The use of respiratory support in the neonatal intensive care unit (NICU) to treat a variety of newborn respiratory conditions (e.g., respiratory distress syndrome and chronic lung disease) is quite common. Both the respiratory pathologies and the interventions used to treat these conditions have been linked to brain injury in preterm and term neonates. This chapter reviews (1) the basic physiology of cerebral blood flow in the neonate; (2) the common causes of neonatal brain injury, including their pathophysiology; (3) how carbon dioxide and oxygen influence brain injury; (4) how mechanical aspects distinct to the mode of ventilation affect brain injury; and (5) how medications used in respiratory management can modulate brain injury. The focus will be on preterm infants, as this is the population at the highest risk for permanent brain injury.
Cerebral Blood Flow in the Neonate
Cerebral blood flow (CBF) is tightly linked to cerebral metabolic demands in the normal brain. In the newborn infant, CBF is low, which corresponds to low neuronal activity in this patient population. Various methods of evaluation, including positron emission tomography, xenon clearance technique, ultrasound flowmetry, magnetic resonance imaging, and near-infrared spectroscopy (NIRS), indicate a wide range of CBF values of between 10 and 20 mL/100 g/min. These values are approximately one-third of the value for a healthy adult brain. A low CBF value in this patient population does not imply poor outcome, as the lower threshold required to maintain neuronal viability remains unknown. Interestingly, on the first day of life, CBF in preterm infants can be lower than the minimal CBF necessary to preserve viability and metabolism in the adult brain.
CBF is a function of cerebral perfusion pressure (CPP) and cerebrovascular resistance (CVR) as follows: CBF = CPP/CVR. Total and regional CBF, coupled with cerebral oxygen consumption, increases with postconceptual and postnatal age corresponding to increases in cerebral metabolic rates and energy demands. This increase is most prominent in the first day of life and probably represents a normal adaptive response of the cerebral circulation to postnatal life. Regional differences in CBF also reflect varying metabolic demands. For instance, blood flow to parasagittal and periventricular white matter is lower relative to that of other regions such as the cerebellum and basal ganglia.
Cerebral Autoregulation and Pressure-Passive Circulation
Cerebral autoregulation is the normal intrinsic ability of the cerebral blood vessels to maintain relatively constant CBF over a range of mean arterial blood pressures (MABPs) ( Fig. 42-1 ). As CPP decreases, CVR also decreases by way of alterations in the diameter of the precapillary arterioles, thus maintaining CBF. This adaptive ability has a limited capacity and will result in a decrease of CBF when the blood pressure falls below a certain threshold and, conversely, will increase when blood pressure reaches an upper threshold. This is referred to as a loss of autoregulation, or a pressure-passive state.

Cerebral autoregulation appears to be intact in fetal and neonatal animal models. It also appears to be intact in the stable human preterm infant. In a study of extremely preterm infants with median gestational age of 24 weeks, CBF was found to be low (range of 4.4 to 11 mL/100 g/min), with no relationship of CBF to systemic blood pressure, suggesting intact autoregulation. In another study of preterm infants with a mean gestational age of 26 weeks, normotensive infants (MABP 37 ± 2 mm Hg) were shown to have intact autoregulation, although there was a loss of autoregulation in those who became hypotensive (MABP 25 ± 1 mm Hg). This study also identified an MABP threshold of ∼30 mm Hg, below which the CBF became pressure passive. However, a definition of this lower threshold remains elusive. Victor et al. analyzed electroencephalography (EEG) patterns in 35 preterm infants (median gestational age 27 weeks) daily until day 4 of life. Notably, four infants had abnormal EEGs, and the MABPs in these infants were significantly lower than those with normal EEGs (22 vs 33 mm Hg, p < 0.001). This suggests that the lower limit of MABP for autoregulation was in the range of 22 to 33 mm Hg in this cohort. Another study showed no difference in CBF between a group of preterm infants with MABP of less than 30 mm Hg and another group with MABP greater than 30 mm Hg.
In summary, although cerebral autoregulation has been documented in the premature infant, it appears to function within a limited blood pressure range and is impaired or absent in the sick hypotensive preterm infant. This vulnerable state places the developing brain at extraordinary risk for injury during times of hypotension and/or elevated blood pressures. As such, CBF is one of the most important factors contributing to the major forms of preterm brain injury, as will be detailed subsequently.
Brain Injury in the Preterm Infant
The most common forms of brain injury in the underdeveloped premature brain are periventricular–intraventricular hemorrhage (IVH, including periventricular hemorrhagic infarction [PVI]) and periventricular leukomalacia (PVL). These lesions are more likely to occur in the smallest, most immature infants with respiratory distress syndrome requiring mechanical ventilation. The majority of permanent long-term neurologic sequelae in preterm infants are a result of these two forms of brain injury.
Periventricular–Intraventricular Hemorrhage
The overall incidence of IVH has declined over the past few decades, although severe hemorrhage continues to be a significant morbidity in the increasing population of very low birth-weight (VLBW) survivors. The incidence and severity of IVH are inversely proportional to gestational age. About 25% of infants between 501 and 750 g and 14% between 751 and 1000 g still develop the most severe forms of hemorrhage, with the incidence remaining essentially unchanged since the mid-1990s.
The primary lesion in IVH is bleeding from the periventricular subependymal germinal matrix. This matrix is a transitional region of neuronal and glial precursor cells that migrate to other regions of the cerebrum. Notably, it is highly cellular, richly vascularized, and gelatinous in texture. It is located between the caudate nucleus and the thalamus at the level of, or slightly posterior to, the foramen of Monro. It begins to involute after 34 weeks, and by term gestation, it is essentially absent. Destruction of the germinal matrix may result in impairment of myelination, brain growth, and cortical development.
Reviewing the vascular supply of the germinal matrix is crucial to understanding its propensity to bleed. The immature capillary network of the germinal matrix is primarily supplied by a branch of the anterior cerebral artery (Heubner’s artery), striate branches of the middle cerebral artery, and the anterior choroidal artery. Venous drainage incorporates a system of medullary, choroidal, and thalamostriate veins that link to form the terminal vein. Where the terminal vein and the internal cerebral vein join, the venous flow makes a striking U-turn before continuing on to join the vein of Galen. The venous anatomy suggests that elevated venous pressure secondary to obstruction of the venous drainage may lead to venous distention and rupture ( Fig. 42-2 ). Moreover, hypotension leading to decreased CBF probably also plays a role in the genesis of IVH in some infants. This presumably occurs via a mechanism of ischemia–reperfusion with rupture of blood vessels upon reperfusion. Precise description of the vasculature of the germinal matrix has been elusive because the small capillaries, venules, and arterioles that populate the matrix are hard to distinguish from one another histologically due to their relatively simple endothelial wall structure.

The physiologic factors contributing to increased risk of IVH are complex and incorporate a combination of vascular, intravascular, and extravascular influences ( Box 42-1 ). Intravascular factors, especially those that involve perturbations in CBF and volume, play a critical role in the development of hemorrhage. A pressure-passive circulation in the sick preterm brain leads to direct changes in CBF with changes in systemic blood pressure. Acute changes in blood pressure and cerebral perfusion are likely to lead to disruption of the vulnerable blood vessels in the germinal matrix. Fluctuating CBF velocity in the VLBW infant also predisposes the infant to hemorrhage. Physiologic increases in cerebral venous pressure exacerbate the already compromised venous drainage of the terminal vein. These physiologic disturbances may be from a variety of factors, such as the labor and delivery process and intrathoracic pressure changes induced by the ventilator or air leaks. Intrathoracic pressure is directly transmitted to cerebral vessels, and these fluctuations are applied to vessels probably already maximally dilated with little autoregulation. Another intravascular component that has been implicated in playing a role in IVH is inflammation, which has been correlated with increased risk of IVH. Although precise mechanisms are unclear, increased interleukin (IL)-1β, IL-6, and IL-8; mononuclear cells; and other inflammatory markers have been increased in the fetal and neonatal period in infants with IVH. Similarly, both clinical and histologic chorioamnionitis have been associated with hemorrhage. Conversely, antenatal steroids decrease the risk. The protective effect of steroids may in part be related to enhanced support for the blood vessels within the germinal matrix. The general vascular support of the germinal matrix capillaries is poor, with increased fibrinolytic activity and decreased glial stabilization. Finally, the germinal matrix capillaries themselves are quite tenuous, as they are in a constant state of involution and remodeling. Their vascular lining is deficient, and they reside in an end-arterial zone between the striate and the thalamic arterial distribution.
Cerebral factors | Vulnerable cerebral vascular beds |
Pressure-passive circulation | |
Respiratory factors | Respiratory distress syndrome |
Pneumothorax | |
High mean airway pressure | |
Hypocarbia, hypercarbia | |
Hypoxia, hyperoxia | |
Vascular factors | Hypotension, hypertension |
Blood pressure fluctuations | |
Perinatal factors | Chorioamnionitis |
Metabolic factors | Acidosis, alkalosis, hypernatremia |
Antenatal interventions | Prevention of premature delivery |
Antenatal corticosteroids | |
Maternal transfer to perinatal center | |
Intrapartum interventions | Mode of delivery |
Postnatal interventions | Avoidance of hemodynamic fluctuations, e.g., sedation |
Synchronized mechanical ventilation | |
Administration of surfactant (?) | |
Minimizing complications of RDS, e.g., pneumothorax | |
Minimize mean airway pressure | |
Minimize the hemodynamic effects of PDA | |
Avoidance of extremes of PaCO 2 | |
Avoidance of metabolic disturbances, e.g., metabolic acidosis | |
Minimize postnatal steroid use | |
Use of caffeine in apnea of prematurity |
PDA , Patent ductus arteriosus; RDS , respiratory distress syndrome.
In most cases the diagnosis of IVH is made with screening ultrasound. The most vulnerable period is the first few postnatal days, with greater than 50% occurring in the first 24 hours and 90% in the first 72 hours of life. Risk factors associated with the development of IVH include low birth weight and gestational age, maternal smoking, breech presentation, premature rupture of membranes, postnatal resuscitation and intubation, early-onset sepsis, respiratory distress syndrome (RDS), pulmonary air leaks, metabolic acidosis, and rapid bicarbonate infusions. Extremes of arterial PaCO 2 in the first 4 days of life are also associated with severe IVH (see below).
Periventricular Hemorrhagic Infarction (Grade 4 Intraventricular Hemorrhage)
One of the complications of IVH is PVI, also termed grade 4 IVH . PVI is a hemorrhagic venous infarction that is associated with severe and usually asymmetric bleeding. It occurs in about 15% of infants with IVH, particularly the smallest, most immature ones. On brain imaging, there is often a large, fan-shaped region of hemorrhagic necrosis in the periventricular white matter, invariably on the side with the larger amount of intraventricular blood ( Fig. 42-3 ). A variety of studies have shown that this lesion is not merely an extension of IVH. Instead, the likely mechanism is the obstruction of the medullary and terminal veins by the intraventricular and germinal matrix blood clot, leading to venous congestion in the periventricular white matter with subsequent hemorrhage and ischemia. PVI is associated with a high rate of mortality (40% to 60%), with survivors being at very high risk of cerebral palsy (CP) (66% in one study) and other neurologic abnormalities.

Periventricular Leukomalacia and Diffuse White Matter Injury
Focal and diffuse white matter injury (WMI) may occur adjacent to the lateral ventricles with or without associated hemorrhage—a condition referred to as PVL ( Fig. 42-4 ). The sonographic findings include cyst formation, ventriculomegaly, and diffuse WMI. This last form often manifests with ventriculomegaly in the absence of cyst formation and occurs most commonly in premature infants who require prolonged ventilator support. The pathogenesis of WMI is complex and involves vascular factors, as well as the intrinsic vulnerability of oligodendrocytes to noxious substances. The vascular factors include the following: (1) The white matter resides in a border zone region, which increases the likelihood of injury during periods of systemic hypotension ( Fig. 42-5 ). (2) The risk for injury to these regions is increased in the face of a pressure-passive circulation as discussed above. (3) There is a limited vasodilatory response to PaCO 2 of those vessels supplying the white matter.


The intrinsic vulnerability of the early differentiating oligodendrocyte has been established in studies that show that these cells are sensitive to injury secondary to the release of numerous factors, including free radicals, excitotoxins (glutamate), and cytokines, as well as a lack of growth factors. The importance of intrinsic vulnerability is suggested from a report of 14 of 632 infants (2.3%) weighing less than 1750 g at birth who developed bilateral cystic PVL. Overt hypotension occurred in only four of the babies with PVL, mostly in the immediate postnatal period. In the other 10 cases, PVL was seen in infants with mild to moderate lung disease without hypotension and was detected only on routine ultrasound screening. Two conditions were found to be significantly associated with development of PVL in this cohort—chorioamnionitis and prolonged rupture of membranes. Other conditions that have been associated with the development of PVL include hypocarbia, meningitis, and recurrent severe apnea and bradycardia. Surviving preterm infants with PVL are at risk for CP (primarily spastic diplegia) and other motor deficits. Cognitive problems are also common, along with more subtle behavioral and attention disturbances.
Influence of Oxygen Concentration and Carbon Dioxide on Cerebral Blood Flow
As discussed above, abnormal CBF is one of the most important pathophysiologic factors in preterm brain injury. The cerebral circulation of the healthy newborn infant, and even in the very preterm infant, responds to physiologic stimuli in much the same way as in the adult. Cerebral blood vessels are sensitive to changes in PaCO 2 , arterial oxygen concentration (Ca o 2 ), and pH. In pathologic situations, a pressure-passive state occurs and cerebral blood vessels may not react to chemical or metabolic stimuli. These infants are at increased risk for developing hemorrhagic and/or ischemic cerebral injury.
Oxygen and Hemoglobin
CBF increases when arterial oxygen tension decreases markedly in the human infant and in neonatal animal models. CBF is regulated by Ca o 2 , which in turn is determined by hemoglobin concentration, oxygen affinity to hemoglobin, and PaO 2 . In preterm infants studied in the first 3 days of life, it has been shown that CBF increases by approximately 12% for every 1-mM decrease in hemoglobin concentration. There is also a direct relationship of CBF with the relative proportion of fetal hemoglobin, probably due to the stronger affinity of fetal hemoglobin for oxygen.
Cerebrovascular dilation occurs within 30 to 60 seconds in response to hypoxia. At lower blood pressures, the vasodilator response to hypoxia may be impaired. Conversely, hyperoxia induces a fall in CBF in preterm infants through cerebral vasoconstriction. Median reduction was found to be 0.06 cm/s for every 1-kPa increase in oxygen tension. When 80% oxygen was given during resuscitation at birth, preterm infants were subsequently shown to have a 25% reduction in CBF velocity versus those infants given room air.
Carbon Dioxide
The fetal and neonatal brain remains sensitive to changes in PaCO 2 ; hypocarbia decreases CBF through vasoconstriction of cerebral arteries and hypercarbia has a relaxant effect. The primary mediator linking arterial CO 2 tension and cerebral vasoreactivity may be pH. In one study performed on isolated dog cerebral arteries, hypercarbia-induced cerebral arterial relaxation was shown to be mediated mainly with a fall of extracellular pH. Because CO 2 crosses the blood–brain barrier readily, abrupt changes in CO 2 tension may also cause a rapid change in vascular reactivity within 1 to 2 minutes. This acute effect is then actively regulated by perivascular pH causing the vessel diameter to normalize gradually during the next 24 hours.
In the healthy adult, CBF changes by a mean of approximately 30% for every 1-kPa change in PaCO 2 . Similarly, in spontaneously breathing preterm infants studied at 2 to 3 hours after birth, CBF–carbon dioxide reactivity was shown to be approximately 30% per 1-kPa change in PaCO 2 . In mechanically ventilated preterm infants, this reactivity was much less, that is, ∼11% per 1-kPa change in PaCO 2 when studied shortly after birth, although it increased to near-adult levels by the second day of life. In the infants in whom severe intracranial hemorrhage subsequently developed, there was a loss of CBF reactivity to changes in PaCO 2 , implying an impairment of CBF regulation before hemorrhage.
Linking Changes in Carbon Dioxide and Oxygen Concentration to Hemorrhagic–Ischemic Injury
Hypocarbia and White Matter Injury
Hypocarbia is a common occurrence in the ventilated preterm infant. It may be seen as a result of improved lung compliance and/or function or with aggressive ventilator support (either unintentional or intentional). Several studies have reported the association of hypocarbia with PVL, neurodevelopmental problems, and CP. In one study, time-averaged PaCO 2 on the third day of life was lower in preterm infants who developed PVL than in those who did not develop PVL. Others have supported a similar dose-dependent effect of hypocarbia, with longer and more frequent episodes of hypocarbia associated with more severe brain injury. In full-term infants, hypocarbia following perinatal hypoxia–ischemia is associated with adverse outcomes, as evidenced by a retrospective study of nearly 250 newborns. This was also noted in the National Institute of Child Health and Human Development Neonatal Research Network’s trial of whole-body hypothermia. When early blood gases were examined (from birth through 12 hours of hypothermia), infants with death or disability had significantly lower minimum P co 2 concentrations (median 22 vs 26 mm Hg, p = 0.15), and this was a predictor of outcome. This relationship also held true in those infants exposed to a higher cumulative duration of hypocarbia, as they also had worse outcomes. Despite these correlations, it remains unclear whether hypocarbia leads to brain injury or is merely an early marker of increased risk.
Hypercarbia and Intraventricular Hemorrhage
Permissive hypercarbia has been advocated as a ventilatory strategy to minimize barotrauma and volutrauma to the lungs of preterm infants and thus prevent evolution to chronic lung disease. Although there may be beneficial effects in terms of lung injury, the risks of elevated PaCO 2 to the brain must not be ignored. As reviewed earlier, hypercarbia is associated with both an increase in CBF and an impairment of cerebral autoregulation in ventilated VLBW infants. In a study undertaken in the first week of life in VLBW infants of gestational age 26.9 ± 2.3 weeks, increasing PaCO 2 resulted in increasing impairment of cerebral autoregulation. Hypercarbia, defined by the maximum PaCO 2 recorded during the first 3 days of life, was also associated with severe IVH in a retrospective cohort study of 574 VLBW infants. As maximum PaCO 2 increased from 40 to 100 mm Hg, the probability of severe IVH increased from 8% to 21%. A retrospective review of 849 infants weighing less than 1250 g suggested that extremes in PaCO 2 , both hypocarbia and hypercarbia, as well as fluctuations in PaCO 2 during the first 4 days of life, increased the risk of severe IVH. In this analysis, infants who developed severe IVH had higher maximum PaCO 2 (median: 72 vs 59 mm Hg, p < 0.001), lower minimal PaCO 2 (median: 32 vs 39 mm Hg, p < 0.001), and a greater range between maximum and minimum PaCO 2 values (median: 39 vs 21 mm Hg, p < 0.001). This was reinforced in a reanalysis of data from the SUPPORT trial. In over 1300 infants from 24 to 27 6/7 weeks, severe IVH was increased in those with PaCO 2 levels in the highest quartile. These infants were also at increased risk of neurodevelopmental impairment. Similarly, infants with a large range between maximum and minimum PaCO 2 (i.e., fluctuators) had increased incidences of neurodevelopmental impairment as well as bronchopulmonary dysplasia (BPD). Comparable results linking increased PaCO 2 fluctuations with IVH were shown in a retrospective analysis by Altaany et al. Taken together, these studies indicate that extremes in PaCO 2 should be avoided during the period in which infants are at high risk of IVH.
Oxygen and Brain Injury
Fraction of inspired oxygen (FiO 2 ) is a parameter that must be chosen by the provider when respiratory support is initiated—this may range from room air to 100% O 2 . Clinicians often use oxygen saturation (SpO 2 ) and PaO 2 to subsequently guide their administration, varying their targets based on factors such as gestational age, risk of retinopathy of prematurity, and heart and lung disease. Although the optimal saturation range of preterm infants is unclear, it is known that the extremes of both hypoxia and hyperoxia can have adverse effects on the developing brain. The SUPPORT trial randomized infants to SpO 2 targets of either 85% to 89% or 91% to 95% to address this question. Although there were no differences in IVH or PVL, the lower oxygen-saturation group had an increased incidence of death (19.9% vs 16.2%; p = 0.04), and the higher oxygen-saturation group had an increased incidence of severe retinopathy (17.9% vs 8.6%; p < 0.001).
The effect of hyperoxia on the preterm infant has received considerable attention. As reviewed earlier, Ca o 2 is a primary regulator of CBF. The preterm infant often has labile oxygen saturations and therefore CBF, which increases the risk of IVH. At a biochemical level, hyperoxia has also been shown to have deleterious effects on the preterm brain through mechanisms of inflammation, apoptosis, and oxidative stress. Excess oxygen can lead to the generation of reactive oxygen species. These free radicals can be harmful to preterm infants because of their already low antioxidant defense compared to term infants. Of particular importance, premyelinating, or immature, oligodendrocytes are vulnerable to oxidative injury, which may be a potent contributor to PVL in a preterm infant receiving excessive oxygen therapy.
In addition to SpO 2 and PaO 2 , the measurement of regional tissue hemoglobin oxygen saturation (rSt o 2 ) has been gaining popularity. Using NIRS, rSt o 2 can be measured noninvasively in various circulatory regions (e.g., cerebral, splanchnic, etc.). A trial of 166 preterm infants of less than 28 weeks’ gestation randomized subjects to visible or blinded cerebral NIRS monitoring for the first 3 days of life. The visible NIRS subjects were managed with the goal of maintaining rSt o 2 between 55% and 85% by targeting respiratory and circulatory parameters. While the treatment group did maintain rSt o 2 in the target range better than the control group, no significant differences in clinical outcomes were found.
In summary, both low and high blood oxygen concentrations have harmful effects on the brain. Pulse oximetry and PaO 2 are the mainstays of adjusting oxygen therapy, but new technologies, such as NIRS, may have a prominent role in the future.
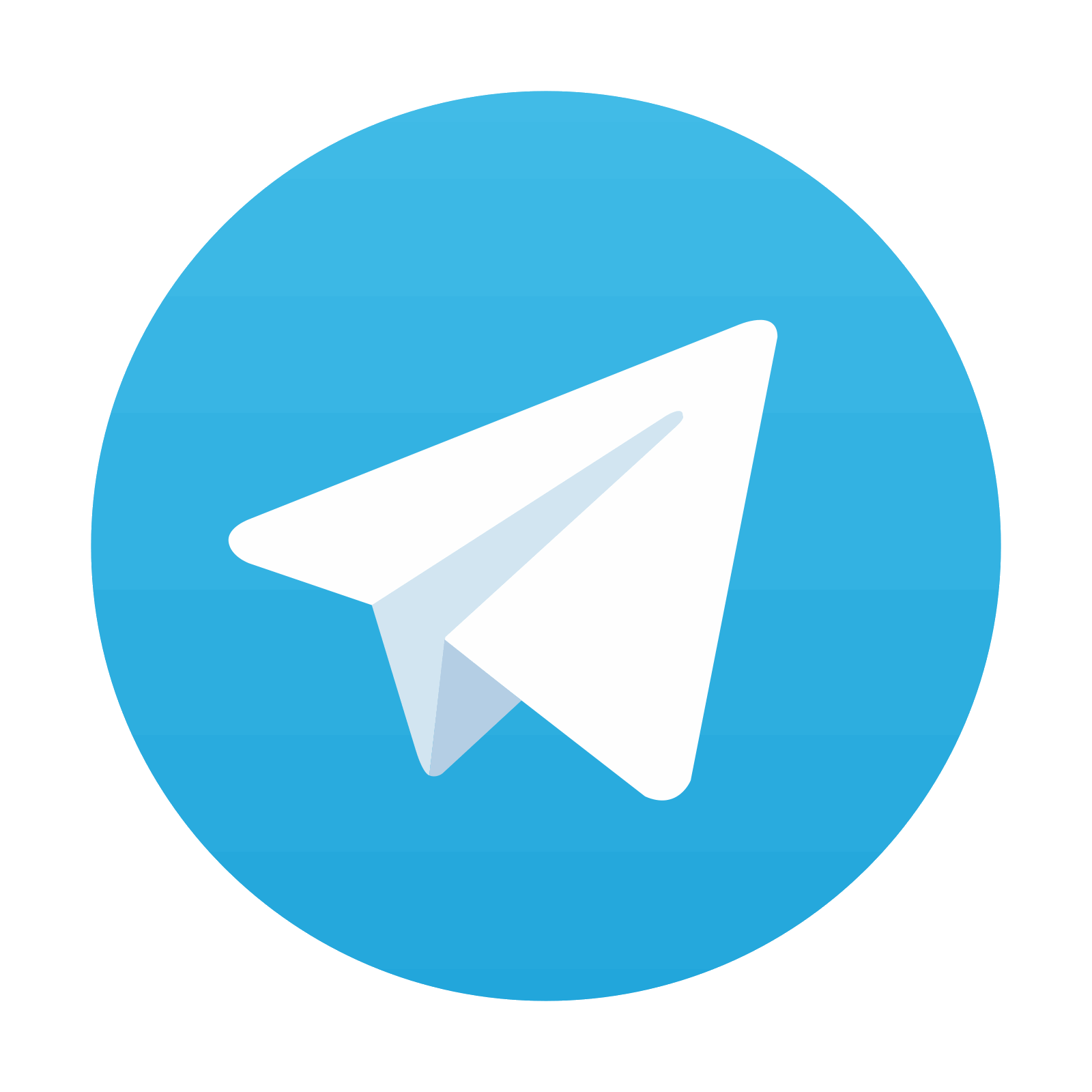
Stay updated, free articles. Join our Telegram channel
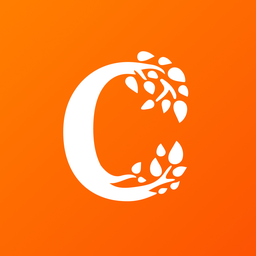
Full access? Get Clinical Tree
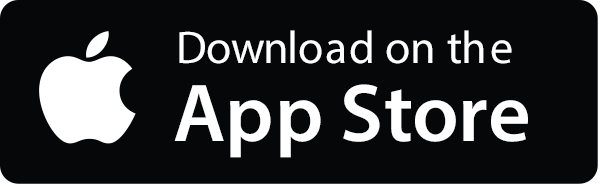
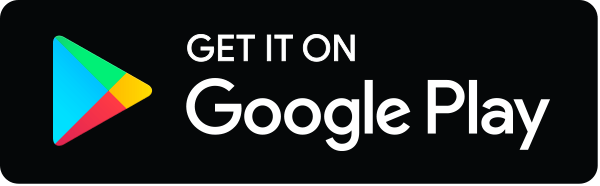