Neurological and Neuromuscular Disorders
Alan Hill
In the context of dramatic improvements in obstetric care and in the treatment of neonatal respiratory disorders and infection, neurologic problems remain a major cause of morbidity and mortality in the newborn. In this chapter, an approach to the neurologic assessment of the newborn is outlined, and practical aspects of diagnosis and management of the most common neurologic and neuromuscular problems of the newborn are reviewed (e.g., seizures, hypoxic-ischemic cerebral injury, intracranial hemorrhage, traumatic birth injury, spinal muscular atrophy, muscular dystrophies and myopathies). Major bacterial and viral infections, metabolic derangements that affect the central nervous system (CNS), and cerebral dysgenesis are discussed in other chapters.
NEUROLOGIC EVALUATION OF THE NEWBORN
History and Physical Examination
The importance of a detailed history and neurologic examination for assessment of the newborn with suspected neurologic problems cannot be overemphasized (1). Such history must include details of the family history and complications encountered during pregnancy, labor, and delivery, because many neurologic abnormalities that present during the neonatal period actually originate antenatally.
The format of the neurologic examination in the newborn is similar to that used in older patients. However, observations must be interpreted in the context of the level of cerebral maturation at different gestational ages (1,2). The examination should not be prolonged unnecessarily to avoid hypothermia and fluctuations in arterial blood pressure that may be associated even with routine handling, especially in premature newborns.
Neurologic observations also are influenced considerably by the level of alertness of the infant. After 28 weeks of gestation, infants are able to awaken spontaneously or may be roused for several minutes by stimulation. Distinct sleep-wake patterns may be recognized by 40 weeks of gestation.
In an examination of the cranial nerves, pupillary constriction to light and the blink reflex may be elicited as early as 28 weeks of gestation. Pupillary responses are consistent by 31 to 32 weeks. Consistent visual tracking of a bright light and opticokinetic nystagmus can be elicited consistently in the awake infant at term. Fundoscopy may reveal retinal hemorrhages in 20% to 50% of newborns following vaginal delivery, but these rarely indicate significant CNS injury. Dysconjugate and jerky eye movements are common in premature infants and may persist to some extent for several months after term. Full extraocular eye movements to doll’s-head maneuver or to caloric stimulation may be elicited after 32 weeks of gestation. Facial muscle weakness may be of central or peripheral origin. Hearing may be difficult to assess, although infants startle to loud noises as early as 28 weeks of gestation. The act of feeding requires coordination of breathing, sucking, and swallowing, which involve principally cranial nerves V, VII, IX, X, and XII. Therefore, observation of feeding provides valuable information regarding function of these cranial nerves and the brainstem in general.
The major features of the motor examination include evaluation of limb posture, spontaneous and elicited movements, muscle power, tendon reflexes, and primitive neonatal reflexes. Muscle tone may be assessed by careful observation of the infant’s posture at rest and during various maneuvers of passive manipulation. With increasing gestational age, there is development of predominance of flexor tone in all limbs. Asymmetry of muscle tone and spontaneous limb movements may indicate focal cerebral lesions or peripheral injury, e.g., brachial plexus injury. Tendon reflexes can usually be elicited in the term newborn. Undue emphasis should not be placed on brisk tendon reflexes, ankle clonus, or crossed adductor responses in the absence of corroborative abnormal neurologic signs. Muscle strength may be assessed by gently pinching the infant’s distal extremities and eliciting the recoil flexor response.
Careful evaluation of sensory function is particularly important in the evaluation of spinal cord or peripheral nerve injury. A useful aspect of sensory evaluation is the determination of habituation, i.e., the normal dampening of responses to multiple (e.g., 5-10) stimulations. Habituation indicates a high level of response that appears to require input from cerebral hemispheres. It is characteristically absent in anencephalic infants.
Neurodiagnostic Techniques
Because the neurologic examination is often limited by concomitant systemic illness and the requirement for complex life-support systems, a variety of noninvasive neurodiagnostic techniques, e.g., neuroimaging, electroencephalography, evoked potentials, often are used as adjunctive methods for the evaluation of neurologic problems. The clinical applications of various techniques will be discussed in the context of specific neurologic conditions.
NEONATAL SEIZURES
Seizures are the most distinctive manifestation of significant CNS disease in the newborn. Prompt diagnosis and intervention are indicated because not only do seizures often indicate serious underlying disease, they also may interfere with supportive care, e.g., ventilation and alimentation. Repeated seizures are often associated with hypoventilation and apnea which, in turn, may result in cardiovascular collapse and ischemic brain injury. Furthermore, the hypercapnia, in combination with increased lactate and an adaptive rise in systemic blood pressure, may result in an abrupt rise in cerebral blood flow with associated risk of intracranial hemorrhage especially in premature infants (3). Additionally, experimental animal studies suggest that neonatal seizures may have a deleterious effect on the developing brain by depleting cerebral glucose levels, which, in turn, may interfere with deoxyribonucleic acid (DNA) synthesis, glial proliferation, differentiation, and myelination (4,5). Although the relevance of data from experimental animal studies to the human newborn is not entirely clear, the significance of neonatal seizures has been corroborated by in vivo studies with magnetic resonance spectroscopy (MRS) (6). In terms of management, the possible detrimental effects of neonatal seizures must be balanced against the potentially deleterious effects of anticonvulsants on the developing brain (7).
Clinical Features
Neonatal seizures differ considerably from seizures observed in older children, principally because the immature brain is less capable of propagating generalized or organized electrical discharges. The principal types of neonatal seizures are summarized in Table 50-1 (8). Although individual seizure types are not indicative of specific varieties of brain injury, certain seizure types are associated more commonly with some conditions. For example, generalized tonic seizures, which may represent brainstem release phenomena or posturing, have been observed with major germinal matrix hemorrhage/intraventricular hemorrhage (GMH/IVH). Focal clonic seizures may be associated with focal cerebral infarction or traumatic cerebral contusion.
Differentiation of seizures from nonepileptic movements may be difficult in the newborn. Clonic seizures may be particularly difficult to differentiate clinically from jitteriness or tremulousness, particularly because both occur frequently in similar clinical contexts, e.g., hypoxic-ischemic encephalopathy, metabolic derangements, and drug withdrawal. However, jitteriness is classified as a movement disorder that may be associated with good outcome. Benign jitteriness often resolves within weeks. The distinction between jitteriness and seizures may be made clinically as follows:
-
Jitteriness is not accompanied by abnormal eye movements.
-
Jitteriness may be spontaneous or stimulus sensitive.
-
The flexion and extension phases of the tremor are equal in amplitude compared to the unequal phases observed with clonic seizure movements.
-
Jitteriness may be stopped by passive flexion or repositioning of the affected body part.
Infants with severe dysfunction of the CNS may have stimulus-sensitive myoclonus, which may be associated with spike or sharp wave discharges on the electroencephalogram (EEG) (9).
TABLE 50-1 CLINICAL FEATURES OF NEONATAL SEIZURES | ||||||||||
---|---|---|---|---|---|---|---|---|---|---|
|
Benign neonatal sleep myoclonus occurs during active sleep in healthy premature and term newborns. Myoclonus may be florid and consists of either bilateral synchronous or asynchronous or asymmetric movements that are not stimulus sensitive, but which cease on arousal from sleep. They are not associated with epileptiform or background disturbances on the EEG. Benign sleep myoclonus usually resolves over several months (10,11).
Simultaneous video and EEG monitoring has raised major issues concerning the incidence, classification, pathophysiology, and management of neonatal seizures. Some stereotypic, paroxysmal clinical phenomena, e.g., oral-buccal-lingual movements, generalized tonic, or extensor posturing are not associated consistently with epileptiform discharges on EEG recordings performed using surface electrodes. Subtle clinical phenomena correlate more frequently with simultaneous abnormal EEG discharges in premature than in term newborns (1,8).
Etiology
Neonatal seizures are rarely idiopathic in origin. Thus, when neonatal seizures occur, immediate attention must be directed toward the identification of an underlying etiology to permit rapid and appropriate intervention (when available) and meaningful prediction of outcome (8). Although neonatal seizures may be as a result of numerous underlying causes, most result from a relatively few causes, e.g., hypoxic-ischemic cerebral injury, intracranial hemorrhage, or metabolic derangements. The most important causes of neonatal seizures and their prognostic significance are listed in order of relative frequency in Table 50-2.
Seizures are a distinctly uncommon manifestation of withdrawal from passive addiction to narcotics, e.g., heroin, methadone, or barbiturates. In contrast, maternal cocaine abuse may be associated more commonly with epileptiform EEG abnormalities or seizures in newborns exposed in utero or by breast-feeding. This may relate to direct neuronal excitotoxicity, teratogenic effects, or destructive ischemic and hemorrhagic lesions (1).
Intoxication with local anesthetic after inadvertent injection into the infant’s scalp during placement of pudendal, paracervical, or epidural blocks is a rare cause of severe but self-limited neonatal seizures (12). Characteristically, severe, tonic seizures begin during the first hours of life, associated with coma and loss of brainstem reflexes, e.g., apnea and severe hypoventilation, bradycardia, hypotonia, fixed and dilated pupils, and absence of extraocular movements in response to the doll’s-head maneuver. The early onset of the latter two features during the first six to eight hours of life is useful for distinguishing such local anesthetic intoxication from hypoxic-ischemic encephalopathy. Evaluation involves careful inspection of the scalp for evidence of injection. Management consists of vigorous support and removal of the drug by diuresis, acidification of urine or exchange transfusion.
Neonatal seizures, which begin after several days of age, are a common manifestation of inborn errors of metabolism. There is increasing evidence that accurate diagnosis requires analysis of specific metabolites in cerebrospinal fluid and in serum (13).
TABLE 50-2 ETIOLOGY AND PROGNOSIS OF NEONATAL SEIZURESa | |||||||||||||||||||||||||||||||||||||||||||||||||||||||||||
---|---|---|---|---|---|---|---|---|---|---|---|---|---|---|---|---|---|---|---|---|---|---|---|---|---|---|---|---|---|---|---|---|---|---|---|---|---|---|---|---|---|---|---|---|---|---|---|---|---|---|---|---|---|---|---|---|---|---|---|
|
In addition to the underlying etiologies listed in Table 50-2, there are several recognized epilepsy syndromes that may present during the newborn period. These include two benign syndromes, i.e., “fifth day fits” and benign familial neonatal seizures. The latter often present as frequent seizures on the third day of life and usually are linked to autosomal dominant gene loci on chromosomes 20 or 8 (14,15). Additionally, there are several recognized severe epilepsy syndromes. Early myoclonic encephalopathy (EME) presents within hours of birth with severe, fragmentary, refractory myoclonus, which often is worsened by handling or stimulation. Infants often have a high-arched palate. The initial neuroimaging is normal, but diffuse cerebral atrophy develops and affected infants frequently die in the first 2 years of life. Another entity, early infantile epileptic encephalopathy, also termed “Ohtahara’s syndrome,” may present with structural cerebral lesions (dysgenesis or destruction) and a severe burst-suppression pattern on the EEG (16).
Diagnosis
Diagnostic evaluation must begin with a careful history and physical examination. Obtaining a maternal history of drug abuse, intrauterine infection, and genetic or metabolic conditions is critical, and it should be obtained directly from the mother whenever possible.
Although the EEG may be useful for confirmation of suspected seizures and for establishment of prognosis, it is rarely helpful for the identification of a specific etiology. Continuous EEG monitoring may be of value for identifying seizures in infants who are paralyzed pharmacologically or those who have electrographic seizures only.
Initial laboratory investigations should address treatable causes (e.g., hypoglycemia, hypocalcemia, hypomagnesemia). Lumbar puncture may identify intracranial infection or hemorrhage. Additionally, cerebrospinal fluid (CSF) analysis may also prove to be critical for identifying inborn errors of metabolism, e.g., folinic-acid responsive seizures and disorders of glucose transport, with low CSF glucose concentration and normal blood glucose concentration which, when treated with the ketogenic diet, may minimize long-term neurologic sequelae (13,17). If the initial screening investigations fail to identify a specific etiology, additional studies should be considered, including neuroimaging, metabolic investigations, e.g., serum amino acids, lactate, ammonia, urine organic acids, screening for drugs, and investigation for congenital viral infections. In some instances, several underlying factors may be operative in the same patient.
Treatment
Treatment of neonatal seizures is directed toward minimizing physiologic and metabolic derangements and preventing the recurrence of seizures. This should involve immediate support of ventilation and perfusion, if required, and correction of hypoglycemia, hypocalcemia, or other metabolic derangements. If seizures persist, a single loading dose of phenobarbital (20 mg/kg) should be administered intravenously, which may be followed by additional doses of 5 mg/kg to a total of 40 mg/kg (including loading dose) as required, if there is no cardiac decompensation. If seizures are still uncontrolled, a single loading dose of phenytoin (20 mg/kg) or possibly fosphenytoin (1.5 mg of fosphenytoin yields 1.0 mg of phenytoin) may be administered slowly with concomitant careful monitoring of cardiac function. If seizures remain refractory to therapy, the use of a benzodiazepine may be considered, e.g., lorazepam (0.05-0.1 mg/kg), or diazepam infusion (0.3 mg/kg/hr). Midazolam may have harmful effects in the newborn and should be avoided. Additionally, there are anecdotal reports of the value of other anticonvulsants for refractory seizures, e.g., primidone, carbamazepine, lamotrigine, and thiopentone (1).
Pyridoxine dependency should be considered if there are recurrent seizures without obvious explanation. This condition may be diagnosed by a therapeutic trial of intravenous pyridoxine (100 mg) with EEG monitoring or oral treatment (100-200 mg daily) which should be continued for approximately 2 weeks.
Several major issues regarding the ongoing treatment of neonatal seizures remain unresolved. These include the optimal maintenance doses and therapeutic ranges of serum levels of anticonvulsants, the importance of eliminating electrographic seizures, and the optimal duration of anticonvulsant therapy. The duration of therapy should be guided by the underlying etiology and the risk of seizure recurrence. Clearly, unnecessary prolongation of therapy should be avoided because of unresolved concerns about possible deleterious effects of anticonvulsants on the immature nervous system (7).
Prognosis
The mortality rate for poorly controlled clinical seizures has decreased to approximately 15% in recent years. The incidence of neurologic sequelae, especially mental retardation and motor deficits (e.g., cerebral palsy), are reported to range from 35% to 55% (1).
Of course, the most important determinant of outcome is the underlying neurologic disease (Table 50-2). Additionally, seizures of early onset, frequent or prolonged seizures, and seizures that are refractory to multiple anticonvulsant treatment are often associated with poor prognosis. Clearly, these features reflect the severity of the underlying cerebral abnormality. Furthermore, in term newborns, normal, background activity on the EEG is rarely associated with neurological sequelae, whereas severe abnormality of background activity, e.g., burst suppression or marked voltage suppression is associated with abnormal outcome in over 90% of cases. However, in a significant proportion of newborns, the EEG is borderline, equivocal, or contains moderate background abnormalities that are associated with an intermediate or uncertain prognosis. Furthermore, the unique features of the neonatal EEG may lead to difficulties in interpretation which, in turn, limits its prognostic value. Also, the number of electrographic seizures and the rate of improvement of serial EEG’s is also a useful prognostic factor (18).
HYPOXIC-ISCHEMIC CEREBRAL INJURY
Hypoxic-ischemic cerebral injury results from a combination of hypoxemia and ischemia, which often is associated with impaired cerebrovascular autoregulation and probably exacerbated by diminished cerebral glucose substrates, lactic acidosis, the accumulation of free radicals and excitotoxic amino acids (especially glutamate), and other metabolic derangements. The localization and extent of perinatal hypoxic-ischemic cerebral injury is determined principally by the maturity of the brain at the time of insult and the severity and duration of the insult (1).
Although the importance of antepartum and intrapartum factors have been recognized since 1862, when Little described a relationship between perinatal complications and cerebral palsy, data from large, epidemiologic studies, including the National Collaborative Perinatal Project and the Western Australia Cerebral Palsy Register, suggest that the etiologic role of antepartum factors may have been underestimated in the past (19,20,21). Review of these large populations of children with cerebral palsy indicate that the incidence of cerebral palsy related to intrapartum asphyxia do not exceed 20% when all factors are considered. Moreover, approximately one-third of these patients had at least one congenital anomaly unrelated to the CNS. This observation raises the possibility that an insult that originated much earlier during gestation may predispose some infants to subsequent hypoxic-ischemic insult at the time of delivery. Nevertheless, there are extensive experimental, clinical, and neuroimaging data that provide compelling evidence that acute, intrapartum hypoxic-ischemic insult is an important etiologic factor for neonatal brain injury (1,22).
Diagnosis
Clinical Features
Term newborns who sustain sufficient intrapartum insult to result in long-term sequelae invariably demonstrate clinical evidence of acute encephalopathy, i.e., altered brain function, during the first days of life (23). In contrast, infants who sustain hypoxic-ischemic cerebral injury earlier in gestation may be asymptomatic during the neonatal period. It is important to consider that the clinical features of hypoxic-ischemic encephalopathy are nonspecific, and similar clinical features may occur in the context of other causes of brain dysfunction, e.g., metabolic derangements, cerebral dysgenesis, and infection. Several aspects of labor and delivery may be used as clinical indicators of probable hypoxic-ischemic insult, e.g., prolonged fetal bradycardia or repetitive late decelerations of the fetal heart rate, low fetal scalp or cord pH, and low extended Apgar scores after 5 minutes of age. However, no single factor or combination of factors can predict accurately the severity or duration of the hypoxic-ischemic insult or the long-term sequelae (22). In the term newborn, the duration and severity of the encephalopathy, the type of EEG abnormality and the neuropathologic patterns of cerebral injury, which may be identified by neuroimaging, may permit determination of outcome with a reasonable degree of accuracy in many instances.
There is a complete spectrum of hypoxic-ischemic encephalopathy in the term newborn. However, classification of the severity of encephalopathy (Table 50-3) is useful for prognostic purposes (23,24,25,26,27). Thus, for example, according to the classification scheme developed by Sarnat and Sarnat (23), mild encephalopathy is characterized by hyperalertness, jitteriness, exaggerated tendon reflexes and Moro response that last only approximately 1 to 2 days. It is generally not associated with long-term neurologic sequelae. Moderate encephalopathy is comprised of lethargy, stupor, hypotonia, diminished spontaneous movements, suppressed tendon reflexes and, in some instances, seizures. Abnormal long-term outcome has been reported in 20% to 40% in this group. Severe encephalopathy is characterized by coma, seizures, brainstem and autonomic dysfunction, absent spontaneous movements, and, in some instances, elevated intracranial pressure (28,29,30). Infants with severe encephalopathy almost invariably die or develop major neurologic sequelae, e.g., microcephaly, spastic quadriplegia, seizures. In severe encephalopathy, the clinical features characteristically worsen during the first 3 days of life. Death occurs most commonly between 24 and 72 hours of life, which corresponds to the time of maximal cerebral edema (1,28). Autopsy studies of infants with increased intracranial pressure demonstrate extensive cerebral necrosis. Thus, increased intracranial pressure appears to be a consequence rather than a cause of extensive hypoxic-ischemic brain injury and interventions to reduce cerebral edema may reduce the intracranial pressure but do not appear to improve the ultimate neurologic outcome (28,31,32,33).
TABLE 50-3 SEVERITY AND OUTCOME OF HYPOXIC-ISCHEMIC ENCEPHALOPATHY IN THE FULL-TERM NEONATE | ||||||||||||||||||||||||||||||||||||||||
---|---|---|---|---|---|---|---|---|---|---|---|---|---|---|---|---|---|---|---|---|---|---|---|---|---|---|---|---|---|---|---|---|---|---|---|---|---|---|---|---|
|
Seizures occur in approximately 50% of asphyxiated infants and are indicative of moderate or severe encephalopathy. Seizures, which most commonly are of the subtle and multifocal clonic type, usually begin during the first 24 hours of life. In severe cases, they often occur soon after delivery and are refractory to anticonvulsant therapy. Infants with hypoxic-ischemic encephalopathy who survive beyond 3 or 4 days of age generally demonstrate an improving level of consciousness and gradual resolution of seizures, although impairment of feeding, abnormal muscle tone, and delayed development may persist.
In addition to the neurologic dysfunction, there is often evidence of acute hypoxic-ischemic insult to organs other than brain, e.g., kidneys are particularly vulnerable, but myocardial, GI, hematologic, endocrine and lung complications may also occur (34,35,36). Such involvement of other organs is often transient and reversible, even in the context of severe encephalopathy, and the extent of systemic abnormalities appears to correlate with the severity of encephalopathy. However, in some instances, especially when intrapartum asphyxia is severe and of short duration, as in the context of uterine rupture or cord prolapse, there may be little or no evidence of injury to organs other than the brain on routine investigations (37,38).
Neuroimaging
In addition to the clinical examination, neuroimaging techniques are of major value for the identification of specific neuropathologic patterns and quantification of extent of cerebral injury (39).
Cranial Ultrasound
In term newborns with severe hypoxic-ischemic injury, abnormalities include diffuse increased echogenicity of parenchyma and effacement of cortical sulci by cerebral edema during the first day of life. Unfortunately, if abnormalities are diffuse, detection may be difficult. This accounts for the disappointing correlation between ultrasound imaging and neurologic outcome in term newborns (39,40).
In term infants who have sustained an hypoxic-ischemic insult, cranial ultrasonography before 24 hours of age has a role for the exclusion of other entities, e.g., hemorrhage or mass lesions, which may produce a clinical picture which may mimic hypoxic-ischemic encephalopathy.
Ultrasound has better predictive value in premature infants with hypoxic-ischemic brain injury, in whom the principal lesions during in the first days of life are increased periventricular echogenicity and intraventricular hemorrhage. The hyperechoic periventricular abnormalities evolve into cystic lesions (cavitations) during the ensuing 2 to 3 weeks in severe cases (41,42). These lesions are transient and if the phase of cyst formation is not documented by serial ultrasound examinations, a later sonogram may show only enlargement of the lateral ventricles with irregular margins.
Current recommendations in many centers are that routine screening with cranial ultrasonography should be performed on all infants of less than 30 weeks gestation at least once between 7 and 14 days of age and should be repeated optimally between 36 and 40 weeks postmenstrual age. Of course, additional scanning may be indicated on the basis of clinical events.
Computed Tomography
In the term newborn, scanning with computed tomography (CT) during the newborn period has excellent prognostic value and may demonstrate diffuse or focal cerebral injury. Optimal timing of CT scans to demonstrate the maximal extent of decreased tissue attenuation is approximately between 2 and 5 days of age, which corresponds to the time of occurrence of maximal extent of cerebral edema (Fig. 50-1A) (28). Accurate interpretation of tissue attenuation on CT requires careful attention to scanning technique to ensure appropriate window and level settings. Two major patterns of hypoxic-ischemic brain injury may be identified on CT. The first pattern in which there is predominantly hypodensity of cortex and subcortical white matter with relative sparing of the deep central grey matter (28), corresponds to a neuropathological pattern which has been documented in experimental animal models following a period of “prolonged, partial asphyxia” usually lasting more than one hour. A second pattern is characterized by low attenuation in thalami and basal ganglia with relative preservation of cortex and subcortical white matter. This pattern occurs in the context of what has been termed an “acute, total” hypoxic-ischemic insult which was identified initially in experimental studies using fetal monkeys. Of course, in the human newborn such an insult should be more correctly termed “acute, near-total” in that the artificially created acute, total insult in the experimental animal studies never occurs even with acute and severe events such as umbilical cord prolapse and uterine rupture (37,43). CT scans performed later in childhood may demonstrate focal or generalized cerebral atrophy, often with multicystic encephalomalacia (Fig. 50-1B).
In contrast to the term newborn, CT is of more limited value for assessment of acute hypoxic-ischemic injury in premature newborns, because the normal high water content of the immature brain results in low tissue attenuation. However, CT scans performed at a later age may demonstrate the characteristic features of end-stage periventricular leukomalacia, which include (a) ventricular dilation with irregular margins of the body and trigone of the lateral ventricles, (b) decreased volume of periventricular white matter, and (c) deep sulci that abut directly on the lateral walls of the ventricles (44).
Magnetic Resonance Imaging
Magnetic resonance imaging (MRI) has the capability to demonstrate specific patterns of injury in both preterm and term newborns that may not be visualized by other imaging techniques (45,46,47,48,49,50,51). Thus, T2 prolongation resulting from edema (which may be transient or permanent) may appear as early as 12 to 18 hours after injury, and T1 shortening (high signal on T1-weighted images) appears in injured areas after approximately 3 days. Thus, if CT has been performed and is inconclusive, MRI should be performed between 2 and 10 days of age. Diffusion-weighted MRI appears to be even more sensitive than standard MRI during the first hours following hypoxic-ischemic injury (49,50). However, technical difficulties, e.g., the longer scanning time and difficulty with close monitoring of the sick newborn in the scanner, may limit the routine clinical application of MRI in the context of acute hypoxic-ischemic encephalopathy. At the present time, there are no large studies of CT or MRI to determine the role of MRI for the routine assessment of premature newborns with suspected hypoxic-ischemic brain injury.
Radionuclide Scanning
Radionuclide technetium scanning (52), positron emission tomography (53,54,55) and single photon emission CT (56), which utilize radionuclides that have the capability to cross the intact blood-brain barrier, can provide insights into regional abnormalities of cerebral perfusion. However, since advances in neuroimaging, especially MRI, have become widely available, these techniques are utilized less frequently in the routine clinical assessment of newborns with hypoxic-ischemic encephalopathy.
Magnetic Resonance Spectroscopy
In severely affected infants studied by phosphorus and proton MRS, a consistent observation has been the development of delayed derangements in cerebral energy metabolism between 12 and 48 hours after resuscitation, despite the maintenance of cardiovascular and respiratory homeostasis, which, in turn, correlates closely with poor neurologic outcome (57,58). However, because of the complexity and cost of the technique, MRS remains essentially a research technique available only on a limited basis at selected centers.
Near-Infrared Spectroscopy
Near-infrared spectroscopy (NIRS), a noninvasive technique that may be used at the bedside, has the capability to provide information concerning cerebral oxygenation and cerebral blood flow in real time. However, the technical limitations of the equipment and complexity of the data analysis have limited its application in critically ill newborns in all but a few centers. In a small number of infants with hypoxic-ischemic encephalopathy, significant cerebral vasodilation and vasoparalysis have been documented using NIRS (59).
Electrodiagnostic Techniques
Electrodiagnostic techniques are used in the evaluation of hypoxic-ischemic cerebral injury, especially in term newborns. On EEG recordings, a discontinuous pattern with voltage suppression or rapid bursts of sharp and slow waves are associated with poor outcome. In contrast, rapid resolution of EEG abnormalities and a normal interictal EEG pattern are associated with a good outcome (23,60,61,62).
Biochemical Markers
Several enzymes and metabolites (e.g., creatine kinase BB isozyme, hypoxanthine, lactate) in urine, blood, or CSF may be of value as indicators of hypoxic-ischemic cerebral injury (1). In this regard, the measurement of urinary lactate to creatinine ratios appears to be most promising (69). However, further study of the precise sensitivity and specificity of these metabolic parameters is required before they can be recommended for routine use. Furthermore, metabolic derangements that are considered secondary to the hypoxic injury, e.g., acidosis, hypoglycemia, and hyponatremia secondary to inappropriate secretion of antidiuretic hormone, and hyperammonemia may contribute to the cerebral injury.
Pathogenesis and Neuropathology
The pathogenetic mechanisms that determine the major neuropathologic patterns of injury may be explained by a combination of regional circulatory and metabolic factors of the affected brain, especially the regional distribution of excitatory amino acid synapses (e.g., glutamate receptors) and the severity and duration of the hypoxic-ischemic insult. The major neuropathologic patterns of hypoxic- ischemic cerebral injury are listed in Table 50-4.
Circulatory Factors
The initial circulatory response to perinatal asphyxia involves the redistribution of cardiac output with increased perfusion of vital organs, e.g., brain, heart, adrenals and with concomitant decreased blood flow to other organs, e.g., the lungs, kidneys, liver. Prolonged hypoxic-ischemic insult results in systemic hypotension. The significance of hypotension is potentiated by impairment of cerebrovascular autoregulation which may occur even after relatively moderate hypoxic-ischemic insult (70,71). Cerebrovascular autoregulation is the homeostatic mechanism that maintains relatively constant cerebral perfusion over a wide range of systemic arterial blood pressures by means of cerebral arteriolar constriction or dilation. As a consequence of the deficiency in the muscular lining of cerebral arterioles in the immature brain, the occurrence of hypercarbia or hypoxemia, or because the normal blood pressure of the newborn is close to the down slope of the normal autoregulatory curve, (or a combination of these factors), the protective mechanism of cerebrovascular autoregulation may be disrupted. This dysfunction of the cerebrovascular autoregulation mechanism results in a direct, linear correlation between cerebral perfusion and systemic blood pressure.
TABLE 50-4 MAJOR NEUROPATHOLOGIC PATTERNS | ||||||||||||||||||||||||||||
---|---|---|---|---|---|---|---|---|---|---|---|---|---|---|---|---|---|---|---|---|---|---|---|---|---|---|---|---|
|
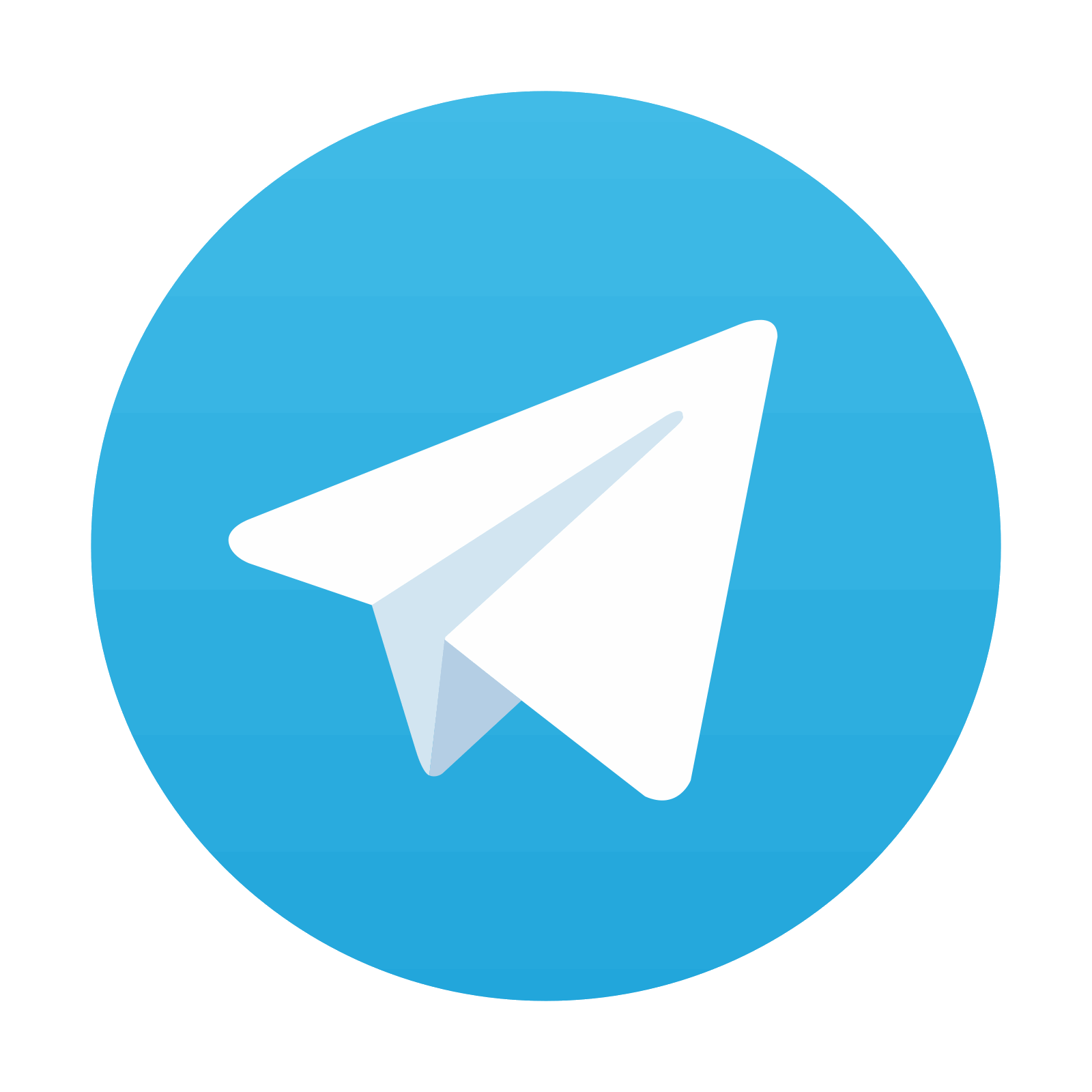
Stay updated, free articles. Join our Telegram channel
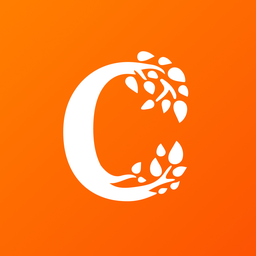
Full access? Get Clinical Tree
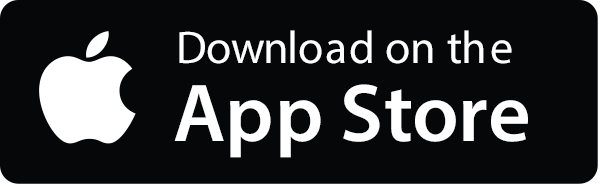
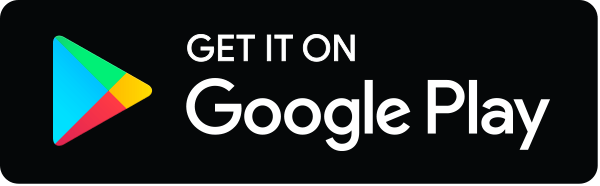