuronal depolarisation ( ); when release into the synaptic cleft does occur, the neurotransmitter is quickly taken up by high-capacity glutamate transporters in astroglia that surround synapses and nerve terminals ( ).
Extracellular glutamate concentrations increase significantly with hypoxic–ischaemic insults ( ; ) and CSF levels of glutamate of asphyxiated newborns are substantially higher than those of healthy newborn infants ( ). Extracellular glutamate increases because of two reasons: firstly, impaired uptake of glutamate; and secondly, excessive release. Compared to the adult brain, the immature brain is especially vulnerable to activation of the NMDA receptor by glutamate ( ). In addition the topography of glutamate receptors, particularly NMDA and APMA receptors, corresponds to the topography of the hypoxic–ischaemic neuronal injury ( ). Further evidence of the central role that glutamate plays in hypoxic–ischaemic injury is the neuroprotection seen with the use of glutamate receptor blockers even when administered after termination of the insult ( ). Stimulation of the NMDA receptor leads to Ca 2+ influx in to the cell and a large number of deleterious effects, described below ( Fig. 40.28 ).

Calcium influx
There are many deleterious effects of increased cytosolic Ca 2+ ( Table 40.16 ) ( ). Different enzymes are activated, including proteases and lipases. Activation of lipases causes breakdown of cellular membranes and results in production of prostaglandins and leukotrienes. During this process oxygen free radicals are generated. Activation of proteases causes activation of the caspase cascade, leading to cellular apoptosis (see below). The utilisation of ATP by ATP-dependent Ca 2+ transport systems, attempting to correct the Ca 2+ accumulation, and the Ca 2+ -mediated uncoupling of oxidative phosphorylation perpetuate the cycle. Another effect of calcium influx is activation of calcium-dependent isoforms of nitric oxide synthase (neuronal and endothelial forms).
CALCIUM ACTION | EFFECT |
---|---|
Activate phospholipases | Phospholipid hydrolysis and membrane injury Generation of free radicals by cyclooxygenase and lipoxygenase pathways |
Activate proteases | Cellular skeleton disruption Proteolysis of other proteins |
Activate nucleases | Nuclear injury |
Activate calcium ATPase and other energy-dependent calcium extrusion mechanisms | Consumption of ATP at a time of deficient ATP |
Uncouple mitochondrial oxidative phosphorylation | Decrease in ATP production |
Increase glutamate and catecholamines | Activation of glutamate receptors |
Activation of a protease that changes xanthine dehydrogenase to xanthine oxidase | Oxidation of hypoxanthine to xanthine and xanthine to uric acid with production of free radicals |
Activate nitric oxide synthase | Generation of nitric oxide and peroxynitrite with toxicity to neurons |
Reactive oxygen and nitrogen species
Free radicals are highly reactive compounds with an uneven number of electrons in the outer orbital. During hypoxia–ischaemia, but even more so during reperfusion, reactive oxygen species (ROS) or oxygen free radicals and reactive nitrogen species (nitric oxide) are produced ( ; ). These highly active chemical compounds react with cellular components and can generate new free radicals, thus producing a chain reaction, which results in irreversible injury (for example, peroxidation of fatty acids, membrane injury and cell necrosis). The body has enzyme systems, which defend the organism against injury by ROS, but these defence systems, including superoxide dismutase, may not be fully developed in the neonatal brain. Free radicals can lead to apoptotic cell death by activation of specific death genes ( ). The immature brain is also more vulnerable than adult brain to oxidative injury due to its pro-oxidant characteristics (high concentrations of polyunsaturated fatty acids and non-protein-bound iron) ( ). Production of ROS/oxygen free radicals is enhanced during resuscitation with 100% oxygen and may have long-term detrimental effects ( ). For this reason current guidelines advise clinicians to resuscitate asphyxiated full-term neonates with room air.
Inflammation
Upon cerebral hypoxia–ischaemia and reperfusion many inflammatory pathways are activated, resulting in production of cytokines, infiltration of neutrophils into the brain and activation of microglia and macrophages ( ). Activated microglia begin to accumulate in the first 4 hours after reperfusion and continue to increase over the next 48 hours ( ). Microglial cells release neurotoxic substances such as glutamate, cytokines, reactive oxygen and nitrogen species. Both IL-1β and TNF-α have been shown to increase in the brain 4–6 hours after the insult; an injection of IL-1β receptor antagonist ameliorates brain injury ( ). Antimicroglial agents such as minocycline are neuroprotective in several experimental paradigms of hypoxia–ischaemia, underlying the importance of microglia in the neurotoxic cascade ( ). As previously mentioned in the section on sensitising factors, activation of the immune response or coexisting infection (or exposure to molecular products of infection such as lipopolysaccharide) potentiates subthreshold hypoxic–ischaemic insults, leading to severe injury ( ; ; ).
Pathways of cell death
Whilst necrotic cell death is prominent in the immediate and acute phases of severe cerebral insults, the predominant mode of cell death during the delayed phase of injury appears to be apoptosis ( ). This may be particularly true with less severe insults and apoptotic cell death seems to be more important in damage to the developing brain than after adult stroke ( ). In necrosis, death is triggered by an overwhelming insult, resulting in loss of membrane integrity and leaking cytoplasmic contents into the extracellular matrix. In contrast, cells dying by apoptosis carry out a highly conserved and regulated genetic programme ( Fig. 40.29 ). They do not lose membrane integrity until late on and the organelles remain intact until the final stages when cell fragments are ‘shrink-wrapped’ in the contracting plasma membrane and bud off as apoptotic bodies, which are subsequently phagocytosed by healthy neighbouring cells. Apoptosis requires time and energy: although apoptosis and necrosis were considered distinct, there is a growing knowledge that dying cells may display a hybrid of apoptotic and necrotic features ( ; ). The presence of a ‘continuum’ phenotype of cell death that varies on a cell-by-cell basis suggests that the phenotype of cell death is dependent on the energy available to drive the apoptotic pathways to completion ( ).

Multiple apoptotic pathways have been shown to be involved in neonatal hypoxic–ischaemic cell death. As previously discussed, excitotoxicity, oxidative stress and other factors lead to injury of the mitochondrial membrane. An increase in the permeability of the mitochondrial membranes to molecules of less than 1500 Da in molecular weight (termed the mitochondrial permeability transition) plays an important role in multiple pathways to cell death ( ). Mitochondrial permeability transition leads to release of proapototic factors into the cytoplasm, including cytochrome c, apoptosis-inducing factor (AIF), caspase-9 and endonuclease ). Release of cytochrome c and procaspase-9 into the cytoplasm leads to activation of caspase-9 3–24 hours after the insult and is followed by conversion of procaspase-3 to active caspase-3 6–48 hours after injury ( ). Caspase-3 activation results in proteolysis of essential cellular proteins, including cytoskeletal proteins and kinases, and can commit the cell to the morphological changes characteristic of apoptosis, including nuclear fragmentation ( ). This cytochrome c-mediated pathway is also referred to as the intrinsic pathway. Activated caspase-3 has been shown in human postmortem brain tissue of full-term neonates with severe perinatal asphyxia.
Some cell surface receptors respond to cytokine (inflammatory) stimulation, resulting in activation of cell death signalling programmes. The TNF receptor superfamily (TNFRSF) belongs to this group of cytokine-responsive receptors. Fas death receptor is one of the most extensively studied TNFRSF members ( ). The apoptotic pathway that is regulated by Fas receptor involves caspase-8 and is referred to as the extrinsic pathway. Caspase-8 leads then to caspase-3 activation.
A caspase-independent apoptotic pathway has also been extensively studied. Poly(ADPribose) polymerase (PARP-1) is a nuclear enzyme that transfers adenosine diphosphate ribose groups from NAD+ to nuclear proteins and facilitates DNA repair. PARP activation consumes NAD+ needed for mitochondrial energy production, which in turn triggers release of cytochrome c and activation of caspases ( ).
A knowledge of these pathways of cell death has led to a very important discovery about gender-specific cell death pathways: being male or female is likely to influence the efficacy of neuroprotective agents and the cells that are most at risk. Male neurons die mainly through activation of an AIF-dependent pathway while female neurons preferentially release cytochrome c from mitochondria and die as a result of subsequent activation of caspase 3 ( ). Male neurons also have lower levels of glutathione following nitrosative stress than neurons from females. These sex-related differences in cell death pathways are present in neonatal mice and rats in vivo ( ). Gender influenced the neuroprotective efficacy of 2-iminobiotin treatment, which was neuroprotective and reduced cytochrome c release and caspase activation only in females ( ). The glutamate antagonist dextromethorphan was protective against stroke in male but not female mice at 12 days of age – this supports the greater influence of the excitotoxic pathway in immature males ( ).
This new information about gender differences in neuronal death pathways in experimental models is clinically relevant to perinatal asphyxia. The 2005 Surveillance of Cerebral Palsy in Europe study reported that male babies are at higher risk for cerebral palsy than females. In low-birthweight infants cognitive and motor outcome of brain injury is worse in males than in females ( ). In future studies it will be important to determine the influence of gender in asphyxiated infants undergoing neuroprotective therapies and optimisation of therapy may require different agents for males and females.
Cerebral blood flow and autoregulation
In healthy neonates CBF is maintained within certain limits by autoregulation when arterial P co 2 or blood pressure fluctuates. Studies using xenon clearance or Doppler ultrasound have demonstrated that autoregulation was impaired in full-term neonates with perinatal asphyxia, making cerebral perfusion pressure passive ( ; ; ). Infants with the poorest outcomes (isoelectric amplitude-integrated electroencephalogram (aEEG), death) had the highest values for CBF and no autoregulation or CO 2 reactivity. Infants with burst suppression and moderate to severe brain injury had slightly elevated values for CBF and impaired autoregulation but sustained reactivity to CO 2 . Infants without evidence of brain injury had normal values for CBF, intact autoregulation and reactivity to CO 2 . This cerebral hyperaemia occurring in infants with perinatal asphyxia has been confirmed with Doppler studies and near-infrared spectroscopy studies. These studies showed an increase in CBF and decreased resistance between 6 and 130 hours. Other near-infrared spectroscopy studies have confirmed this loss of vascular reactivity, increase in cerebral blood volume and CBF ( ) as seen before in the fetal sheep ( ). The postasphyxial newborn is in a state of vasoparesis and cerebral hyperaemia which correlates with the degree of brain injury. This state of maximal vasodilation may be related to the effects of elevated H + , prostaglandins, adenosine, free radicals or nitric oxide. It is unknown, however, whether it is an adaptive mechanism to preserve brain injury or whether it causes additional injury.
Pathology
Animal models of term hypoxia–ischaemia
The use of animal models in the study of perinatal asphyxia has a long history. ( ) noted that, in newborn rabbits asphyxiated by submersion, respiration survived for 27 minutes, whereas in adult rabbits breathing ceased after 2 minutes. In 1870, Bert saw a similar resistance to asphyxia in the newborn rat submerged in water – respiratory movements lasted 30 minutes whereas in 20-day animals respirations ceased in 1.5 minutes ( ). Animal studies have shown that the premature animal is more tolerant of asphyxia than the term infant, which is in turn more resistant to asphyxia than the adult. These early studies have also shown that males appear to be less resistant than females and that high temperature, thyroxine, insulin or adrenalectomy reduces resistance to asphyxia.
The primate neonatal model shows very clearly that the pattern of brain injury is affected by the severity and type of hypoxia–ischaemia. Two distinct patterns of injury were described in the 1950s–1970s – acute total asphyxia ( ) and chronic partial asphyxia ( ). Acute total asphyxia was induced in monkeys near term by detaching the placenta at hysterotomy under local anaesthesia, keeping the fetal membranes intact. Eleven to 16 minutes later the fetuses were delivered from their membranes and resuscitated. Two to 9 days later the monkeys were euthanised: there was a common pattern of symmetrical injury to the ventral posterior nuclei of the thalamus, putamen, globus pallidus, inferior colliculus, gracile and medial cuneate nuclei – a very similar topographical distribution to that seen in infants with basal ganglia and thalamus (BGT) injury following, for example, a sentinel event. The cerebral cortex was damaged in only one out of five monkeys and there was no relationship with cerebral vascular distribution. The injury began with primary nerve cells with secondary damage of myelin sheaths and astrocytic reaction.
In the mid-1970s, established a different model of chronic partial asphyxia in the rhesus monkey to investigate the effect on the newborn monkey brain. Intrauterine asphyxia was produced in the pregnant term monkey by breathing halothane of a high enough concentration to cause a significant drop in mean blood pressure in the mother. Each fetus was exposed to asphyxia for 1–5 hours. At the end of the period of asphyxia, each fetus was delivered surgically, intubated and ventilated with 100% oxygen. Monkeys were given sodium bicarbonate and dextrose for correction of the acidosis and taken off the ventilator when able to breathe on their own. Five of the eight asphyxiated newborn monkeys exhibited low Apgar scores and delayed seizures starting from 14.5 hours after delivery. Following an average of 46 hours of survival the brains were perfusion-fixed and the pattern of brain injury assessed. A mainly symmetrical pattern of parasagittal injury was seen, involving the convexity of the hemispheres, particularly the paracentral and posterior parieto-occipital areas ( Fig. 40.30a ). In some newborn monkeys basal ganglia damage was present; however injury to brainstem structures was absent. In around half the animals there was an asymmetry and in these cases the left side was always more affected. A primate model of perinatal asphyxia is still used for neuroprotection studies ( ). These findings are very similar to the pattern of injury in newborn infants who have experienced chronic partial asphyxia ( Figs 40.30b and c and 40.31 ).


Patterns of injury
Using MRI, the patterns of hypoxic–ischaemic brain injury that have been described in pathology studies in the past can now be demonstrated with superb detail and resolution in vivo in human neonates ( ).
Basal ganglia/thalamus pattern
The BGT pattern of injury is the most common pattern seen in infants with HIE ( ) when there has been a sentinel event such as a ruptured uterus or placental abruption during labour ( ) involving ‘acute near-total asphyxia’. This pattern is associated with more severe neonatal signs, more intensive resuscitation at birth, more severe encephalopathy and more severe seizures ( ). These deep nuclear structures are very susceptible to acute perinatal hypoxic–ischaemic injury because of their high metabolic rate and high concentration of NMDA receptors. BGT injury predominantly affects bilaterally the central grey nuclei (ventrolateral thalami and posterior putamina) and perirolandic cortex (especially around the central sulcus, interhemispheric fissure and insula) ( Fig. 40.32 ). Brainstem injury often accompanies the BGT pattern of injury. Lesions are often seen in the midbrain (the inferior colliculus) and pons and the cerebellar vermis ( ). This finding is in keeping with the studies of and ( ) of asphyxiated monkeys with total asphyxia, and the primate studies of (see above).

BGT lesions vary in extent and severity ( Fig. 40.33 ). Mild lesions are focal lesions in the ventrolateral nuclei of the thalamus and posterior putamen. Moderate lesions are defined as multifocal areas or more diffuse abnormalities on T2, involving several regions of the basal ganglia. In severe injury there is widespread abnormal signal intensity – this may involve the whole of the BGT area but often the caudate is spared. White-matter injury may occur with severe BGT injury as part of the original insult or occur secondarily to the BGT lesions and is seen later in infancy and associated with poor head growth ( ).




The posterior limb of the internal capsule (PLIC) is actively myelinating at term-equivalent age and is also very susceptible to injury at this stage of development ( ). Damage to the PLIC usually correlates with the severity of the BGT lesions. On MRI it can be described as normal, equivocal and abnormal. A normal PLIC is myelinating at term age and on MRI is seen as high signal on T1-weighted images and low signal but more ovoid shape on T2-weighted images ( Fig. 40.33a ). An abnormal PLIC has loss or reversed signal intensity on T1- and T2-weighted images ( Fig. 40.33c,d ); an equivocal PLIC is one with reduced or asymmetrical signal intensity ( Fig. 40.33b ).
Outcome
A total of 20–30% of infants with HIE die in the neonatal period and 15–20% of children may die in the first 2–3 years. have observed that the best predictor of death is the presence of brainstem lesions. They found that 50% of infants with brainstem lesions died in the neonatal or infant period.
Survivors face a range of functional impairments which include cerebral palsy, feeding problems, speech and language problems, visual and hearing impairment, later seizures and cognitive impairment. Cerebral palsy affects three-quarters of infants with HIE with BGT lesions and the severity of the BGT lesions is the best predictor of motor problems. The signal intensity of the PLIC is the best predictor of the ability to walk at 2 years ( ). From their extensive experience in following infants with HIE, Martinez-Biarge et al. have produced very comprehensive flow charts for likely outcome for mild, moderate or severe BGT in surviving infants ( Fig. 40.34a–c ).



Watershed-predominant pattern
There are several terms for this pattern of injury – parasagittal cerebral injury or border zone injury. This injury pattern is thought to result from chronic partial hypoxia – in the primate model this injury was produced by several hours of maternal hypotension (see the section on animal models, above). The watershed pattern is also encountered after hypotension, infection and hypoglycaemia, all of which may be associated with a more chronic course and may be preceded by antenatal hypoxia. In one cohort representative of the spectrum of severity of term NE, the watershed-predominant injury pattern was the most common injury pattern, seen in 45% of the cohort ( ); however a mixed pattern is often seen and watershed injury is often accompanied by less severe BGT injury.
The injury comprises cortical necrosis involving the immediately subjacent white matter with characteristic distribution, encompassing the parasagittal, superomedial areas of the convexities bilaterally, with posterior (parieto-occipital) more involved than anterior regions. The areas of necrosis in watershed injury are in the border zones between the major cerebral arteries. These border zones are the areas most susceptible to ischaemia from a fall in perfusion pressure ( Fig. 40.30a–c ). Another very vulnerable area for ischaemic cerebral injury is at the depth of the sulci. showed that, as sulci form and deepen near term in the human brain, the meningeal vessels are forced to bend acutely at the cortical–white-matter junction. This area represents ‘a border zone within a border zone’. Therefore cerebral injury is more severe in the depths of the sulci; over time the cortex of the affected gyri shrinks and the term ‘ulegyria’ is used to define the type of cortical abnormality characterised by atrophy at the depth of the sulci and relative sparing of the crest of the gyri ( ). The term ‘ulegyria’ was introduced from the Greek word oυλη′ (scar) and its presence suggests that the injury occurred around term age. With further development, additional vessels appear to supply this area.
Outcome
BGT and watershed injuries are associated with impairments in different developmental domains. Infants with a watershed pattern of injury have predominantly cognitive impairments, often without functional motor deficits. Cognitive deficits include memory impairments, visual–motor or visual–perceptive dysfunction, or increased hyperactivity, sometimes in the absence of functional motor problems ( ; ; ; ). These cognitive deficits often result in delayed school-readiness and a need for additional school-age interventions ( ). Cognitive deficits may not be detected at 12 months – therefore school-age assessment is needed, as are ongoing formal neuropsychological evaluation, as well as parental and teacher education, to help aid in the cognitive and behavioural rehabilitation resulting from perinatal hypoxic–ischaemic brain injury.
Global pattern
Involvement of the subcortical white matter and cortex can be seen, referred to as the ‘white cerebrum’, as diffusion-weighted imaging (DWI) shows an almost completely white cerebrum, in contrast with a normal-looking cerebellum. These patients show severe NE and the condition tends to be fatal. In the rare surviving neonate, multicystic encephalomalacia will develop ( Fig. 40.35 ). Another important cause of multicystic encephalomalacia is central nervous system infection, especially with herpesvirus.

Cerebral energy metabolism associated with hypoxia–ischaemia
Magnetic resonance spectroscopy (MRS) has been used to study brain energy metabolism non-invasively; over the last 30 years phosphorus-31 ( 31 P) and proton ( 1 H) MRS have provided unique information on cerebral energy metabolism during the evolution of brain injury following hypoxia–ischaemia in the newborn infant ( ), neonatal rat ( ) and newborn piglet ( ; ). In human infants, shortly after intrapartum hypoxia–ischaemia 31 P MRS often reveals apparently normal cerebral energetics ( ). However, in infants with adverse outcome, despite adequate oxygenation and circulation, PCr and nucleotide triphosphate (NTP, mainly ATP) decline, and Pi increases, in the first days of life ( ; ; ; ). These metabolic changes were termed ‘secondary energy failure’ (SEF) on the basis that they followed impaired intrapartum cerebral energy generation (resulting in transiently reduced PCr and NTP and increased Pi), which resolved following resuscitation ( ). It was assumed that SEF was consequential to a pathological mechanism initiated by intrapartum hypoxia–ischaemia and/or reperfusion/reoxygenation. 1 H MRS provides complementary information to 31 P MRS (in particular cerebral lactate, a marker of anaerobic metabolism and N -acetyl aspartate (NAA), an abundant amino acid found mostly in neurons in the central nervous system ( )) and because of the greater sensitivity of the 1 H nucleus, data can be obtained from smaller regions of the brain. NAA appears as a singlet peak in the 1 H MR spectrum and is easier to quantify than lactate ( Fig. 40.33a–d ). The roles of NAA include action as: (1) an osmolyte ( ); (2) a supplier of acetate for myelin sheath synthesis ( ); (3) a facilitator of mitochondrial energy generation ( ); and (4) a ligand for some metabotropic glutamate receptors ( ). Cerebral lactate increases and NAA decreases during transient hypoxia–ischaemia; these metabolites return almost to baseline levels after successful resuscitation, only to be followed by a secondary increase in lactate and slower reduction in NAA in the hours that follow. These 1 H MRS changes occur in parallel with the energy disruption (reduction in PCr/exchangable phosphate pool (EPP) and NTP/EPP) seen on 31 P MRS ( Fig. 40.36 ).

We have used the composite biomarker consisting of the ratio of the cerebral lactate (Lac) and NAA peak areas at TE 288 ms (Lac/NAA) in our MRS studies of NE. The reciprocal changes in Lac and NAA concentrations increase the sensitivity to detect even minor neural injury, as well as enabling assessment of more severe injury. A recent meta-analysis of the prognostic accuracy of MR methods demonstrated that thalamic 1 H MRS Lac/NAA peak area ratio acquired between days 5 and 14 is a highly sensitive and specific biomarker of long-term neurodevelopmental outcome in infants with NE ( ). Lac/NAA was more predictive than any other MRI technique including early and late conventional MRI, presence or absence of the normal signal from the PLIC and apparent diffusion coefficient (ADC) imaging.
Biphasic impairment of cerebral energy metabolism with transient hypoxia–ischaemia has been demonstrated in the newborn piglet ( ; ; ); these studies provided a basis for the realisation that rescue treatment after hypoxia–ischaemia may reverse or ameliorate SEF. Experimental studies with moderate hypothermia ( ) and other potential therapies soon followed ( ). Recent work in the piglet model has shown that hypothermia extends the therapeutic window for other pharmacological therapies and the more severe the hypoxia–ischaemia, the shorter the therapeutic window ( ).
Biomarkers of brain injury
A biomarker is a characteristic that is objectively measured and evaluated as an indicator of normal biologic processes, pathogenic processes or pharmacologic responses to a therapeutic intervention. Biomarkers are needed in infants with HIE to predict which infants are most at risk of an abnormal outcome and who would benefit most from neuroprotective intervention (early biomarkers) and to predict long-term outcome following neuroprotective interventions (late biomarkers) ( ).
Markers of exposure to hypoxia–ischaemia
Markers of exposure to hypoxia–ischaemia are the obvious biomarkers to predict outcome; however many of these measures have low predictive values for neural injury. For example, the presence of non-reassuring fetal heart changes, increased base deficit and blood lactate values on cord blood gases and need for resuscitation (i.e. Apgar score) infer exposure to hypoxia–ischaemia and are routinely recorded. However, non-reassuring heart rate changes are well known to have a very low positive predictive value for neural injury ( ). Base deficit ( ) and fetal lactate concentrations show an imprecise relationship with NE ( ). Early neonatal lactate values in blood are predictive of encephalopathy stage ( ; ).
Biochemical biomarkers
Currently, many of the biochemical biomarkers can distinguish between mild and severe injury, but are not able to predict outcome accurately in infants with moderate or stage II encephalopathy. In a recent meta-analysis serum IL-1β and IL-6, and CSF IL-1β and NSE measured before 96 hours of age were predictive of long-term outcome ( ). IL-6 ( ), S100 beta ( ), Activin A ( ), adrenomedullin and protein carbonyls are raised in the umbilical cord samples of hypoxic infants compared to controls. However so far only IL-6 and S100 beta have shown any ability to distinguish between grade of HIE ( ). showed that umbilical arterial S100 beta, at a cut-off of 2.02 µg/L, had a sensitivity of 86.7% and a specificity of 88.0% for predicting moderate or severe HIE, although an alternative grading system to the Sarnat system was used in this study. In another study a cut-off >1.0 µg/L urinary protein S100 beta had a sensitivity/specificity of 100% for predicting neonatal death ( ). Nucleated red blood cells have been shown to be higher in encephalopathic infants with abnormal cerebral Doppler ultrasound, abnormal cranial ultrasound (cUS) and outcome at 2 and 3 years ( ). A urinary lactate-to-creatinine ratio has been associated with adverse outcome in infants with HIE ( ; ), but the significant overlap between normal/mild and moderate/severe disability groups limits the predictive value of this measure. Further research into the time course of changes in relation to the evolution of brain injury as well as into new methodologies such as bioinformatics and metabolomics ( ) is needed before we can be sure that any of these measures can improve early outcome prediction.
Imaging biomarkers
In clinical practice, MRI is increasingly used after perinatal asphyxia as an effective tool for diagnosis and treatment efficacy assessment ( ). To increase prognostic objectivity, quantitative cerebral biomarker modalities such as 1 H MRS have also been used ( ; ; ). As described above, a recent meta-analysis demonstrated that the cerebral 1 H-MRS Lac/NAA peak area ratio acquired 5–14 days after birth is the most sensitive and specific biomarker of long-term neurodevelopmental outcome in NE ( ); this surrogate outcome measure is currently being used in a phase II clinical trial of hypothermia versus hypothermia plus inhaled xenon with the aim of speeding up clinical translation of effective therapies. The search for robust, sensitive and specific biomarkers is an important area for future research.
Clinical aspects
Fetal compromise
The intrapartum period is a time of risk for asphyxia; intrapartum care aims to identify those babies who are experiencing hypoxia–ischaemia. The fetus is generally well adapted to cope with the physiological stress normally imposed by labour, but on occasion the ‘reserve capacity’ is overwhelmed. The aim of intrapartum monitoring is to detect this.
Intrapartum fetal monitoring
When intermittent auscultation is used for low-risk pregnancies, twice as many babies will require admission to the neonatal intensive care unit (NICU) with NE than if electronic monitoring is used; however intermittent auscultation is supported by a Cochrane review ( ). Continuous cardiotocography during labour was associated with a reduction in neonatal seizures, but no significant differences in cerebral palsy, infant mortality or other standard measures of neonatal well-being ( ).
Some studies suggest electronic fetal monitoring correlates well with metabolic acidosis and neurological injury. The best intrapartum fetal heart rate parameter to predict development of acidaemia was minimal/absent variability for at least 1 hour (a single abnormal finding) or accompanied by late decelerations in the absence of accelerations ( ). However, although the sensitivity of these electronic fetal monitoring abnormalities for identifying intrapartum asphyxia was 93%, the positive predictive value was only 3–18%. Neonates who developed seizures had a significantly longer duration of abnormal fetal heart rate patterns (72 ± 12 versus 48 ± 12 minutes, P < 0.001) ( ). In fetal monkeys, during the course of fetal hypoxia severe enough to cause death, fetal heart rate variability decreased, then disappeared; late decelerations preceded fetal acidaemia. Other studies have not found electronic fetal monitoring to be clinically helpful in the identification of fetal acidosis or neurological injury. Vigilance is a very important part of intrapartum fetal monitoring ( ).
Caesarean section
Studies have shown an inverse relationship between elective caesarean section and NE ( ). This is, however, a contentious issue – the risks of high rates of caesarean section to both mother and baby are considerable.
Staff training, guidelines and clinical governance
Several investigations suggest that intrapartum asphyxia is preventable ( ). Suboptimal intrapartum care has been shown to occur in 40–50% of cases with metabolic acidosis at birth ( ). It has been shown that intrapartum care protocols can decrease caesarean section rates and result in fewer adverse outcomes ( ). In the UK, the Royal College of Obstetricians and Gynaecologists recommends that all obstetric services have robust and transparent clinical governance frameworks in place, with multidisciplinary care and good communication being key factors for good practice.
Clinical manifestations of hypoxic–ischaemic encephalopathy
Clinical features
Apgar scores
The original intent of the Apgar score was to provide a description of the newborn’s physical condition and enable comparison of obstetric practice, maternal analgesia and resuscitation ( ). A hypoxic–ischaemic insult may cause depression of the Apgar score, but this is not invariable and many other non-asphyxial factors can cause depression of the Apgar score, e.g. maternal analgesia, infection, prematurity. Nevertheless prolonged depression of the Apgar score is related to death or severe neurodevelopmental outcome ( ).
Neurological syndrome and encephalopathy scores
NE is an evolving clinical illness typically with worsening of clinical signs (seizures and impaired conscious state) after the first 4–16 hours and a slow improvement after about 4–5 days. introduced a grading system to describe the neurological abnormalities, which has been modified by . An updated version has been used by the National Institute of Child Health and Human Development (NICHD) Neonatal Research Network to identify newborn infants who may be eligible for neuroprotection ( ) ( Table 40.17 ). Several other scoring systems now exist, all of which are based on that of Sarnat and Sarnat; recent modifications have been directed at developing quantifiable scores with good reproducibility ( ; ; ) ( Table 40.18 ).
SEVERITY OF ENCEPHALOPATHY | |||
---|---|---|---|
Mild | Moderate | Severe | |
Level of consciousness | Alert or hyperalert | Lethargy | Stupor or coma |
Spontaneous activity | May be normal | Decreased activity | No activity |
Posture | May be normal | Distal flexion, complete extension | Decerebrate |
Tone | Normal or hypertonia | Hypotonia (focal or generalised) | Flaccidity |
Primitive reflexes | |||
Suck | Weak | Weak | Absent |
Moro | Exaggerated | Incomplete | Absent |
Autonomic function | |||
Pupils | Dilated | Constricted | Deviated, dilated or non-reactive to light |
Heart rate | Tachycardia | Bradycardia | Variable |
Respiration | Normal | Periodic breathing | Apnoea |
Others | Irritability, jitteriness | Brainstem dysfunction | ± elevated intracranial pressure |
Seizures | Absent | ± | Frequent, often refractory to anticonvulsants |
EEG background | Normal | Low-voltage, periodic or paroxysmal | Periodic or isoelectric |
Outcome | Normal | 20–40% abnormal | Death or 100% abnormal |
SCORE | ||||
---|---|---|---|---|
Sign | 0 | 1 | 2 | 3 |
Tone | Normal | Hypertonic | Hypotonic | Flaccid |
conscious state | Normal | Hyperalert, stare | Lethargic | Comatose |
Fits | Normal | Infrequent, <3/day | Frequent, >2/day | |
Posture | Normal | Fisting, cycling | Strong distal flexion | Decerebrate |
Moro | Normal | Partial | Absent | |
Grasp | Normal | Poor | Absent | |
Suck | Normal | Poor | Absent/bites | |
Respiration | Normal | Hyperventilation | Brief apnoea | Apnoeic |
Fontanelle | Normal | Full, not tense | Tense |
Stage 1 encephalopathy (mild)
Infants with the mildest degree of encephalopathy have transient irritability, hypertonia and poor feeding. Muscle tone is normal or increased and the deep tendon reflexes are hyperactive. The Moro reflex is often exaggerated. Pupillary dilatation and tachycardia are frequently seen. Mild encephalopathy usually lasts less than 24–48 hours and is associated with a good neurological outcome, although there are concerns about learning and memory difficulties in later childhood even in infants who appear to do well ( ).
Stage 2 encephalopathy (moderate)
Newborn infants with moderate encephalopathy (stage 2) show lethargy, hypotonia, hyporeflexia and seizures. The EEG typically shows reduced background activity associated with a lowering of the lower baseline on the amplitude integrated EEG with seizures. Infants can typically be aroused with auditory stimuli or touch. Feeding is poor. Muscle tone is decreased with a prominent head lag. Exaggerated deep tendon reflexes are seen and clonus may be elicited. Cranial nerve examination reveals a weak suck and decreased gag reflex. Spontaneous movement is decreased. Pupils are often constricted and periodic breathing and bradycarida may occur. Seizures typically occur. Stage 2 encephalopathy is associated with a poor outcome in approximately 15–40% of the infants ( ), especially if abnormal neurologic signs persist for over 1 week.
Stage 3 encephalopathy (severe)
The most severely affected infants have profound stupor or coma and the EEG is usually isoelectric, suppressed or shows burst suppression. Infants are flaccid and unresponsive to any stimuli. They also have episodic decerebrate (extensor) posturing and poor brainstem function. Deep tendon reflexes and sucking are absent. Seizures are common and may be difficult to treat. The infant often has bradycardia, hypotension, irregular respirations and apnoea. The mortality rate is high and nearly all survivors develop sequelae.
In many infants clinical features are intermediate between these stages or there is a transition from one stage to another over the first 1–3 days.
Multiorgan dysfunction
During hypoxia–ischaemia, the diving reflex is activated. This reflex shunts blood from the skin and splanchnic area to the heart, adrenals, and brain, ostensibly to protect these vital organs from hypoxic–ischaemic injury. It has been suggested that neonates with brain injury secondary to hypoxia–ischaemia would have activated the diving reflex for long enough to cause dysfunction of one or more nonessential organs, particularly kidney and liver. Multiorgan dysfunction has been considered a constant feature of the neonatal postasphyxial syndrome. However, in a retrospective cohort study of infants with HIE, there was no association between multiorgan dysfunction and outcome or between individual or combinations of organ involvements and outcome ( ). In this study specific renal, cardiac, pulmonary and hepatic criteria for multiorgan dysfunction ( Table 40.19 ) were used. All infants had evidence of multiorgan dysfunction (at least one organ dysfunction in addition to HIE). Renal, cardiovascular, pulmonary, and hepatic dysfunction was present in 58–88% of infants with good outcome and 64–86% of infants with adverse outcome.
SYSTEM/ORGAN | CRITERIA |
---|---|
Renal | Anuria or oliguria (< 1 ml/kg/h) for 24 hours or more, and a serum creatinine concentration >100 mmol/l; or anuria/oliguria for >36 hours; or any serum creatinine >125 mmol/l; or serial serum creatinine values that increased postnatally |
Cardiovascular | Hypotension treated with an inotrope for more than 24 hours to maintain blood pressure within the normal range, or electrocardiographic evidence of transient myocardial ischaemia |
Pulmonary | Need for ventilator support with oxygen requirement >40% for at least the first 4 hours after birth |
Hepatic | Aspartate aminotransferase >100 IU/l or alanine aminotransferase >100 IU/l at any time during the first week after birth |
Management of hypoxic–ischaemic encephalopathy
Resuscitation ( Ch. 13.1 )
In the UK, 10% of newborns (approximately 70 000 annually) require some form of resuscitaton after birth. Most infants respond quickly and make a full recovery, although it is concerning that infants who do not develop encephalopathy may have an increased risk of a low IQ score at 8 years if they required resuscitative efforts at birth, and infants with just brief depression of the Apgar score (<7 for 1 min after birth) may have poorer function in cognitive tests in later life ( ).
A systematic review of the literature of surviving infants with Apgar zero at 10 minutes and who were resuscitated successfully, show that the outcomes for infants who do not respond to cardiopulmonary resuscitation by 10 minutes of age ( ). These findings present strong evidence that adequate resuscitation beyond 10 minutes is not justified and form the basis for the ILCOR recommendation to discontinue resuscitation after 10 minutes of adequate resuscitation ( ). However, in a recent analysis of infants who qualified for the NICHD cooling trial, the outcome of survivors with an Apgar score of 0 at 10 minutes was more heterogeneous; 6 of the 13 survivors had either a mild or an absent disability at an 18–22-month assessment ( ).
Our practice is based on the 2006 International Liaison Committee on Resuscitation (ILCOR) guidelines, although it is important that experienced staff attend if the infant does not respond promptly to standard resuscitative measures. Complications such as a tension pneumothorax may be difficult to ascertain in the full-term infant in the delivery suite and some infants may have coexisting abnormalities that may be undetected by inexperienced personnel. Resuscitation in room air is advised – the use of 100% oxygen at birth is associated with worse outcomes than the use of room air, possibly because of free-radical injury and pulmonary atelectasis ( ).
Clinical management in the NICU
Best-practice guidelines are available ( ). The initial management of infants with HIE following admission to the neonatal unit consists of standard neonatal intensive care measures, continuous core temperature monitoring using a rectal or oesophageal probe, initiation of therapeutic hypothermia if appropriate (see section on therapeutic hypothermia , below) and neurological monitoring by regular clinical examination, continuous EEG or aEEG and regular cUS examinations. Clinical management will depend on the severity of NE.
Ventilation and oxygenation
Inadequate ventilation and persistence of hypoxia can further aggravate cerebral injury and should be treated promptly. On the other hand, in many postasphyxial infants, cardiorespiratory function improves rapidly, outstripping weaning and resulting in unintentional hyperoxaemia and/or hypocapnia. Severe hyperoxaemia and severe hypocapnia have been associated with adverse outcome in infants with postasphyxial HIE, therefore during the first hours of life, oxygen supplementation and ventilation must be rigorously controlled ( ). In a study adjusting for the severity of birth asphyxia, peak Pa o 2 values exceeding 26.6 kPa (200 mmHg) and trough Pa co 2 values of 2.6 kPa (20 mmHg) or lower during the first 20–120 minutes of life were associated with death or adverse neurodevelopmental outcome. The risk of adverse outcome was greatest in subjects who had both. Hypocapnia, by decreasing CBF, may increase the risk of injury in ischaemic cerebral disease states ( ); mechanisms of oxygen-induced brain damage are well established ( ).
Cardiac dysfunction
Cardiovascular injury from hypoxia–ischaemia may be manifested as decreased ventricular function, abnormalities of rate and rhythm, tricuspid regurgitation and hypotension. Adequate perfusion of the brain is essential to prevent additional cerebral damage and inotropes and volume expanders may be necessary, especially if there is a transient hypoxia-induced myocardiopathy.
Metabolism
Normoglycaemia should be maintained, and adequate protein and caloric intake should be provided as soon as possible. Excessive early enteral feeding may facilitate the occurrence of necrotising enterocolitis, because the gut may be injured by hypoxic–ischaemic damage. Hypoglycaemia may aggravate the damage from hypoxic–ischaemic injury ( ).
Coagulopathy and other organ damage
Birth asphyxia-related disseminated intravascular coagulation reduces levels of coagulation factors and thrombocytes, which can result in prolonged bleeding times. Renal dysfunction due to acute tubular necrosis is common, and is nearly always a temporary problem that resolves over the first week of life. Renal problems range from mild oliguria, proteinuria and haematuria to renal tubular acidosis and renal failure. However, permanent renal damage associated with HIE is very rare. Liver damage may exacerbate coagulation problems and may lead to elevated liver enzymes. Metabolic abnormalities are common and include hypoglycaemia, hypocalcaemia, hypomagnesaemia and sodium and potassium abnormalities.
Treatment of seizures
Seizures enhance the metabolic demand of the neonatal brain and independently exacerbate hypoxic–ischaemic injury ( ). Therefore, early recognition of seizure activity is important. Phenobarbital is the most commonly used first-line anticonvulsant ( ). In many units in Europe midazolam or lidocaine is used as add-on therapy. Elsewhere in the world phenytoin, lorazepam and primidone have been used. Future anticonvulsants for neonates may be topiramate, a blocker of the AMPA-type glutamate receptor, bumetanide, a blocker of NKCC1, a Cl − cotransporter or levetiracetam. In most cases therapy can be limited to the first week after hypoxia–ischaemia. In neonates with severe brain injury maintenance of anticonvulsants may be indicated, but this is uncommon.
Documentation
It is important to obtain a detailed structured clinical history to identify risk factors for HIE, and evidence of alternative diagnoses and comorbidities. This information needs to be carefully documented so that it is readily available to multidisciplinary teams that are often involved in the long-term clinical management of affected children. The detail needed in the documentation is shown in Table 40.20 ( ).
DOCUMENTATION | DETAILS |
---|---|
Maternal and family history | Maternal and paternal age and health, consanguinity, ethnic background, parity, details of previous pregnancies, health of other children, family history of thrombosis, thyroid disease, active herpes infection, neurological disease, cerebral palsy, seizures, neonatal or infant deaths, travel abroad before or during pregnancy |
Details of current pregnancy | Last menstrual period and expected date of delivery and certainty, conception, whether natural or assisted, history of infertility, previous fetal losses and stillbirths, maternal medication/drugs, antenatal care, results of chorionic villous sampling/amniocentesis, results of antenatal scans, placental site, fetal position, fetal movements, including time of onset and any changes, chorionicity of twins, history of twin-to-twin transfusion syndrome, presence of oligo/polyhydramnios, growth pattern, infection, essential hypertension/pre-eclampsia, diabetes mellitus/gestational diabetes, accidental injury, rhesus incompatibility, results of any cardiotocogram in pregnancy |
Details of resuscitation | Evidence for intrapartum fetal distress, cord blood gases and lactate from both arterial and venous samples, Apgar scores at 1, 5 and 10 minutes, need for intubation and duration, difficulties in intubation or ventilation, presence of meconium below the cord, onset of satisfactory heart rate, time to first gasp, onset of regular respirations, maximum ventilatory requirement, any drugs/fluids administered |
Care in the NNU | Remember to inform registry, e.g. TOBY UK cooling register (www. npeu.ox.ac.uk/tobyregister) |
Neurological monitoring
Regular neurological assessment is necessary to determine the severity of NE, detect deterioration and complications such as cerebral sinus thrombosis or haemorrhage, to assess the response to therapies such as anticonvulsant therapy and to assess likely neurological outcome to plan further clinical management. Details of neurological monitoring are given in Table 40.21 ( ).
NEUROLOGICAL MONITORING | ACTION |
---|---|
Daily neurological examinations | Document clinical encephalopathy score as HIE evolves over 1–4 days |
Continuous aEEG or EEG | For a minimum of 48 hours |
Monitor for clinical seizures | Correlate subtle signs such as lip smacking, head turning, eye turning, bradycardias and apnoeas with the aEEG. Subclinical seizures may be apparent on aEEG |
Treat clinical (and subclinical) seizures | Phenobarbital 20 mg/kg first-line, repeated if necessary. |
Daily cranial ultrasound | For first 4 days. Measure cerebral flow velocities and calculate pulsatility index during first 3 days |
MRI/ 1 H MRS | If only one scan can be performed, the optimal timing is 5–14 days |
Investigations
When encephalopathy has developed following resuscitative efforts at birth with evidence of perinatal asphyxia and following a sentinel event, a diagnosis of HIE is likely to be correct. Nevertheless, coexisting or predisposing conditions such as infection or trauma or metabolic disorder also need to be considered. A protocol will help ensure the most appropriate investigations are carried out and important ones not left out. Suggested first-, second- and third-line investigations are described in recent reviews on NE and summarised in Table 40.22 ( ).
INVESTIGATIONS | |
---|---|
First-line * |
|
Second-line | Investigation for metabolic or genetic disorders if any dysmorphic features, parental consanguinity, abnormal intracranial anatomy, severe growth restriction, unusual pattern of injury on MRI scan, normal-looking MRI scan with ongoing neurological problems |
Third-line |
|
Cranial ultrasound
cUS has been used extensively in neonatal practice, since it is a convenient technique to visualise the neonatal brain through the anterior fontanelle serially without moving or disturbing the patient in a neonatal intensive care setting.
cUS scanning is helpful to exclude structural abnormality suggesting metabolic and other diagnoses, detect calcification and cysts suggestive of viral infection and detect atrophy suggestive of long-standing damage. It will also identify cerebral haemorrhage. Sequential observation of the evolution of injury following a recent hypoxic–ischaemic insult at birth is helpful both for defining the pattern of lesion and timing its onset. Brain swelling is a non-specific finding on cUS, which supports the diagnosis of hypoxia–ischaemia, but is not always present. The presence of ‘slit-like’ ventricles on the first day of life, however, is a very non-specific finding, since many healthy neonates have small ventricles. The ultrasound appearances of cerebral oedema include loss of the normal anatomical detail with obscuration of the sulcal markings and closure of the interhemispheric and sylvian fissures. The brain has a sparkly appearance with increased echoreflectance.
Following acute and severe hypoxia–ischaemia, echogenicity of the BGT may be seen to appear 24–48 hours after birth. The thalami appear egg-shaped and echoreflectant. In the coronal view the internal capsule remains of normal echogenicity and gives rise to a ‘stripe’ as it sits between the thalami and lentiform nuclei, both of which are abnormally echoreflectant ( ). A clear and persistent abnormality of the thalami and basal ganglia on cUS correlates with MRI findings, which indicate a poor prognosis ( ) ( Fig. 40.37 ). Similarly, the appearance of a hyperechoic line running through the central grey matter is also a poor prognostic sign. Early detection may be facilitated by additional scanning with a low transducer frequency of 5 MHz – this lower transducer frequency allows deeper penetration in larger infants or those with thick hair and enables visualisation of deeper structures (i.e. the BGT in large infants and the posterior fossa) ( ). MRI is necessary to determine the exact site and extent of injury and involvement of the PLIC.

Due to its localisation at the brain’s convexity, the watershed or parasagittal injury pattern is not easily detectable with cUS. It often shows as a ‘tramline’ appearance of the cortex, that is, broadening of the hypoechogenic cortical rim combined with increased echogenicity of the subcortical white matter. Increasing the transducer frequency to 10 MHz will improve detection of this injury pattern with cUS; however, confirmation by MRI is indicated ( ). Doppler studies of CBF velocity showing a low resistance index are predictive of a poor outcome ( ).
Electrophysiology (EEG, aEEG, evoked responses)
Neurophysiology plays an important role in determining the severity of the insult in the newborn infant after hypoxia–ischaemia. In earlier studies the multichannel EEG was used to predict neurodevelopmental outcome ( ; ). In these studies, the EEG was usually performed after the first 12–24 hours. The electrical background activity was more predictive than the presence or absence of seizure activity. Since multichannel recordings have practical limitations, more compact systems, available within the intensive care unit, providing immediate access and storage of signals at the cot side over long period of time, are now increasingly being used. With this technique a single- or two-channel EEG is recorded from biparietal electrodes. The filtered signal is rectified, smoothed and amplitude-integrated before it is written out on a semilogarithmic scale at slow speed (6 cm/h). aEEG has a high concordance with the standard EEG ( ; ). The method is not always reliable for detection of seizures but works well in many babies and the neonatal staff can be taught to interpret the result. The results obtained using aEEG to predict outcome are similar to those obtained using EEG: a very early trace showing burst suppression or no activity can normalise by 6 hours and the baby may do well, but persisting burst suppression or isoelectric activity carries a poor prognosis ( ; ; ).
Over the last 20 years several studies have confirmed that the aEEG pattern in the first 6 hours of life is strongly predictive of outcome in infants with NE ( ; ). The speed of recovery, as well as the severity of the abnormality, in the aEEG trace is prognostically valuable ( ). The predictive value of aEEG has been shown to extend to infants who were monitored after 24 hours of age and to infants with encephalopathy caused by conditions other than hypoxia–ischaemia, such as metabolic conditions ( ), sepsis or meningitis ( ).
Sleep–wake cycling (SWC) is present in healthy term newborns and is a sign of brain integrity. A good neurodevelopmental outcome was predicted by the onset of SWC before 36 hours in 82% of infants with NE in one study ( ). Ideally, aEEG recording should be continued long enough for the onset of SWC to be determined.
The aEEG can be classified according to pattern or voltage criteria ( ; ). The voltage criteria are based on assessment of the lower and upper voltage margins of aEEG background ( ) ( Fig. 40.38 ). This method was used for selecting into the Cool-Cap and Whole Body Hypothermia for the Treatment of Perinatal Asphyxial Encephalopathy (TOBY) trials infants with encephalopathy ( ; ). The voltage classification ( Fig. 40.39a–d ) is easier to use for clinicians with little experience in reading aEEG, but one should always try to assess the underlying pattern ( Fig. 40.40a–e ). It has been shown that a burst suppression pattern may be read as a normal voltage pattern when a ‘drift of the baseline’ brings the lower margin above 5 µV ( ). The facility of inspecting the raw EEG in the new digital aEEG machines improves the accuracy of the interpretation of the aEEG. However, although some artifacts are easy to recognise, in many cases the recognition of an artifact requires an ability to interpret the EEG.











Recent studies show that therapeutic hypothermia changes the predictive value of early aEEG ( ; ). Normalisation of an infant’s aEEG while being cooled occurs later: in an infant who develops normally and who is not cooled, the aEEG would be expected to return to normal by 24 hours, whereas in an infant who is cooled and develops normally, it may take as long as 48 hours for the aEEG to normalise. In one study the time to normal aEEG was a better predictor than time to SWC; however never achieving SWC always predicted poor outcome ( ).
Computed tomography (CT)
CT scans have been used in the past in full-term neonates with hypoxia–ischaemia. MRI is superior to CT in all aspects and in most places CT has been replaced by MRI. Even the presence of calcifications, until recently only possible with ultrasound or CT scans, can be demonstrated with appropriate MRI sequences (susceptibility-weighted imaging). In a study comparing MRI with CT on day 3 in infants with NE, CT was able to detect the predominant pattern of injury with a 67% agreement with DWI MRI ( ). The risk of exposure to ionising radiation with CT must be kept in mind and MRI used if at all possible.
MRI and MRS
MRI is the optimal imaging modality for the early evaluation of brain injury in full-term neonates with perinatal asphyxia. Hypoxic–ischaemic changes of the brain can be visualised with higher spatial resolution, sensitivity and specificity than with cUS and CT. The pattern of brain injury may not be fully apparent until after the first 4–7 days on conventional MRI, but may be seen with DWI. The main patterns of injury are: (1) basal ganglia-predominant; (2) watershed-predominant; or (3) severe global injury. The severity and duration of hypoxia–ischaemia (acute total or chronic partial) influence the pattern of injury; the patterns of injury and their likely outcome are discussed in the section on patterns of injury, above.
Proton MRS is increasingly available and provides important prognostic data during the first week ( ; ). A reduction in NAA and elevation of Lac in the thalamus/basal ganglia ( ). Evolving abnormalities on MRS during the hours and days after birth and the use of MRS as a surrogate outcome measure are described in the section on cerebral energy metabolism associated with hypoxia–ischaemia, above.
DWI may help clinicians detect hypoxic–ischaemic lesions earlier than standard T1- or T2-weighted imaging. A reduced apparent diffusion coefficient can be calculated, showing reduced values (restricted diffusion) during the first few days after the insult, with pseudonormalisation by the end of the first week ( ) ( Fig. 40.41 ). Sequential imaging has shown that lesions in the basal ganglia may vary in size and site during the first week after birth ( ). The appearance of new areas of reduced diffusion simultaneous with pseudonormalisation of areas that had reduced diffusion at earlier times results in entirely different patterns of injury on ADC maps acquired at different time points.

Counselling
The parents of a baby with HIE experience acute and terrible distress: after many months of waiting for their baby to be born they are faced with the possibility of their child either dying or surviving with permanent disability. During the first few days after birth the senior attending physician, together with the nurse looking after the baby, should hold regular discussions with parents. A clear account of the baby’s condition and prognosis and an explanation of the therapeutic options should be provided (see section on neurodevelopmental outcome , below, for neuroprotective therapies). Several meetings will be required, not least because it is not possible to give an accurate prognosis very early in the course of HIE. The parents’ views are critical but the infant’s best interests must be kept in mind; in our experience it is usual to reach a consensus decision.
Redirecting care
Around 30% of infants who meet the criteria used in the cooling trials die following moderate/severe HIE. Most of these babies die following a redirection of medical care and discontinuation of assisted ventilation. The longer the baby remains in a severe encephalopathic state (stage 3 encephalopathy) with a severely suppressed aEEG/EEG (EEG voltage extremely low or absent), the more likely it is that there will be a severely abnormal outcome. Persistence of this state beyond 48–72 hours indicates that further life-supporting treatment is futile. If possible we obtain an MRI to confirm the diagnosis and severity of brain injury. We would not rely on aEEG alone, in case of artifact, and would seek to confirm a low-voltage trace with formal multichannel EEG.
Some infants have a partial recovery but the level of encephalopathy persists at stage 2/3, with the aEEG/EEG remaining moderately or severely abnormal (e.g. burst suppression pattern or a severely discontinuous pattern). Clinical assessment of the prognosis is difficult; however the prognosis may be better than expected following therapeutic hypothermia ( ).
The preterm infant with hypoxic–ischaemic injury
Regional differences in the vulnerability of the preterm compared to the term brain exist. In two studies evaluating brain injury patterns in preterm infants with evidence of perinatal asphyxial encephalopathy ( ; ), basal ganglia, thalamic and brainstem injury were seen with relative sparing of the perirolandic cortex. Cortical injury was commoner in older-gestation infants and less common in lower-gestation infants. found that preterm infants with a presentation consistent with HIE have a high incidence of severe BGT and brainstem involvement associated with significant mortality and neurologic morbidity. White-matter lesions were common, though often mild, and cystic periventricular leukomalacia was not found. Placental abruption was a clear risk factor. As with term HIE, early MRI in preterm infants with HIE allows accurate prediction of neurological outcome and therefore may contribute significantly to the management and clinical decision-making in this preterm group.
Neuroprotective treatments
The seminal discovery about perinatal hypoxia–ischaemia in the last century was that, although some brain injury can occur during a sufficiently prolonged/severe episode of hypoxia–ischaemia, in many cases damage actually evolves for hours after resuscitation, during the recovery period ( ; ). This evolution offers the prospect that there might be a ‘window of opportunity’ to provide treatment to reduce or prevent injury. This potential has been confirmed by the finding that therapeutic hypothermia can significantly reduce neurodevelopmental disability in infants with moderate to severe HIE at birth ( ).
Therapeutic hypothermia
A series of large randomised clinical trials has now shown that mild to moderate induced hypothermia ( ; ; ; ) (also termed targeted temperature management): (1) decreases death and disability among infants with less severe aEEG abnormalities ( ); (2) decreases death and moderate/severe disability; and (3) increases the number of survivors without disability ( ; ; ). Therapeutic hypothermia under intensive care settings appears safe; although thrombocytopenia and arrhythmias are more frequent with therapeutic hypothermia, these can be corrected with appropriate clinical care ( ; ). Inotropic support was used more commonly in cooled infants but this may have been due to physician bias ( ). Furthermore, there are suggestions that the favourable outcome of cooled infants at 18 months may be associated with favourable outcome at age 7–8 ( ).
Therapeutic hypothermia is now widely offered to infants with NE in high-income countries ( ). In the UK, in May 2010, the National Institute for Health and Clinical Excellence recommended that therapeutic hypothermia with intracorporeal temperature monitoring for hypoxic perinatal brain injury should be offered to ‘carefully selected infants … in units experienced in the care of severely asphyxiated infants [who] enter the details of infants undergoing cooling into the UK TOBY cooling register’ ( ; http://www.nice.org.uk/nicemedia/live/11315/48809/48809.pdf ). No large trials have assessed the safety and efficacy of therapeutic hypothermia in neonatal encephalopathy in low resource settings and there is concern that risk factors and population differences may alter risks and benefits of this therapy in such settings ( ).
Meta-analysis of trials with 18 months of follow-up suggests a number needed to treat of approximately 9, with a relative risk for death or disability of 0.81 (95% CI 0.71–0.93; P < 0.002) ( ). This advance is the culmination of >40 years of basic and clinical research ( ), and provides a strong platform from which to improve outcomes further. Eligibility criteria in the trials included a pH <7.0 or a base deficit of >16 mmol/L in umbilical cord blood or any blood during the first hour after birth. If blood gas was not available, additional criteria were required. These included an acute perinatal event and either a 10-minute Apgar score of 5 or less or assisted ventilation initiated at birth and continued for at least 10 minutes. A neurological exam with the presence of moderate to severe encephalopathy, and in some trials aEEG with specific findings, were required. Infants outside inclusion criteria for previously published clinical trials, including infants of 36 weeks’ gestation, infants who present outside the previously studied 6-hour window and infants with encephalopathy not attributable to HIE, remain in the unstudied realm for cooling therapy. US studies are underway to determine whether cooling for longer and deeper (96 hours to 32°C) provides better neuroprotection or whether cooling initiated after 12 hours from birth is beneficial.
Experimental studies, however, indicate that the earlier cooling is commenced, the better the outcome ( ). This is particularly critical since the therapeutic window is substantially reduced with increasing severity of injury ( ). Guidelines are in place for cooling infants prior to and during transport to a cooling centre in the UK ( ). Once in a cooling centre, servo-controlled cooling devices are generally used (Tecotherm and Criticool) and whole-body cooling is practised more frequently than selective head cooling ( ; ). Accumulating evidence from adult cooling studies emphasises the importance of rewarming as slowly as possible, by a maximum of 0.5°C over 2 hours. Key points in the clinical management during therapeutic hypothermia are shown in Table 40.23 and Figure 40.42 . It is very important to avoid overcooling below 30°C, as this has been associated with adverse outcomes in experimental studies ( ). It is essential to monitor rectal temperature continuously, especially during transport. In the UK, continued surveillance via the national registry is needed to detect unusual complications and audit in therapeutic hypothermia. In particular, further experience needs to accumulate in understanding the safety of therapeutic hypothermia applied to infants with systemic complications associated with perinatal asphyxia, such as pulmonary hypertension or myocardial ischaemia; none of the trials were powered to study this.
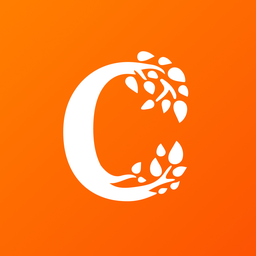
Full access? Get Clinical Tree
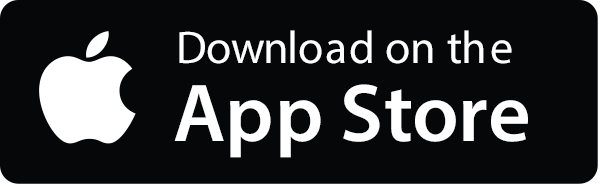
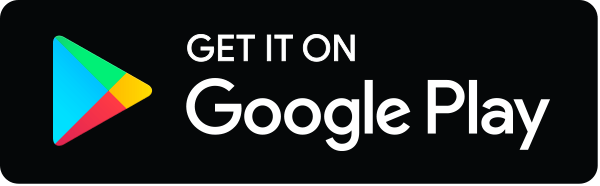