The nervous system consists of three main regions:
- •
The central nervous system (CNS) consists of the brain and spinal cord and is protected by the cranium and vertebral column.
- •
The peripheral nervous system (PNS) includes the neurons outside the CNS as well as the cranial nerves and spinal nerves (and their associated ganglia), which connect the brain and spinal cord with peripheral structures.
- •
The autonomic nervous system (ANS) has parts in the CNS and PNS and consists of the neurons that innervate smooth muscle, cardiac muscle, glandular epithelium, and combinations of these tissues.
Development of Nervous System
The first indications of the developing nervous system appear during the third week as the neural plate and neural groove develop on the posterior aspect of the trilaminar embryo ( Fig. 17.1 A ). The notochord and paraxial mesenchyme induce the overlying ectoderm to differentiate into the neural plate. This transformation (neural induction) involves intercellular signaling molecules, such as members of the transforming growth factor-β family, Wnts, sonic hedgehog (SHH), and bone morphogenic proteins (BMPs) . Formation of the neural folds, neural crest, and neural tube is illustrated in Figs. 17.1 B to F and 17.2 .
- •
The neural tube differentiates into the CNS.
- •
The neural crest gives rise to cells that form most of the PNS and ANS.


Neurulation (formation of the neural plate and neural tube) begins during the fourth week (22 to 23 days) in the region of the fourth to sixth pairs of somites (see Fig. 17.1 C and D ). At this stage, the cranial two thirds of the neural plate and tube as far caudal as the fourth pair of somites represent the future brain, and the caudal one third of the plate and tube represents the future spinal cord.
Fusion of the neural folds and formation of the neural tube begins at the fifth somite and proceeds at multiple locations until only small areas of the tube remain open at both ends ( Fig. 17.3 A and B ). The lumen of the neural tube becomes the neural canal, which communicates freely with the amniotic cavity (see Fig. 17.3 C ). The cranial opening (rostral neuropore) closes at approximately the 25th day, and the caudal neuropore closes at approximately the 27th day (see Fig. 17.3 D ).

Closure of the neuropores coincides with the establishment of a vascular circulation for the neural tube. Syndecan 4 (SDC4) and van gogh–like 2 (VANGL2) proteins appear to be involved with neural tube closure. The neuroprogenitor cells of the wall of the neural tube thicken to form the brain and spinal cord ( Fig. 17.4 ). The neural canal forms the ventricular system of the brain and the central canal of the spinal cord .
The current hypothesis is that there are multiple (possibly five) closure sites involved in the formation of the neural tube. Failure of closure of site 1 results in spina bifida cystica (see Fig. 17.15 ). Meroencephaly (anencephaly) results from failure of closure of site 2 (see Fig. 17.13 ). Craniorachischisis results from failure of sites 2, 4, and 1 to close. Site 3 nonfusion is rare.
The neural tube defects (NTDs) are described later (see Fig. 17.17 ). It has been suggested that the most caudal region may have a fifth closure site from the second lumbar vertebra to the second sacral vertebra and that closure inferior to the second sacral vertebra occurs by secondary neurulation. Epidemiologic analysis of neonates with NTD supports the concept that there are multiple closures of the neural tube in humans.

Development of Spinal Cord
The primordial spinal cord develops from the caudal part of the neural plate and caudal eminence. The neural tube caudal to the fourth pair of somites develops into the spinal cord ( Fig. 17.5 ; see Figs. 17.3 and 17.4 ). The lateral walls of the neural tube thicken, gradually reducing the size of the neural canal until only a minute central canal of the spinal cord exists at 9 to 10 weeks (see Fig. 17.5 C ). Retinoic acid signaling is essential in the development of the spinal cord from early patterning to neurogenesis.

Initially, the wall of the neural tube is composed of a thick, pseudostratified, columnar neuroepithelium (see Fig. 17.5 D ). These neuroepithelial cells constitute the ventricular zone (ependymal layer), which gives rise to all neurons and macroglial cells (macroglia) in the spinal cord ( Fig. 17.6 ; see Fig. 17.5 E ). Macroglial cells are the larger members of the neuroglial family of cells, which includes astrocytes and oligodendrocytes. Soon, a marginal zone composed of the outer parts of the neuroepithelial cells becomes recognizable (see Fig. 17.5 E ). This zone gradually becomes the white matter of the spinal cord as axons grow into it from nerve cell bodies in the spinal cord, spinal ganglia, and brain.

Some dividing neuroepithelial cells in the ventricular zone differentiate into primordial neurons (neuroblasts). These embryonic cells form an intermediate zone (mantle layer) between the ventricular and marginal zones. Neuroblasts become neurons as they develop cytoplasmic processes (see Fig. 17.6 ).
The supporting cells of the CNS, called glioblasts (spongioblasts), differentiate from neuroepithelial progenitor stem cells, mainly after neuroblast formation has ceased. The glioblasts migrate from the ventricular zone into the intermediate and marginal zones. Some glioblasts become astroblasts and later astrocytes , whereas others (oligodendrocyte progenitor cells) become oligodendroblasts and eventually oligodendrocytes (see Fig. 17.6 ). When the neuroepithelial cells cease producing neuroblasts and glioblasts, they differentiate into ependymal cells, which form the ependyma (ependymal epithelium) lining the central canal of the spinal cord. SHH and Olig2 basic-helix-loop-helix signaling controls the proliferation, survival, and patterning of neuroepithelial progenitor cells by regulating GLI transcription factors (see Fig. 17.2 ).
Microglia (microglial cells), which are scattered throughout the gray and white matter of the spinal cord, are small cells that are derived from mesenchymal cells (see Fig. 17.6 ). Microglia invade the CNS rather late in the fetal period after it has been penetrated by blood vessels. Microglia originate in the bone marrow and are part of the mononuclear phagocytic cell population.
Proliferation and differentiation of neuroepithelial cells in the developing spinal cord produce thick walls and thin roof plates and floor plates (see Fig. 17.5 B ). Differential thickening of the lateral walls of the spinal cord soon produces a shallow longitudinal groove on each side, the sulcus limitans ( Fig. 17.7 ; see Fig. 17.5 B ). This groove separates the dorsal part (alar plate) from the ventral part (basal plate) . The alar and basal plates produce longitudinal bulges extending through most of the length of the developing spinal cord. This regional separation is of fundamental importance because the alar and basal plates are later associated with afferent and efferent functions, respectively.

Cell bodies in the alar plates form the dorsal gray columns , which extend the length of the spinal cord. In transverse sections of the cord, these columns are the dorsal gray horns (see Fig. 17.7 ). Neurons in these columns constitute afferent nuclei, and groups of them form the dorsal gray columns. As the alar plates enlarge, the dorsal median septum forms. Cell bodies in the basal plates form the ventral and lateral gray columns.
In transverse sections of the spinal cord, these columns are the ventral gray horns and lateral gray horns , respectively (see Fig. 17.5 C ). Axons of ventral horn cells grow out of the spinal cord and form the ventral roots of the spinal nerves . As the basal plates enlarge, they bulge ventrally on each side of the median plane. As this occurs, the ventral median septum forms, and a deep longitudinal groove (ventral median fissure) develops on the ventral surface of the spinal cord (see Fig. 17.5 C ).
Development of Spinal Ganglia
The unipolar neurons in the spinal ganglia (dorsal root ganglia) are derived from neural crest cells ( Figs. 17.8 and 17.9 ). The axons of cells in the spinal ganglia are at first bipolar, but the two processes soon unite in a T -shaped fashion. Both processes of spinal ganglion cells have the structural characteristics of axons , but the peripheral process is a dendrite in that there is conduction toward the cell body. The peripheral processes of spinal ganglion cells pass in the spinal nerves to sensory endings in somatic or visceral structures (see Fig. 17.8 ). The central processes enter the spinal cord and constitute the dorsal roots of spinal nerves .


Development of Spinal Meninges
The meninges (membranes covering the spinal cord) develop from cells of the neural crest and mesenchyme between 20 and 35 days. The cells migrate to surround the neural tube (primordium of the brain and spinal cord) and form the primordial meninges (see Fig. 17.1 F ).
The external layer of these membranes thickens to form the dura mater ( Fig. 17.10 A and B ), and the internal layer, the pia arachnoid, is composed of pia mater and arachnoid mater (leptomeninges) . Fluid-filled spaces appear within the leptomeninges that soon coalesce to form the subarachnoid space (see Fig. 17.12 A ). The origin of the pia mater and arachnoid from a single layer is indicated in the adult by arachnoid trabeculae, which are numerous, delicate strands of connective tissue that pass between the pia and arachnoid. Cerebrospinal fluid (CSF) begins to form during the fifth week (see Fig. 17.12 A ).

Positional Changes of Spinal Cord
The spinal cord in the embryo extends the entire length of the vertebral canal (see Fig. 17.10 A ). The spinal nerves pass through the intervertebral foramina opposite their levels of origin. Because the vertebral column and dura mater grow more rapidly than the spinal cord, this positional relationship of the spinal nerves does not persist. The caudal end of the spinal cord in fetuses gradually comes to lie at relatively higher levels. In a 24-week-old fetus, it lies at the level of the first sacral vertebra (see Fig. 17.10 B ).
The spinal cord in neonates terminates at the level of the second or third lumbar vertebra (see Fig. 17.10 C ). In adults, the cord usually terminates at the inferior border of the first lumbar vertebra (see Fig. 17.10 D ). This is an average level because the caudal end of the spinal cord in adults may be as superior as the 12th thoracic vertebra or as inferior as the third lumbar vertebra. The spinal nerve roots , especially those of the lumbar and sacral segments, run obliquely from the spinal cord to the corresponding level of the vertebral column (see Fig. 17.10 D ). The nerve roots inferior to the end of the cord (medullary cone) form a bundle of spinal nerve roots called the cauda equina (Latin, “horsetail”), which arises from the lumbosacral enlargement (swelling) and medullary cone of the spinal cord (see Fig. 17.10 D ).
Although the dura mater and arachnoid mater usually end at the S2 vertebra in adults, the pia mater does not. Distal to the caudal end of the spinal cord, the pia mater forms a long fibrous thread, the filum terminale (terminal filum), which indicates the original level of the caudal end of the embryonic spinal cord (see Fig. 17.10 C ). The filum (Latin, “thread”) extends from the medullary cone and attaches to the periosteum of the first coccygeal vertebra (see Fig. 17.10 D ).
Myelination of Nerve Fibers
Myelin sheaths around the nerve fibers within the spinal cord begin to form during the late fetal period and continue to form during the first postnatal year ( Fig. 17.11 E ). Myelin basic proteins, a family of related polypeptide isoforms, are essential in myelination; β 1 -integrins regulate this process. Fiber tracts become functional at approximately the time they become myelinated. Motor roots are myelinated before sensory roots. The myelin sheaths around the nerve fibers in the spinal cord are formed by oligodendrocytes (oligodendroglial cells), which are types of glial cells that originate from the neuroepithelium. The plasma membranes of these cells wrap around the axon, forming several layers (see Fig. 17.11 F to H ). Profilin 1 (PFN1) protein is essential in the microfilament polymerization that promotes changes to the oligodendrocyte cytoskeleton.

The myelin sheaths around the axons of peripheral nerve fibers are formed by the plasma membranes of the neurilemma (sheath of Schwann cells), which are analogous to oligodendrocytes. Neurilemma cells are derived from neural crest cells that migrate peripherally and wrap themselves around the axons of somatic motor neurons and preganglionic autonomic motor neurons as they pass out of the CNS (see Figs. 17.8 and 17.11 A to E ). These cells also wrap themselves around the central and peripheral processes of somatic and visceral sensory neurons and around the axons of postsynaptic autonomic motor neurons. Beginning at approximately 20 weeks, peripheral nerve fibers have a whitish appearance resulting from the deposition of myelin (layers of lipid and protein substances).
Most defects result from failure of fusion of one or more neural arches of the developing vertebrae during the fourth week. NTDs affect the tissues overlying the spinal cord: meninges, neural arches, muscles, and skin ( Fig. 17.12 ). Defects involving the embryonic neural arches are referred to as spina bifida ; subtypes of this defect are based on the degree and pattern of the NTD. The term spina bifida denotes nonfusion of the halves of the embryonic neural arches , which is common to all types of spina bifida (see Fig. 17.12 A ). Severe defects also involve the spinal cord, meninges, and neurocranium (bones of cranium enclosing the brain; Fig. 17.13 ). Spina bifida ranges from clinically significant types to minor defects that are functionally unimportant ( Fig. 17.14 ). Recent advances in fetal surgery have led to some hope for successful closure of these defects in utero, allowing for some improvement in neurologic outcomes.
A dermal sinus is lined with epidermis and skin appendages extending from the skin to a deeper-lying structure, usually the spinal cord. The sinus (channel) is associated with closure of the neural tube and formation of the meninges in the lumbosacral region of the spinal cord. The birth defect is caused by failure of the surface ectoderm (future skin) to detach from the neuroectoderm and meninges that envelop it. As a result, the meninges are continuous with a narrow channel that extends to a dimple in the skin of the sacral region of the back (see Fig. 17.13 ). The dimple indicates the region of closure of the caudal neuropore at the end of the fourth week and therefore represents the last place of separation between the surface ectoderm and the neural tube.
Spina bifida occulta is an NTD resulting from failure of the halves of one or more neural arches to fuse in the median plane (see Fig. 17.12 A ). This NTD occurs in the L5 or S1 vertebra in approximately 10% of otherwise normal people. In the minor form, the only evidence of its presence may be a small dimple with a tuft of hair arising from it (see Figs. 17.12 A and 17.14 ). The cause of the hypertrichosis (excessive hair) has not been established. An overlying lipoma dermal sinus or other birthmark may also occur. Spina bifida occulta usually produces no symptoms. A few affected infants have functionally significant defects of the underlying spinal cord and dorsal roots.
Severe types of spina bifida, which involve protrusion of the spinal cord and/or meninges through defects in the vertebral arches, are referred to collectively as spina bifida cystica because of the meningeal cyst that is associated with these defects ( Fig. 17.15 ; see Fig. 17.12 B to D ). This NTD occurs in approximately 1 of 5000 births and shows considerable geographic variation in incidence. When the cyst contains meninges and CSF, the defect is spinal bifida with meningocele (see Fig. 17.12 B ). The spinal cord and spinal roots are in the normal position, but there may be spinal cord defects. Protrusion of the meninges and CSF of the spinal cord occurs through a defect in the vertebral column.
If the spinal cord or nerve roots are contained within the meningeal cyst, the defect is spina bifida with meningomyelocele (see Figs. 17.12 C and 17.15 A ). Severe cases involving several vertebrae are associated with the absence of the calvaria (skullcap), absence of most of the brain, and facial abnormalities; these severe defects are called meroencephaly (see Figs. 17.13 and 17.17 ). The defects entail drastic effects in some brain areas and lesser or no effects in others. For these neonates, death is inevitable. The term anencephaly for these severe defects is inappropriate because the term falsely implies that no part of the brain exists.
Spina bifida cystica shows various degrees of neurologic deficit, depending on the position and extent of the lesion. Dermatomal loss of sensation along with complete or partial skeletal muscle paralysis occurs with the lesion (see Fig. 17.15 B ). The level of the lesion determines the area of anesthesia (area of skin without sensation) and the muscles affected. Sphincter paralysis (bladder or anal sphincters) is common with lumbosacral meningomyelocele (see Figs. 17.12 C and 17.15 A ). A saddle block anesthesia typically occurs when the sphincters are involved; loss of sensation occurs in the body region that would contact a saddle.
Meroencephaly is strongly suspected in utero when there is a high level of alpha-fetoprotein (AFP) in the amniotic fluid (see Chapter 6 , box titled “Alpha-Fetoprotein and Fetal Anomalies”). The level of AFP may also be elevated in maternal blood serum. Amniocentesis is usually performed on pregnant women with high levels of serum AFP for the determination of the AFP level in the amniotic fluid (see Chapter 6 , Fig. 6.13 ). An ultrasound scan may reveal an NTD that has resulted in spina bifida cystica. The fetal vertebral column can be detected by ultrasound at 10 to 12 weeks, and if there is a defect in the vertebral arch, a meningeal cyst may be detected in the affected area (see Figs. 17.12 C and 17.15 A ).
Meningomyelocele is a more common and a more severe defect than spina bifida with meningocele (see Figs. 17.15 A and 17.12 B ). This NTD may occur anywhere along the vertebral column; however, they are most common in the lumbar and sacral region (see Fig. 17.17 ). More than 90% of cases have associated hydrocephalus due to coexistence of an Arnold–Chiari malformation . Most patients require surgical diversion of CSF to avoid high intracranial pressure–related complications. Some cases of meningomyelocele are associated with craniolacunia (defective development of the calvaria), which results in depressed, nonossified areas on the inner surfaces of the flat bones of the calvaria.
Myeloschisis is the most severe type of spina bifida ( Fig. 17.16 ; see Figs. 17.12 D and 17.15 B ). In this defect, the spinal cord in the affected area is open because the neural folds and overlying skin failed to fuse. As a result, the spinal cord is represented by a flattened mass of exposed nervous tissue. Myeloschisis usually results in permanent paralysis or weakness of the lower limbs.
Nutritional and environmental factors undoubtedly play a role in the production of NTDs. Gene–gene and gene–environment interactions are likely involved in most cases. Food fortification with folic acid and folic acid supplements taken before conception and continued for at least 3 months during pregnancy reduce the incidence of NTDs. In 2015, the Centers for Disease Control and Prevention urged “all women of childbearing age who can become pregnant to get 0.4 mg of folic acid every day to help reduce the risk of neural tube defects” (for more information, go to http://www.cdc.gov/folicacid ). Epidemiologic studies have also shown that low maternal B 12 levels may significantly increase the risk of NTDs. Certain drugs (e.g., valproic acid) increase the risk of meningomyelocele. This anticonvulsant drug causes NTDs in 1% to 2% of pregnancies if taken during early pregnancy, when the neural folds are fusing ( Fig. 17.17 ).
Development of Brain






The brain begins to develop during the third week, when the neural plate and tube are developing from the neuroectoderm (see Fig. 17.1 ). The neural tube , cranial to the fourth pair of somites, develops into the brain. Neuroprogenitor cells proliferate, migrate, and differentiate to form specific areas of the brain. Fusion of the neural folds in the cranial region and closure of the rostral neuropore form three primary brain vesicles from which the brain develops ( Fig. 17.18 ):
- •
Forebrain (prosencephalon)
- •
Midbrain (mesencephalon)
- •
Hindbrain (rhombencephalon)

During the fifth week, the forebrain partly divides into two secondary brain vesicles , the telencephalon and diencephalon ; the midbrain does not divide. The hindbrain partly divides into two vesicles, the metencephalon and myelencephalon . Consequently, there are five secondary brain vesicles.
Brain Flexures
16
During the fifth week, the embryonic brain grows rapidly and bends ventrally with the head fold. The bending produces the midbrain flexure in the midbrain region and the cervical flexure at the junction of the hindbrain and spinal cord ( Fig. 17.19 A ). Later, unequal growth of the brain between these flexures produces the pontine flexure in the opposite direction. This flexure results in thinning of the roof of the hindbrain (see Fig. 17.19 C ). Early in development, a constriction-isthmic organizer is formed between the midbrain and hindbrain. Apparently, it functions as a signaling center. Wnt and Fgl signaling, which occur in this region, have been implicated in the patterning of the adjoining midbrain and hindbrain.

Initially, the primordial brain has the same basic structure as the developing spinal cord; however, the brain flexures produce considerable variation in the outline of transverse sections at different levels of the brain and in the position of the gray and white matter. The sulcus limitans extends cranially to the junction of the midbrain and forebrain, and the alar and basal plates are recognizable only in the midbrain and hindbrain (see Figs. 17.5 C and 17.19 C ).
Hindbrain
The cervical flexure demarcates the hindbrain from the spinal cord (see Fig. 17.19 A ). Later, this junction is arbitrarily defined as the level of the superior rootlet of the first cervical nerve, which is located roughly at the foramen magnum. The pontine flexure, located in the future pontine region, divides the hindbrain into caudal (myelencephalon) and rostral (metencephalon) parts. The myelencephalon becomes the medulla oblongata (commonly called the medulla ), and the metencephalon becomes the pons and cerebellum. The cavity of the hindbrain becomes the fourth ventricle and the central canal in the medulla (see Fig. 17.19 B and C ).
Myelencephalon
The caudal part of the myelencephalon (closed part of the medulla) resembles the spinal cord developmentally and structurally (see Fig. 17.19 B ). The neural canal of the neural tube forms the small central canal of the myelencephalon. Unlike those of the spinal cord, neuroblasts from the alar plates in the myelencephalon migrate into the marginal zone and form isolated areas of gray matter: the gracile nuclei medially and the cuneate nuclei laterally (see Fig. 17.19 B ). These nuclei are associated with correspondingly named nerve tracts that enter the medulla from the spinal cord. The ventral area of the medulla contains a pair of fiber bundles (pyramids) that consist of corticospinal fibers descending from the developing cerebral cortex (see Fig. 17.19 B ).
The rostral part of the myelencephalon (open part of the medulla) is wide and rather flat, especially opposite the pontine flexure (see Fig. 17.19 C and D ). The pontine flexure causes the lateral walls of the medulla to move laterally like the pages of an open book. As a result, its roof plate is stretched and greatly thinned (see Fig. 17.19 C ). The cavity of this part of the myelencephalon (part of the future fourth ventricle) becomes somewhat rhomboidal (diamond shaped). As the walls of the medulla move laterally, the alar plates become lateral to the basal plates. As the positions of the plates change, the motor nuclei usually develop medial to the sensory nuclei (see Fig. 17.19 C ).
Neuroblasts in the basal plates of the medulla , like those in the spinal cord, develop into motor neurons. The neuroblasts form nuclei (groups of nerve cells) and organize into three cell columns on each side (see Fig. 17.19 D ). From medial to lateral, the columns are named as follows:
- •
General somatic efferent , represented by neurons of the hypoglossal nerve
- •
Special visceral efferent , represented by neurons innervating muscles derived from the pharyngeal arches (see Chapter 9 , Fig. 9.6 )
- •
General visceral efferent , represented by some neurons of the vagus and glossopharyngeal nerves (see Chapter 9 , Fig. 9.6 )
Neuroblasts in the alar plates of the medulla form neurons that are arranged in four columns on each side. From medial to lateral, the columns are designated as follows:
- •
General visceral afferent , which receives impulses from the viscera
- •
Special visceral afferent , which receives taste fibers
- •
General somatic afferent , which receives impulses from the surface of the head
- •
Special somatic afferent , which receives impulses from the ear
Some neuroblasts from the alar plates migrate ventrally and form the neurons in the olivary nuclei (see Fig. 17.19 C and D ).
Metencephalon
The walls of the metencephalon form the pons and cerebellum , and the cavity of the metencephalon forms the superior part of the fourth ventricle ( Fig. 17.20 A ). As in the rostral part of the myelencephalon, the pontine flexure causes divergence of the lateral walls of the pons , which spreads the gray matter in the floor of the fourth ventricle (see Fig. 17.20 B ). As in the myelencephalon, neuroblasts in each basal plate develop into motor nuclei and organize into three columns on each side.
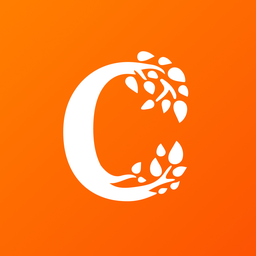
Full access? Get Clinical Tree
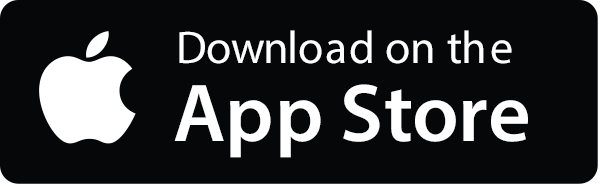
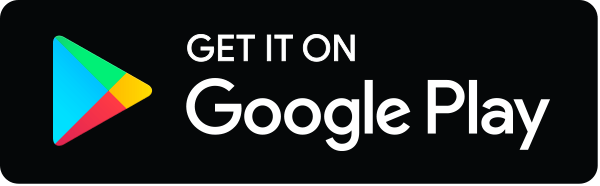