In human pregnancy, the developing individual is considered to be an embryo until the end of the eighth week after fertilization of the ovum. After this time the individual is referred to as a fetus. Gestational age refers to the interval between the first day of the mother’s last menstrual cycle and the current date (e.g., the date of a sonogram or delivery). Gestational age is therefore post-fertilization age plus two weeks. A detailed consideration of human neuroembryology is beyond the scope of this book. The reader is referred to the many publications of O’Rahilly and Muller, including their masterful textbook (1). Other texts and atlases are also very useful (2–4).
George L. Streeter was director of the Department of Embryology at the Carnegie Institution of Washington. In the early 1940s, he developed a standardized system of 23 stages (the “Carnegie stages”) that could be used as a timetable to compare across species the developing vertebrate embryo (5, 6). The stages are delineated through the development of structures, not by size or age (7).
In practical terms, the pathologist seldom encounters or is expected to perform a detailed examination of an embryo. We only occasionally encounter fetuses prior to ~15 weeks gestational age. However, the stages of development must be known in order to recognize normal brain development and to understand major malformations.
It is critical to keep in mind that cell proliferation and fate determination, cell migration, and cell maturation are sequential for an individual cell, but that all three processes occur simultaneously when considering different cell populations within a given region of the central nervous system (8). Furthermore, the timing of these events varies widely by region, with the spinal cord and brainstem maturing much earlier than the cerebral hemispheres (9). As the neural tube forms, most cell proliferation occurs along the internal wall within the ventricular and subventricular zones. Daughter cells migrate outward and begin to differentiate into neuron precursors that form the laminar neocortex very early (10, 11; Figures 19.1 and 19.2). Axons from the cortex begin growth into the intermediate zone (IZ) at stage 22; the IZ is well populated by axons at 8 weeks post-conception. By the end of the embryonic period, the brain has developed at least rudiments of most major structural features, with the exception of the corpus callosum. At this stage, the wall of the telencephalon is thin and the lateral ventricles are relatively large (Figure 19.3). Corticofugal and thalamocortical axons can be identified, followed by the growth of commissural and association fibers during the second and third trimesters (12, 13).
Figure 19.1 Diagrams showing cytologic development of human neocortex as a function of post-fertilization age. The main proliferative sites are the ventricular and subventricular zones, although mitoses occur with less frequency in all layers. Abbreviations: CP – cortical plate, IZ – intermediate zone, MZ – marginal zone, PP – preplate, SG – subpial granular layer (part of the MZ), SP – subplate zone, SVZ – subventricular zone, VZ – ventricular zone.
Figure 19.2 Diagram showing neuronal differentiation in human embryonic brain from Carnegie stage 16 to stage 22. Reelin (Reln) immunoreactive neurons (blue) appear in the marginal zone (outer neural tube) at stage 16, and then increase in quantity. Calretinin (CR) immunoreactive neurons (light green) appear at stage 19 in the preplate. Glutamic acid decarboxylase (GAD) immunoreactive neurons (red) appear at stage 20 in the preplate while Reln neurons move to the subpial compartment. At stage 21, maturing pioneer neurons (dark green) send axons into the intermediate zone (arrow). At stage 22, cortical plate neurons (yellow) migrate from the ventricular zone along radial glia (rg) into the cortical plate. Abbreviations: IZ – intermediate zone; MZ – marginal zone; PP – preplate; VZ – ventricular zone.
Figure 19.3 Coronal section through the frontal lobes at the end of the embryonic period (Carnegie stage 23). A prominent ventricular zone (dark band, arrows) surrounds the lateral ventricles. Ch Pl – choroid plexus, Gp – globus pallidus, Ins – insula, Put – putamen, V3 – third ventricle. Bar = 200 µm.
Tables 19.1 and 19.2 show highlights of central nervous system development during the embryonic and early fetal stages.
Post-fertilization age in days (length of embryo or fetus) | Carnegie stage (embryos only) | Important milestones | References |
---|---|---|---|
1 | 1 | fertilization of the ovum | |
2–3 | 2 | 16 cell spherical morula | |
4–5 | 3 | contact with the endometrial lining of the uterus | |
6 | 4 | ||
7–12 | 5 | implantation into endometrium; development of amniotic cavity; two layered embryonic disc | |
17 | 6 | formation of primitive streak and primitive groove | |
19 | 7 | formation of notochord | (15, 16) |
23 (1–1.5 mm) | 8 | formation of neural ectoderm and neural plate | |
26 | 9 | formation of neural groove, neural folds, and early heart; neural crest appears at mesencephalon level | (17) |
29 (2–3.5 mm) | 10 | neural folds begin fusion at rostral myelomeric level (future spinal cord) (Figure 19.4) | |
30 (2.5–4.5 mm) | 11 | closure of rostral neuropore; optic vesicles appear (Figure 19.5) | (18) |
30 | 12 | closure of caudal neuropore; glial cells begin to develop in ventricular and subventricular layers of the prosencephalon; secondary neurulation begins at caudal end of neural tube (upper sacral level); pia mater appears at mesencephalic level | (19–21) |
32 | 13 | cerebellum rudiments appear | |
33 (5–7 mm) | 14 | future cerebral hemispheres identifiable as bilateral bulges from prosencephalon; neurogenesis begins in telencephalon; radial glia present; blood vessels begin to penetrate wall of brain | (21–25) |
35 (7–9 mm) | 15 | clearly defined telencephalon, diencephalon, mesencephalon, and rhombencephalon; appearance of telencephalic lateral ventricular (ganglionic) eminence; radial glia immunoreactive for WT1 (Figure 19.6) | (26, 27) |
38 | 16 | Reelin expressing neurons appear in marginal zone; all cranial nerves present; rudiments of hippocampus appear; basilar artery appears | (11, 28, 29) |
40 (11–14 mm) | 17 | olfactory nerve axons enter the brain; dura mater appears near tentorium; venous plexi prominent; chondrification in skull base; microscopic foci of ossification appear in calvarium; microglia present throughout CNS parenchyma | (21, 30–32) |
42 (13–17 mm) | 18 | rudiments of striatum appear; inferior cerebellar peduncles; leptomeninges begin to cavitate (precursor of subarachnoid space) | (21) |
44 | 19 | Cajal-Retzius neurons appear in marginal zone; choroid plexus appears in fourth ventricle | (11) |
47 | 20 | choroid plexus appears in lateral ventricles | (33) |
50 (22–24 mm) | 21 | cortical plate appears in cerebral hemispheres; pioneer projection neurons (calretinin immunoreactive) appear; arterial circle (of Willis) complete | (11, 34) |
52 | 22 | internal capsule axons emerge from cerebral hemispheres | |
56 (27–31 mm) | 23 | external germinal layer of cerebellum appears; motor and sensory axons well defined in rhombencephalon; cortical plate covers entire neopallium – ~250 µm thick in dorsal frontal region (including germinal layer); axonal growth cones present in marginal zone; cartilaginous vertebral column complete | (35–37) |
End of embryonic period |
Fetal fertilization age in weeks (crown-rump length) | Gestational age | Important milestones | References |
---|---|---|---|
9 weeks (40 mm) | 11 weeks | expansion of cerebral hemispheres in a curve to form the temporal lobes is associated with C-shape of caudate nucleus, choroid plexus, and hippocampal connections; pyramidal neurons apparent in hippocampus; flocculo-nodular lobes of cerebellum defined; ossification begins in skull base (sella turcica); arterial system resembles adult configuration | (31, 38–40) |
11 weeks | 13 weeks | massa commissularis develops above septal region (precursor to anterior corpus callosum); anterior commissure well developed; synaptophysin first detected in CNS (spinal cord) | (41–43) |
12 weeks (87 mm) | 14 weeks | corpus callosum earliest axon crossing; microglia begin to express MHC class II antigens; bones of calvarium extensively ossified but not fused (Figure 19.7) | (42, 44, 45) |
13 weeks | 15 weeks | cerebellar vermis develops early folia and fissures; synaptophysin in thalamus | (43, 46) |
15 weeks (130 mm) | 17 weeks | retina layers complete | (47) |
16 weeks (140 mm) | 18 weeks | corpus callosum reaches full rostro-caudal extent (Figure 19.8); synaptophysin in pons and cerebellum | (41, 43, 48) |
Figure 19.4 Drawing of a 2.4 mm human embryo (oblique, posterior view; Carnegie stage 10) with the amnion and yolk sac cut away to reveal the neural tube which has partially closed at the spinal cord level.
Figure 19.5 Drawing of a 3.2 mm human embryo (Carnegie stage 11) neural tube (lateral surface, A; and median sagittal plane section, B). The optic vesicles bulge from the telencephalic pallium, the anterior (rostral) neuropore has almost closed, and the cephalic flexure is pronounced immediately caudal to the mesencephalon.
Figure 19.6 Drawing of a 7 mm human embryo (Carnegie stage 15) neural tube (lateral surface, A; and median sagittal plane section, B). The optic cups form the future eyes, the pallial bulges form the future cerebral hemispheres, and a rudimentary cerebellar bulge is present.
Figure 19.7 Drawing of a 90 mm human fetus (14 weeks gestation) head with half the skull cut away to show the lateral aspect of the brain. The lobes of the cerebral hemispheres are clearly defined, the pontine bulge is prominent, and the cerebellum is smooth.
Figure 19.8 Drawings of the medial surfaces of fetal brains at approximately 11, 13, and 16 weeks gestation (80, 95, and 150 mm respectively) showing development of the commissural structures including the corpus callosum and anterior commissure, as well as the columns of the fornix (hippocampal connection to septal region and mamillary bodies).
References
Basic organization of the CNS is established during the embryonic and early fetal period (see Chapter 19). However, at this stage, the human brain is barely formed. From the 20th to the 40th week of gestation, brain weight increases tenfold. It doubles again during the first year of postnatal life, and continues to increase another 50% during the next 10 to 15 years when adult weight is finally reached (see Chapter 26) (1). Growth is accomplished through several processes. The quantity of brain cells increases at a brisk rate in the periventricular germinal matrix until approximately 30 weeks gestation and at a much lesser rate until approximately full-term gestation (2). The exception is the cerebellum, wherein the external granular zone continues to produce neurons until approximately 9 months postnatal age (see Chapter 22) (3). When cell proliferation declines and neuronal and glial precursors have migrated to reach their destinations, expansion of cells (in particular neurons) and myelination (see Chapter 21) (4) account for the increasing brain weight. Recent transcriptome analyses (i.e., identification of messenger RNA patterns) correlate with the decades of morphologic analyses. In the neocortex, neurogenesis signals are high in the fetus and drop to low levels around full-term gestation. Neuronal differentiation signals peak around birth and gradually decline into adolescence, while synaptogenesis signals peak in early childhood and persist at substantial levels into adulthood. Astrogliogenesis signals gradually climb in the late fetal period, peaking in late infancy and early childhood. Myelination signals begin in the late fetal period and rise throughout childhood to remain stable in adulthood (5, 6).
Germinal Matrix
The ventricular zone (VZ) and subventricular zone (SVZ) were introduced in Chapter 19. The ventricular zone (VZ) is a narrow layer (<10 cells thick) of proliferating cells and radial glia; it lies immediately adjacent to the cerebrospinal fluid channels (7, 8). The VZ gives rise to progenitors of the SVZ and to glutamatergic excitatory neurons beginning in the late embryonic period and continuing to ~18 weeks gestation; rare excitatory neurons appear to be generated as late as 28 weeks (8). The adjacent SVZ is mitotically active throughout the cerebrum until ~18 weeks gestation. Over the length of the future caudate nucleus, the SVZ becomes thickened, especially along the lateral walls of the frontal horns of the lateral ventricles. This is the ganglionic eminence, which produces precursor neurons of the basal nuclei, inhibitory interneurons of the cerebral cortex, a population of thalamic neurons, and glial progenitors (9). SVZ volume peaks at 23–25 weeks gestation (10). Mitotic activity remains prominent in the ganglionic eminence until approximately 28 weeks gestation, after which it declines in parallel with thinning of the SVZ, reaching low levels by 30–32 weeks (2) (Figures 20.1 and 20.2). Within the ganglionic eminence, the VZ can be delineated histologically from the SVZ because the VZ cells are slightly larger and less densely packed. As the VZ ceases to be proliferative, it disappears and is replaced by ciliated ependymal cells. This begins at about 27 weeks over the ganglionic eminence but is earlier along other parts of the ventricle wall. As the SVZ involutes, it becomes patchy. It remains as a slightly hypercellular but gradually thinning layer below the ependymal cells until about six months age (Figure 20.3 and 20.4). Small clusters of residual SVZ cells are often present in dispersed or perivascular clusters of the frontal white matter near the lateral ventricle, basal to the frontal horn, and within the white matter bundles of the striatum; these must not be mistaken for inflammatory cells (Figure 20.5). Rare multipotent SVZ cells persist into adulthood (11).
Figure 20.1 Whole mount photomicrographs showing coronal sections of human fetal brain at the level of the ganglionic eminence (GE). Upper row shows hematoxylin and eosin (H&E) stained slices and the lower row shows the Ki67 (proliferation marker) immunostained slices. At 15 weeks gestation, a prominent groove (arrow) divides the dorsal and ventral regions of the GE (corresponding to the lateral and medial ganglionic eminences referred to in the animal literature). The ventricular zone (VZ) along the roof of the lateral ventricle is highly proliferative and a moderate proportion of cells in the intermediate zone (IZ) are positive. At 20 weeks gestation, the GE remains thick, but only the ventral portion is highly proliferative (arrow). From 26 weeks to 36 weeks, the GE involutes and the proportion of Ki67-positive cells is reduced.
Figure 20.2 Upper scatter plot shows thickness of the GE (mm) as a function of the corrected post-menstrual age (weeks) in human fetuses. The thickness is maximal from 20–30 weeks. Lower scatter plot shows the proportion of GE cells immunoreactive for Ki67 in the ventral SVZ. Proliferation peaks from 20 to 26 weeks followed by a steep decline, corresponding to the thinning of the GE. After 34–35 weeks, a small number of cells continue to proliferate into the early post-natal period.
Figure 20.3 Photomicrographs showing histologic changes in the ventral ganglionic eminence over the normal fetal caudate nucleus head from 18 to 39 weeks gestation. The ventricular zone (VZ) lies between the subventricular zone (SVZ) and the ventricle (V). White arrows mark the VZ/SVZ interface. When the VZ ceases to be proliferative, it thins and is replaced by a simple columnar to cuboidal ciliated ependymal cell layer (red arrows). Up to 25 weeks the SVZ is densely cellular, but beginning at 27 weeks it thins and becomes patchy. At 34 to 39 weeks the SVZ between the ependymal layer and the caudate nucleus head (CN) is only slightly hypercellular and is minimally proliferative. All images hematoxylin and eosin stain; original magnification 200x.
Figure 20.4 Photomicrographs showing histologic changes in postnatal caudate nucleus (CN) head from 1 to 11 months age. The ciliated ependymal cell layer (red arrow) gradually changes from columnar to cuboidal. The slightly hypercellular SVZ between the ependymal layer and the caudate nucleus head (black arrows) becomes progressively less obvious. All images hematoxylin and eosin stain; original magnification 200x.
Figure 20.5 Clusters of residual subventricular zone cells in white matter near the frontal lateral ventricle. In a 4-month infant (left panel) the cells are clustered near the ventricle angle (v) and in the white matter (arrow; inset enlarged). In a 2 month infant (right panel), the cells are more dispersed (arrow). These must not be mistaken for inflammatory cells. Hematoxylin and eosin stain.
When examining fetal brains, one should be aware of the rostral migratory stream. This cluster of cells branches from the basal SVZ at the anterior-most limit of the frontal horn of the lateral ventricle and is directed toward the olfactory ventricle and olfactory tract. Migrating neuroblasts are abundant in the fetal brain and in early infancy, but are rare after six months of age (12–14).
Most neocortical GABAergic inhibitory interneurons come from the ventral ganglionic eminences (15). They are produced as late as 27–35 weeks gestation (16, 17). Neurons from the ganglionic eminence that are destined for the thalamus are generated to ~34 weeks gestation (18). They migrate along the ventricle wall through the gangliothalamic migratory pathway (19). The complexity of the SVZ is gradually being revealed by studies of human material. It is now evident that there are multiple precursor populations within the SVZ and that the human SVZ is both quantitively and qualitatively different from that of lower mammals (20–23).
Vascularization of the Cerebrum
Small blood vessels invade the telencephalon from the surrounding mesenchyme during weeks 5–7 of the embryonic period (24, 25) and continually expand and branch as the brain grows (26, 27). The blood-brain barrier (BBB), which is essential to maintain CNS homeostasis, is created by modified endothelial cells, which develop tight junctions and specialized transport mechanisms (28). During angiogenesis, neural progenitor cells release vascular endothelial growth factor (VEGF), Wnt, and other mediators to stimulate BBB induction. BBB sealing and maintenance is governed by the release of Shh from adjacent astrocytes and Ang-1 from pericytes (29). Pericytes surround endothelial cells, embedded within the basement membrane. Most seem to arise from myeloid/mesenchymal stem cells that accompany the growing blood vessels (30). In addition to their role in the BBB, pericytes help in the regulation of capillary blood flow to match local metabolic needs, exhibit phagocytic activity, and may function as pluripotent stem cells (31). Pericytes and smooth muscle cells have many immunohistochemical markers in common (32). Endothelial cells express tight junction proteins and transport proteins at 12 weeks gestation and adult-like patterns by 18–22 weeks (33, 34). Although the fetal BBB is more permeable than in adults, it remains to be determined if the fetal BBB is truly “immature” or if the differences are matched to the developmental needs and pressure circumstances of the fetal brain (35).
The density and diameter of blood vessels in the human brain increases rapidly between 26 and 35 weeks gestation (36), with the highest densities in the germinal regions (37). Veins in the periventricular germinal matrix are fragile because their walls are thin and the surrounding tissues offer minimal structural support (38). Smooth muscle accumulation around artery walls begins in deep cerebral structures at about 30 weeks gestation. Vascular maturation seems to progress outward toward the pial surface (39); this correlates with progressive improvement in cerebral blood flow autoregulation (40).
Growth of the Hippocampus
The hippocampus represents one of the most primitive parts of the cerebral hemispheres. It plays critical roles in the consolidation of short-term memory into long-term memory and in spatial navigation. Consequently, damage to the hippocampus can be associated with significant cognitive disability. The relative sensitivity of hippocampal neurons to hypoxic-ischemic insults makes it an invaluable structure for histopathologic evaluation of various types of brain damage. The hippocampus is situated in the temporal lobe, along the floor of the lateral ventricle. In coronal sections, its characteristic microanatomy consists of a layer of densely packed pyramidal neurons (cornu ammonis, abbreviated as CA), which curl in a U shape that spreads and merges with the temporal cortex in the subiculum. The other end of the “U” (the CA4 sector) is surrounded by the backward-facing U- to V-shaped dentate gyrus, which consists of small granular neurons (Figure 20.6).
Figure 20.6 Photograph showing microanatomy of the hippocampus from a 39-week gestation newborn. Large pyramidal neurons of the cornu ammonis (CA) are divided into the CA1, CA2, CA3, and CA4 sectors. The CA2 neurons tend to be more tightly clustered and are relatively resistant to hypoxic-ischemic damage in comparison to the other sectors. The CA4 sector is surrounded by the dentate gyrus (DG). At its terminal the CA1 neurons fan out and merge with the subiculum (sub). Axons in the alveus (alv) merge in the fimbria (fim) along the wall of the ventricle (v) and then pass through the fornix into the septal region of the brain. Hematoxylin and eosin stain; original magnification 40x.
Beginnings of the hippocampus appear in the embryo at Carnegie stage 16 (41), and the hippocampal plate is present at 9 weeks gestation (42, 43). Rudiments of the dentate gyrus appear at 14 weeks (42). Synapses appear in the hippocampus by 15 weeks gestation (44). The VZ disappears from the hippocampus by 20–22 weeks, by which time all hippocampal pyramidal neurons have been generated (45). Hippocampal neurons expand gradually during the fetal period (46). Several different glutamate receptors are present as early as 11 weeks gestation and increase progressively to 32 weeks (47). However, even at full term gestation, the neurons remain small and immature in appearance (especially in the CA1 sector) (Figures 20.7 and 20.8). The cytoarchitectonic features of the hippocampus are mature by two years of age (45, 48) but completely adult-like morphology is not present until the teenage years (49). Granule neurons of the dentate gyrus are generated with moderate frequency during the first postnatal year and at low frequency thereafter (42, 50). There is a debate about whether these neurons are generated after the teenage years (51–55).
Figure 20.7 Photomicrographs showing histologic changes in the hippocampus from 18 to 39 weeks gestation. At 18 weeks the dentate gyrus (DG) is obvious, but neurons of the cornu ammonis are small. By 27 weeks the relatively more compacted band of CA2 neurons can be distinguished (between arrows). All images hematoxylin and eosin stain; original magnification 40x.
Figure 20.8 Photomicrographs showing histologic changes in postnatal hippocampus from 0.5 to 11 months age. The CA2 sector (between arrows) is obviously compact up to 6 months, but as the neurons (and hippocampus overall) enlarge, they spread. All images hematoxylin and eosin stain; original magnification 40x.
Cytologic Growth of the Cerebral Hemispheres
In general, excitatory neurons with long projections migrate radially (along radial glia) from the VZ located along the ventricle wall. Later born neurons migrate to progressively more superficial layers, giving rise to the characteristic laminar pattern of the neocortex, which can be characterized using a range of antibody markers (56, 57). Neurons with connections to the brainstem and spinal cord lie mainly in cortical layer 5, neurons with long subcortical connections in layer 6, and neurons with cross callosal connections mainly in layers 2 and 3 (58). Glutamate receptors and transporters are expressed in the cortex beginning no later than 16–17 weeks gestation (59, 60). GABAergic inhibitory interneurons in the neocortex come from the ventral ganglionic eminences (15, 61) and are generated later than long projection neurons, typically from midgestation up to 27–35 weeks (16, 17). The development of various neurotransmitter systems has been well documented in the fetal brain (62). Immature neurons often express non-phosphorylated neurofilament, which is replaced by phosphorylated neurofilament in mature axons (63).
As the cortex matures during the fetal and postnatal period, individual neurons become increasingly larger and more dispersed, and the cortical lamination becomes more well defined (Figures 20.9–20.12). Note that fine details of cortical structure and timing of maturation differ slightly between different Brodmann areas (e.g., see (64)).
Figure 20.9 Photomicrographs showing histologic changes in the dorsal frontal cortex from 18 to 39 weeks (wk) gestation (pial surface to left). At 18 weeks, the cortical plate (CP) and subplate (SP) merge; the intermediate zone (IZ) is highly cellular with many streams of migrating cells. At 21 weeks, a radial columnar pattern is evident in the cortex. Large Cajal-Retzius neurons can be identified near the subpial surface (inset). At 24 weeks, layers 2 and 3 of the cortex remain densely packed with small cells (between arrows). At 25 weeks, layers 4 to 6 (between arrows) are more dispersed. At 28 weeks, only layer 2 remains densely cellular (arrow), and this is less so at 29 weeks. At 34 weeks, all of the cortical layers are obvious (1–6) and they continue to spread at 39 weeks. All images hematoxylin and eosin stain; original magnification 100x.
Figure 20.11 Photomicrographs showing histologic changes in layer 5 of the dorsal frontal cortex from 18 to 39 weeks (wk) gestation (pial surface at the top). At 18 weeks, the cells are all small; neurons cannot be distinguished with certainty. At 21 weeks a columnar organization becomes more obvious and rare neurons with larger nuclei and prominent nucleoli can be found (arrow). At 25 and 27 weeks, more definite neurons are identifiable (arrow). At 28 weeks, large pyramidal neurons are obvious as the neuropil expands (arrow). At 34 weeks, large neurons are often slightly clustered (arrow) but by 39 weeks the clusters have dispersed in the further expanded neuropil (arrow). All images hematoxylin and eosin stain; original magnification 400x.
Figure 20.12 Photomicrographs showing histologic changes in layer 5 of the dorsal frontal cortex from postnatal 0.5 months (mo) to 21 years (y). From 0.5 to 1.5 months individual pyramidal neurons have obviously enlarged (arrow) and the neuropil continues to expand at 11 months (*). After that stage, the largest neurons do not get much larger, but others continue to enlarge at 2.5. years (arrow). The neuropil does not reach adult bulk until about 10 years of age. All images hematoxylin and eosin stain; original magnification 400x.
The subplate zone is a transient neuronal region immediately subjacent to the developing cortex. It arises from VZ cells and forms during weeks 14–17 of gestation (65, 66). Subplate neurons are the earliest functional neurons in the fetal cortex and therefore one of the early sites of synaptophysin accumulation. From 21–25 weeks gestation, subplate neurons interact with thalamocortical axons and later serve as transient interneurons and guides for the earliest thalamocortical connections (67, 68). The subplate dissipates at about 35 weeks, concurrent with secondary gyrification (69). Large (interstitial) neurons in white matter are likely residual subplate cells (70, 71).
Astrogliogenesis has been studied in the human brain mainly using immunohistochemistry. From 9 to 11 weeks gestation, S100 immunoreactive cells distinct from radial glia and thought to represent astrocytes appear in the hippocampus and cerebrum (72, 73). Glial fibrillary acidic protein (GFAP) immunoreactive astroglial cells appear in the midbrain at 11–12 weeks gestation (74) and are rare in the neocortex at 14 weeks (62). However, by 15 weeks, “mature astrocytes” can be found throughout the CNS in low density (75, 76), and from 21 weeks onward they become increasingly more abundant (62, 77). Beginning in the second half of gestation, the ganglionic eminence SVZ generates an abundance of glial precursors for the cerebrum (78). Some vimentin immunoreactive radial glia might transform into stellate astrocytes during the third trimester (79). GFAP mRNA expression reaches persistently high levels in the hippocampus and neocortex around full-term gestation (80). The morphology and distribution of GFAP expressing cells changes in the postnatal brain. From term birth onward, the external glia limitans gradually becomes more prominent and thicker. In the normal cortex, GFAP positive cells are small with fine processes and largely confined to the perivascular regions (81).
White matter and oligodendroglial development are addressed in Chapter 21.
Gyrification of the Cerebral Hemispheres
As the brain grows and the cerebral cortex becomes thicker and broader, the cortical surface area expands quickly. To accommodate the large surface area within a head of reasonable size, evolutionary pressures have led to gyrification of the brain (82). Normal development of the cerebral sulci and gyri follows a set pattern and tempo. In combination with weight (see Chapter 26), gyral patterns allow the observer to make estimates of the gestational age of fetal brains. The temporal appearance of sulci and gyri has been reported in tabular form from 10 to 44 weeks gestation (83). Similar information has been published using photographs of brains in various orientations. These can be used as quick references at the time of brain examination (84, 85). Figures 20.13 to 20.15 show photographic examples of normal fetal brains. Figures 20.16 and 20.17 show diagrammatic representations of gyral development from 12 to 39 weeks gestation. Note that brain softening due to autolysis can blunt the details of gyri and sulci. Stripping of the leptomeninges may be necessary to evaluate the cortical surface if the vasculature is extremely congested.
Figure 20.13 Photographs showing the lateral and medial cerebral surfaces from fetuses 16 to 21 weeks gestational age. At 16 weeks the lateral surface is smooth, but the medial occipital region exhibits the calcarine fissure (arrow). At 18 weeks, the frontal and temporal lobes enlarge and expand laterally, thereby defining the insular cortex (arrows). At 21 weeks, irregularities due to the early formation of the central sulcus are apparent (arrow).
Figure 20.14 Photographs showing the lateral and medial cerebral surfaces from fetuses 22 to 28 weeks gestational age. At 22 weeks, the cingulate sulcus is present (arrow). At 24 weeks, the central sulcus is well defined (arrow). At 26 weeks, more frontal lobe sulci have developed (arrow). At 28 weeks, the superior temporal sulcus is well defined (arrow).
Figure 20.15 Photographs showing the lateral and medial cerebral surfaces from fetuses 32 to 40 weeks gestational age. At 32 weeks, the lateral surface of the temporal lobe remains somewhat simplified. The superior, middle, and inferior temporal gyri are apparent at 34 weeks. The gyral pattern becomes increasingly complex on the lateral and medial surfaces at 38 and 40 weeks, by which time the adult pattern (but not brain size) has been reached.
Figure 20.16 Diagrams showing major gyral features of the human brain in lateral, dorsal, basal, and coronal slice orientations from 12–26 weeks gestation. The bar represents 1 cm at each stage. Abbreviations: CC – corpus callosum; Cing – cingulum; Circ – circular sulcus; Coll – collateral fissure; Fs – frontal sulcus; GR – gyrus rectus; ip – intraparietal sulcus; Olf – olfactory tract; P-O – parietal-occipital groove; Parahip – parahippocampal sulcus; PreRol PostRol – pre and post-Rolandic sulci; Rol – Rolandic (central) sulcus; Sylv – Sylvian fissure; Ts – superior temporal sulcus.
One of the shortcomings of gyral development reports is that they are based upon somewhat subjective criteria using only a small number of optimal autopsy specimens. Recent magnetic resonance imaging studies of live fetuses have been used to analyze volumetric growth, gyral patterns (86, 87), segmental distinction of gray and white matter and subcortical structures (88), and development of major white matter tracts (89). Although also hampered by small sample sizes, they validate observations made using autopsy specimens with respect to the patterns of normal human brain development. Perhaps more importantly for the future evaluation of malformations and development after premature birth, imaging in combination with mathematical methods of averaging morphologic data is potentially capable of detecting subtle deviations from normal (90).
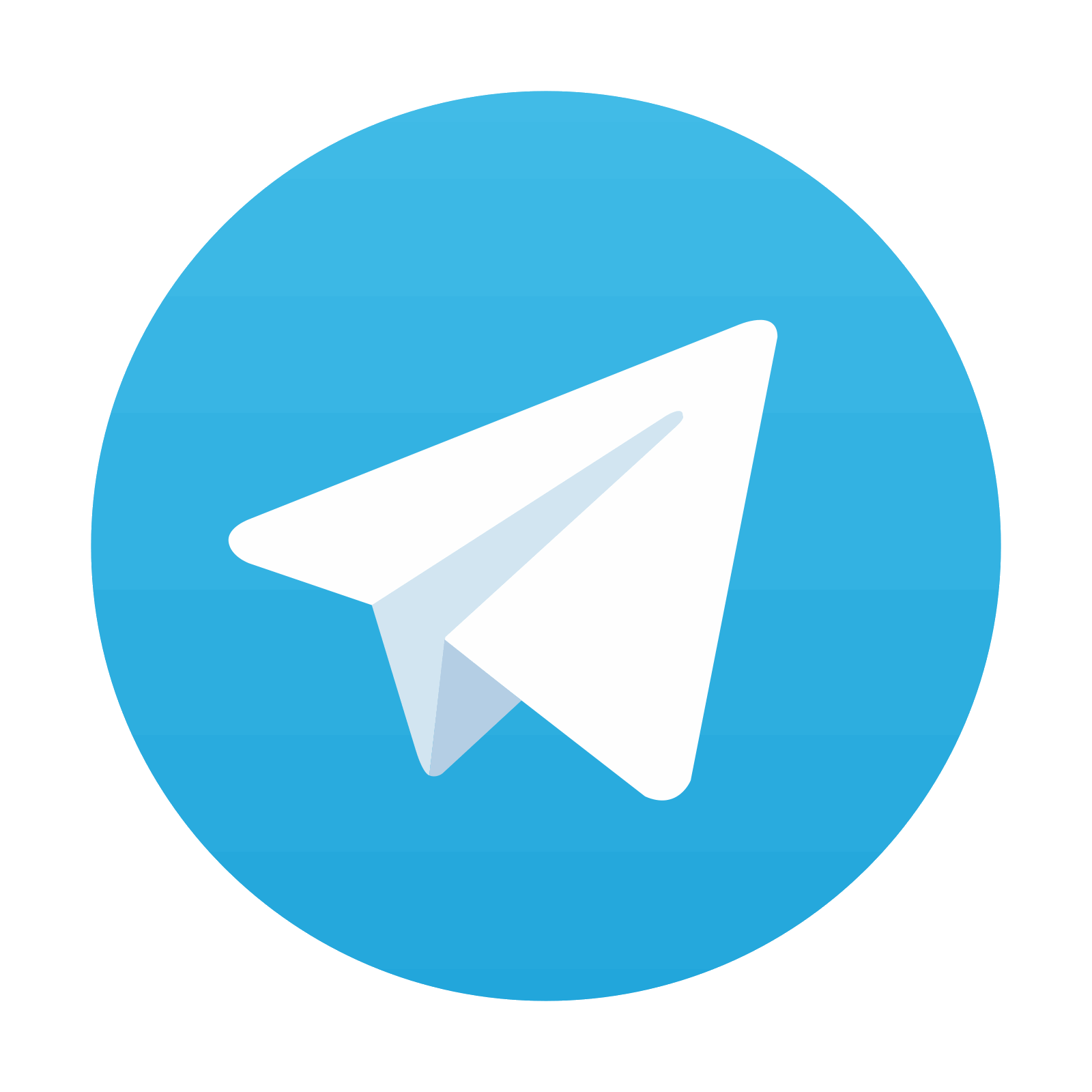
Stay updated, free articles. Join our Telegram channel
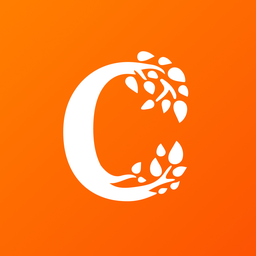
Full access? Get Clinical Tree
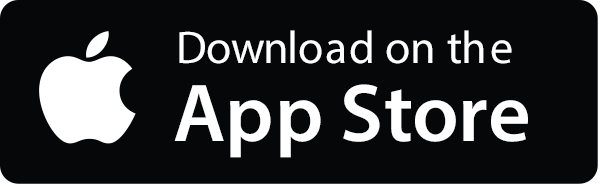
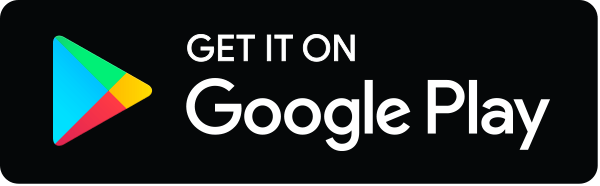