-
Chapter Contents
-
Part 1: Immunodeficiency 994
-
Introduction 994
-
The immune system 994
-
The development of the immune system 998
-
The maternofetal immunological relationship 999
-
Immune development and the neonatal gut 1000
-
Immunodeficiency disorders: general principles 1001
-
Classification and genetics 1001
-
-
Diagnosis and investigation of immunodeficiency 1001
-
Specific primary immunodeficiencies 1004
-
DNA repair defects and immunodeficiency 1007
-
Immunoregulation disorders 1007
-
Defects of lymphocyte apoptosis 1007
-
-
Other immunodeficiencies 1008
-
Defects of antibody production 1008
-
Disorders of phagocytic cells 1008
-
Treatment of immunodeficiency 1010
-
Vaccination of the child with a potentially impaired immune response 1010
-
Supportive care 1010
-
Specific measures for prevention of infections 1010
-
Treatment of infections 1010
-
Replacement immunoglobulin 1010
-
Granulocyte colony-stimulating factor 1011
-
Blood product support 1011
-
Haematopoietic stem cell transplantation 1011
-
-
-
Part 2: Infection in the newborn 1013
-
The origins of nosocomial infection related to feeding practices 1013
-
Neonatal skin and infection 1014
-
Incidence of infection and causative organisms 1015
-
Clinical presentation, investigation and management of neonatal sepsis 1021
-
Treatment of neonatal sepsis 1024
-
Treatment of fungal infection 1027
-
Fungal prophylaxis 1027
-
-
Treatment of ‘very sick septic neonates’ 1027
-
Additional (adjuvant) treatments for infection 1028
-
Prevention of infection – infection control 1029
-
Care bundles in the reduction of nosocomial infection 1030
-
Focal bacterial infections in the newborn 1030
-
Specific neonatal lung infections 1033
-
Superficial infections 1037
-
Other neonatal bacterial infections 1038
-
Perinatal viral infections 1042
-
Parasitic infections 1047
-
Non-bacterial congenital infections 1049
-
Congenital infections in the developing world 1052
-
Other congenital infections 1052
-
Immunodeficiency
- Andrew J Cant
- Andrew R Gennery
Introduction
The immune system distinguishes ‘self’ from ‘non-self’, destroying microorganisms but leaving other proteins intact. Immunological abnormalities result in an increased susceptibility to infection, as well as allergy and autoimmunity. The term baby’s immune system will generally respond appropriately, but failure may occur because of naïvety to an antigen or lack of fine-tuning (e.g. the antibody response to polysaccharide antigen). The preterm infant is immature in several respects, and is often subject to breaches of protective barriers by intravenous catheters and endotracheal tubes.
The immune system
Every effort should be made to exclude pathogens, particularly from the neonatal intensive care unit environment. The importance of hand-washing and high standards of hygiene cannot be overemphasised. The baby’s epithelial surfaces and mucous membranes provide the initial defence, augmented by cilia and respiratory tract mucus secretions, stomach acids and other mucous membrane secretions. Commensal intestinal and skin flora produce locally acting antibiotics. These barriers are particularly important in neonates, whose immunological immaturity predisposes them to infections.
Specific and non-specific mechanisms of immunity
Pathogens that breach the barrier defences first encounter non-specific and then specific components of the immune system. These are interdependent; many specific immune mechanisms use non-specific elements to exert their effects, and factors produced by the specific immune response can greatly potentiate non-specific functions.
Phagocytes such as neutrophils or monocytes, as well as the complement system, deliver highly effective non-antigen-specific immune responses using a limited number of pattern recognition receptors specific for conserved microbial structures, including Toll-like receptors and nucleotide-binding olgomerisation domain (NOD)-like receptors.
The specific immune system responds to antigens by proliferation of antigen-specific T and B lymphocytes following antigen presentation to their receptor by cells of the non-specific (innate) system. These responses are then committed to ‘immunological memory’, resulting in more rapid and enhanced responses upon subsequent antigen exposure. The differences between the innate and adaptive immune systems are summarised in Table 39.1 .
INNATE IMMUNITY | ACQUIRED (ADAPTIVE) IMMUNITY | |
---|---|---|
Cellular elements | Cellular: Phagocytes Neutrophils Basophils Eosinophils Macrophages Natural killer cells | T lymphocytes CD4+ helper T cells T H 1 cells T H 2 cells CD8+ cytotoxic T cells CD19+ B lymphocytes Plasma cells – secrete immunoglobulin |
Humoral elements | Chemical: Complement Mannose-binding lectin C-reactive protein Cytokines | Immunoglobulin (Ig)3 IgM IgA IgG IgE Cytokines |
Response to infection | Rapid: 0–4 hours | Delayed. Slow at first encounter (>96 hours) |
Receptor characteristics | Germline encoded | Encoded in multiple gene segments requiring gene rearrangement |
Expressed by all cells of a particular type | Clonal distribution | |
Broad specificity | Antigen specificity | |
Memory | Common to species, static | Specific to individual |
Updatable |
Non-specific immune mechanisms
Humoral
Complement is the major humoral component of the innate response. When the central component, C3b, which can be activated by at least three pathways, is bound to an antigen, it interacts powerfully with C3b receptors on neutrophils and monocytes, enhancing phagocytosis. C3b also initiates a cascade activating further components such as C5–C9, which lyse cell membranes, a mechanism particularly important in the handling of systemic neisserial infections.
Mannan-binding lectin, which binds to mannose on the surface of microorganisms, activates the classical complement pathway; this is important in the early stages of infection before antibody production, particularly in young children whose antibody-producing ability is immature.
Alpha- and beta-interferon (IFN-α, IFN-β) proteins are produced by virally infected cells. They render other cells immune to virus infection by producing an antiviral state. They also increase natural killer (NK) cell activity and increase human leukocyte antigen (HLA) class I antigen expression on cells. Gamma-interferon (IFN-γ), though having some antiviral effect, upregulates immune responses by enhancing intracellular killing of microorganisms such as mycobacteria.
Many bacteria require iron for growth, and decreasing iron availability is one mechanism of defence used by the host. An avid iron-binding protein, lactoferrin, present in human milk, reduces the growth of Escherichia coli . The removal of serum iron, which occurs during infections, increases the bacteriostatic effect of serum.
Lysozyme is found in neutrophil lysosomes and body secretions, including tears, saliva, and at increased concentrations on newborn skin, and has antibacterial properties.
Cellular
Phagocytic cells such as neutrophils and monocyte/macrophages migrate to the site of infection attracted by products of the acute inflammatory response (chemotaxis), including cleaved complement fragments. Adherence to the bacterium and ingestion (phagocytosis) follow, aided by immunoglobulin G (IgG) and complement (C3b), which stick to bacteria and phagocyte cell surface receptors Fcg and CR1/3, respectively (opsonisation). Following phagocytosis, lysosomes fuse with the bacterium-containing phagosome and release lysosomal enzymes (e.g. myeloperoxidase) and free oxygen radicals, which kill and digest microorganisms. Neutrophil numbers and function are enhanced by the inflammatory response.
Monocytes and macrophages kill extracellular bacteria more slowly and less efficiently than neutrophils but are important in defences against intracellular microorganisms, such as Mycobacterium tuberculosis and Listeria monocytogenes , because their activity is greatly enhanced by cytokines, particularly IFN-γ, produced by T lymphocytes.
NK cells are part of the non-T, non-B-cell lymphoid population and recognise and kill tumour and virus-infected cells.
Specific immune mechanisms
Specific responses are generated by T and B lymphocytes, whose receptors are antigen-specific. Binding of antigen to receptor initiates antibody production or the generation of specific cytotoxic T lymphocytes ( Fig. 39.1 ).

Antigen-presenting cells (APC) present antigens to T lymphocytes, degrade them to small peptide fragments and then express them on the cell surface in association with the major histocompatibility complex (MHC) molecules. The T-lymphocyte receptor (T-cell receptor, TCR) recognises the peptide in association with a self MHC molecule. CD4+ helper T lymphocytes respond to antigen bound within the groove of class II MHC molecules (HLA-DR) whilst CD8+ cytotoxic T lymphocytes respond to antigen bound within the groove of class I (HLA-AB) molecules. There are two arms of the specific immune system – antibody-mediated and cell-mediated.
Antibodies
These are produced by B lymphocytes and their derivatives, plasma cells, in response to specific antigens such as microbial or vaccine peptide.
The immunoglobulin class (isotype) is determined by the heavy chain. During B-lymphocyte development, genes coding for the variable and constant parts of the immunoglobulin chain are rearranged. One each of the variable (V), diversity (D) and joining (J) heavy-chain genes are rearranged to lie adjacent to the relevant heavy-chain constant-region gene, so that transcription produces a single protein with a constant and a variable portion ( Fig. 39.2 ). Approximately 10 16 different specific B-lymphocyte receptors (with a similar number of TCR) are generated. Further rearrangement to bring a particular variable gene combination to lie adjacent to a different heavy-chain constant-region gene allows the B lymphocyte to switch to another antibody isotype with the same antigen specificity. The m chain is always produced first; class switching to other isotypes occurs later. Specific antibody diversity is created by the large number of possible combinations of variable-region genes that can be selected and is increased further by a very high mutation rate in V genes. A similar but slightly less complex process takes place with light chains, of which there are two types, kappa and lambda.

IgM is the first antibody class produced in primary immune responses. For most antigens, there is a subsequent switching to other classes. Its large pentameric structure confines it to the intravascular space, where it fixes complement.
IgG is the main class of antibody produced in secondary immune responses. Functions include opsonisation, complement fixation leading to C3 opsonisation and complement-mediated lysis, neutralisation of toxins or viruses, and participation in antibody-dependent cellular cytotoxicity. Though there is considerable overlap, the four subclasses of IgG tend to have different functions. Responses to protein antigens are mainly in the IgG l and IgG 3 subclasses. IgG 2 binds polysaccharide, such as group B streptococcal surface antigen. The role of IgG 4 is, as yet, unclear.
IgA is the main antibody in secretions, where it forms a dimeric molecule. In addition to protecting mucous membranes and the gastrointestinal tract from infection, IgA may have a role in limiting food antigen uptake from the gut. Serum IgA is mainly in monomeric form, though dimeric and trimeric forms are also found. It is secreted in breast milk.
Mast cells and basophils have receptors for the Fc part of IgE. Antigen binding causes degranulation of the cells, triggering an immediate hypersensitivity response.
The final stages of B-lymphocyte differentiation require stimulation with the appropriate antigen, leading to maturation into plasma cells. The full process requires the cooperation of antigen-specific T helper (CD4+) lymphocytes. Isotype switching from IgM production to the other antibody classes is critically dependent on T/B-lymphocyte interaction via the B-lymphocyte CD40 antigen and the CD40 ligand expressed on activated T lymphocytes (CD40L, CD154).
Cell-mediated immunity
T (thymus-derived) lymphocytes kill intracellular microbes and regulate immune responses by secreting cytokines and growth factors.
T lymphocytes develop from bone marrow stem cells, but mature in the thymus, where their receptor genes (TCR) are rearranged to generate the diversity of lymphocyte receptors needed to counter every potential antigen. Self-reactive T lymphocytes are deleted and only those recognising antigen in association with self MHC are preserved and exit the thymus. Regulatory CD4+ T lymphocytes (T REG ) help maintain peripheral tolerance to self antigens.
The TCR is similar to the immunoglobulin molecule, with constant and variable parts and the generation of diverse specificity achieved in a similar way. Recombinase activating genes 1 and 2 ( RAG1, 2 ) are critical for TCR gene rearrangement. During thymic maturation, T lymphocytes also acquire important functional surface molecules. CD3 is closely associated with the TCR. The CD4+ T-helper lymphocyte is the primary effector cell. Once switched on by antigen presentation via the MHC II molecule, these cells develop either by the T H 1 route, with production of the cytokines interleukin-2 (IL-2) and IFN-γ, stimulating macrophage function, cell-mediated immunity and B-lymphocyte class switching to IgG 2 production, or to T H 2 cells, which produce predominantly IL-4 and IL-10, which promote antibody responses and class switching, particularly towards IgG 1 , IgG 4 and IgE. Allergic responses and those against parasites are of T H 2 type. Antigen responses may follow a T H 1 or T H 2 route, depending on a complex set of circumstances. CD8+ cytotoxic lymphocytes are activated by antigen presentation via the MHC I molecule, and directly kill virally infected cells.
The development of the immune system
Prenatal development
T- and B-lymphocyte development commences early in human embryogenesis ( ). Cells capable of responding in mixed lymphocyte culture, or to mitogens, and recognisable NK cells are present in fetal liver from 6 weeks. T- and B-lymphocyte precursors are identifiable from 7–8 weeks. Thymic stroma starts to form from the sixth week; T-lymphocyte precursors and stem cells colonise the rudimentary thymus from the ninth week. By the second trimester, circulating lymphocytes with mature T-lymphocyte surface markers, including CD3+, CD4+ and CD8+, are present in fetal blood. Surface B-lymphocyte markers, including surface immunoglobulin, are also expressed at this stage. However, lymphocyte response to antigens, especially by making immunoglobulin, is limited until birth.
Absence of these lymphocyte markers can be used for the antenatal diagnosis of severe combined immunodeficiency (SCID) by fetal blood sampling.
The non-specific elements of immunity also develop early. Neutrophil precursors can be identified in the yolk sac; mature neutrophils appear in the circulation in the second trimester, but numbers are low until the onset of labour. Complement components are present by 6 weeks of gestation.
The immune system in the newborn
The primary mechanism for distinguishing harmful from benign antigens depends on the activation status of APCs, especially dendritic cells, also known as ‘professional’ APCs. Lymphocyte responses can be activated by costimulatory molecules in the presence of ‘alarm signals’ from the environment, e.g. proinflammatory cytokines when tissues are under stress, or when pathogenic microbial products are present; and rendered tolerant in the absence of such costimulatory signals. Hence, mature virgin neonatal cells can be immunised, tolerised, or switched to T H 1 or T H 2 responses according to the dose of antigen ( ), the type of antigen in the environment ( ) or the type of APC ( ).
Neonatal T lymphocytes, therefore, can behave like adult T lymphocytes ( ) and are similarly capable of activation if appropriately stimulated by APCs. The immune system is dependent on evolutionarily conserved pathogen pattern recognition receptors on cells of the innate immune system; these allow recognition of certain pathogen-associated molecular patterns not found in the host, e.g. human Toll-like receptors. These pattern recognition molecules induce activation in cells of the innate immune system such as dendritic cells. However, the immune systems of both full-term and preterm infants exhibit a physiological immunodeficiency ( ), though it is more marked in the premature, and particularly in the sick or stressed infant. This accounts for the newborn’s increased susceptibility to infection, whether overwhelming group B streptococcal sepsis or disseminated herpes simplex infection. Placentally transferred IgG partially offsets the deficiency, but transfer of immunoglobulin occurs late in gestation, and so preterm infants have significantly reduced levels; those at the limit of viability (23–24 weeks) have extremely low levels. The protective effect of maternal immunoglobulin depends on the mother having the appropriate antigen-specific IgG antibody, and in group B streptococcal sepsis, lack of specific maternal antibody is a major risk factor. The newborn infant shows particularly poor antibody responses to polysaccharide antigens, does not switch from making IgM to IgA and IgG so readily and does not produce tightly sticking antibody.
Neonatal T lymphocytes differ in other ways. There is a high CD4 : CD8 ratio, and there is usually less than 10% of the memory isoform and predominant expression of the naïve isoform. These proportions gradually reverse during childhood. Exposure to intrauterine infection often provokes a more ‘mature’ picture.
Naïve T lymphocytes are harder to stimulate, accounting for poorer responses in neonates. Furthermore, the overall balance of neonatal T-lymphocyte responsiveness is tilted towards a T H 2 rather than a T H 1 response. This may contribute to the susceptibility to intracellular bacterial pathogens, such as Listeria monocytogenes or Salmonella species, since defences to these pathogens rely on a T H 1 pattern response.
Non-specific immune mechanisms are also immature at birth. Neutrophil bone marrow reserves are easily exhausted, leading to neutropenia; chemotaxis and cell deformability are also reduced ( ), whilst neutrophil numbers and function tend to deteriorate in the presence of infection. At term, complement levels and function are at approximately two-thirds of the adult value, and often below one-half in preterm babies, although it is not clear whether these findings significantly predispose to neonatal sepsis.
Whilst low immunoglobulin and complement levels are directly proportional to gestational age, depressed T-lymphocyte function may occur in infants who have suffered severe intrauterine growth restriction. Intrauterine growth restriction has been demonstrated up to 5 years of age, though the clinical significance of such findings is unclear. The placental transfer of immunoglobulin in situations of severe intrauterine growth restriction is probably also compromised, though not all studies have confirmed this ( ).
Postnatal development
Following birth, exposure to a wide variety of antigenic stimuli triggers immunological maturation, regardless of gestational age. Initially IgM is mostly produced, but gradually IgG responses develop, and by 2 months of age, infants are able to produce good IgG antibody responses to protein vaccines, such as tetanus toxoid. During this period, maternal IgG levels fall due to catabolism, and a physiological nadir in IgG level occurs at 3–6 months of age, before the infant’s production picks up ( Fig. 39.3 ). Thereafter, isotype levels rise at different rates; adult levels of IgM are achieved by 4–5 years, IgG by 7–8 years, whilst serum IgA levels and secretory IgA (SIgA) rise only very slowly, not achieving adult values until the teenage years. Most antipolysaccharide IgG antibody in adults is found in the G2 subclass, and whilst young children make G1 polysaccharide responses, this immaturity probably explains infants’ susceptibility to polysaccharide-encapsulated organisms such as pneumococcus and the lack of responsiveness of children under the age of 18–24 months to pure polysaccharide vaccines, such as pneumococcal vaccine ( ). Conjugation of the polysaccharide to a protein or peptide facilitates early responsiveness to both components, as demonstrated by the high efficacy of Hib conjugate vaccine and the pneumococcal conjugate vaccines, although prematurity can blunt the antibody responses when certain vaccine combinations are used ( ; ).

T-lymphocyte immunity matures rapidly in the early weeks of life following antigen exposure. T-lymphocyte expression of CD40 ligand improves, as does cytokine production, with the T H 1/T H 2 balance shifting towards T H 1. However, maturation and development of the cell-mediated immune system continue through early childhood. Subtle immaturities in cell-mediated immunity probably account for the increased susceptibility of young children to tuberculosis and of young infants to invasive salmonellosis and listeriosis.
The maternofetal immunological relationship
The close physiological contact at the placental interface of the fetus and mother has important effects on immunological development and disease. During pregnancy, the mother and her incompatible fetus are relatively immunologically hyporesponsive, preventing rejection of the fetus and allowing pregnancy to be maintained. The changes involve polarisation of T-helper lymphocytes towards T H 2 and regulatory (T reg ) responses in both mother and fetus. In humans, during the third trimester, fetal T cells are able to mount antigen-specific responses to environmental and food-derived antigens. Antigen-specific T lymphocytes are detectable in cord blood almost universally, indicating that in utero sensitisation occurs. If the pre-existing T H 2 dominance is not counterregulated effectively, an allergic phenotype may well develop. The neonate possesses all the elements to develop a robust immune system, but most are downregulated. During the initial period of immune maturation, the neonate is at risk of infection, and relies on the mother for ‘immune protection’ via transplacental transfer of immunoglobulins, and by immune protective agents in breast milk. The fact that the innate immune system of a newborn baby is able to mount a robust, and occasionally exaggerated, proinflammatory response under certain conditions ( ) is testimony to the fact that all of the elements for the development of a robust immune system are present, but most elements are downregulated at birth.
Maternal IgG is actively transferred across the placenta to the fetus from about 12 weeks’ gestation, and by term, cord blood IgG levels are higher than those in maternal blood. IgG 1 and IgG 3 are principally transferred but little IgG 2 is transferred, compounding the poor neonatal response to encapsulated bacteria such as the group B streptococcus.
Placentally transferred maternal IgG occasionally causes fetal or neonatal disease. Most maternal antifetal immunoglobulins bind to fetal antigen expressed at the centre of the placental villus, and thus never cross to the fetus. However, some antigens expressed on highly differentiated fetal cells are not expressed at the placental villus, and so antibody can cross and cause disease such as Rhesus disease, neonatal thyrotoxicosis and myasthenia gravis. Neonatal haemochromatosis is a rare and often fatal disease which can be ameliorated by giving high-dose immunoglobulin to the mother during pregnancy, and hence may have an autoimmune basis ( ). Maternal anti-Ro antibodies, found in systemic lupus erythematosus (SLE), can cause a transient neonatal SLE, but also damage the fetal cardiac conduction pathway, leading to permanent neonatal heart block which may require cardiac pacing. Maternal hypogammaglobulinaemia may be manifest in the newborn infant, as there will have been no placental transfer of IgG. Maternal medication can also cross the placental barrier, and maternally acquired immunosuppressive medication such as ciclosporin or azathioprine can lead to transient neonatal lymphopenia and hypogammaglobulinaemia. Viruses such as cytomegalovirus (CMV) can also cross the placenta and are not effectively excluded by fetal cell-mediated immunity, allowing progressive infection or persistent viral excretion.
Immune development and the neonatal gut (written with Alison Bedford Russell)
The newborn gut is critical to the development of normal immune defence mechanisms in the infant, through the interaction of colonising organisms, nutrients and gut-associated lymphoid tissue, and nutrition and immune function are closely interlinked. Understanding the importance of early exposure to maternal breast milk and the benefits of prophylactic use of pre- and probiotics and lactoferrin in reducing neonatal sepsis and necrotising enterocolitis (NEC) rates, require knowledge of gut immune mechanisms.
In utero, the fetal gut is active and the fetus starts to swallow up to 200–250 ml/kg/day of amniotic fluid, containing carbohydrates, proteins, peptides, lipids and trophic growth factors ( ) at around 8 weeks ( ), contributing to gut development ( ; ; ). Normally, in the immediate peripartum period, the gut becomes colonised with maternal skin and gut bacteria, inducing the development of a competent and functional immune system. This exposure to maternal bacteria and concurrent provision of immunological factors in breast milk are key events which promote gut-associated and systemic immunity, and also nutritional and functional gut maturation ( ).
The gut of the human adult contains 10 times as many bacterial cells as there are human cells in the body (10 14 in the gut versus 10 13 human cells) and more than 400 different species of bacteria, mostly commensals ( ). In addition to microbial antigens, the gut is exposed to a large number of complex dietary antigens. It is important that each individual develops tolerance of commensal faecal flora and dietary antigens, whilst able to react vigorously to intestinal pathogens ( ; ). Such balance of immunological response is facilitated through the interaction of innate and adaptive immune systems ( ).
Toll-like receptors and NOD proteins on dendritic cells and M cells sense luminal and mucosally adherent bacteria ( ), in organised lymphoid tissue of Peyer’s patches or mesenteric lymph nodes, or away from organised lymphoid tissue, as dendritic cells can interdigitate between enterocytes and extend processes into the lumen ( ). The local cytokine environment is critical in shaping dendritic cell–lymphocyte interactions ( ). B lymphocytes switch from IgM production to other immunoglobulin isotypes, dependent on the local cytokine environment and cell–cell contact with T lymphocytes ( ; ). Generally, IgA responses protect against inflammation, whereas IgG is proinflammatory and promotes bacterial opsonisation. IgE responses may promote inflammation by disrupting epithelial barrier and neural function. Three major groups of CD4+ T helper lymphocytes can drive different forms of intestinal inflammation – T H 1, T H 2 and T H 17 cells. T H 17 cells are generated in response to transforming growth factor-β (TGF-β), IL-23 and IL-6 ( ) and produce IL-17 ( ; ). T REG populations, produced in response to TGF-β or IL-10 ( ; ; ; ), include T H 3 cells, which produce TGF-β – a key cytokine for mucosal IgA responses. If pathogens induce inflammatory local cytokine production at the time of initial priming, sensitisation, rather than tolerance, may develop.
There are two ways in which this flora-driven immune programming may be disrupted: either by alterations in the colonising flora in early life, or by reduced host immune reactivity. The basis of the ‘hygiene’ hypothesis relating to allergic, autoimmune and inflammatory diseases is now thought to relate to failed induction of regulatory immune responses in abnormal early-life infectious exposure, rather than the straightforward deviation of T H 1/T H 2 balance previously postulated. First infectious exposures of the nascent immune system shortly after birth may be of much greater importance to immune development than later exposures, and disordered immune programming may result in immune dysregulation with lifelong effects.
The bacteria which arrive in the newborn gut first, usually coliforms and other maternal gut bacteria, establish a permanent niche by interacting with gut-associated lymphoid tissue, and are at a competitive advantage to those arriving late. The normally born infant will be exposed to normal maternal gut flora, which induces normal gut and immune maturation ( ). Colonisation of the newborn is supported and ‘made safe’ by the baby receiving its own mother’s breast milk as soon as possible after delivery. The mother’s breast milk contains maternal flora-specific SIgA, which prevents invasion of gut mucosa by potentially pathogenic species, as well as lactobacilli and bifidobacteria (known as probiotic species), and complex carbohydrates which have prebiotic activity ( Fig. 39.4 ). A baby born by caesarean section will have very different colonising gut bacteria than one born by normal vaginal delivery, face to anus ( ). Peripartum antibiotics inevitably alter the composition of gut flora, with possible later consequences for the child’s immune health ( ), and antibiotic therapy is associated with abnormal faecal flora profiles in preterm babies within a neonatal unit ( ).

Colostrum contains a rich supply of maternal faecal flora-specific SIgA. The major breast milk protein, lactoferrin, has microbicidal, immunostimulatory and anti-inflammatory activities as a result of blocking production of Il-6, Il-8, Il-1β and TNF-α. In addition, breast milk contains immunoregulatory cytokines such as TGF-β, and lymphocytes expressing gut homing markers ( ). Other protective factors in breast milk include lysozyme, neutrophils and macrophages. Importantly, bacteria, particularly bifidobacteria, are also transported via breast milk and have an important role in modifying the microbiota of the neonatal gastrointestinal tract, which in turn influence mucosal immunological maturation (M’ ). The naïve gut thereby becomes colonised with more normal bacteria, with responses modulated by transferred maternal antibody and immune cells, which further assist with inducing normal gut and immune development ( ) .
However, breast milk may also be a vehicle through which CMV can be transmitted, which may be of particular concern in preterm or immunodeficient infants ( ).
Immunodeficiency disorders: general principles
Classification and genetics
Immunodeficiency may be due to primary or secondary defects. The World Health Organization working party on immunodeficiency has classified the primary disorders ( ) ( Table 39.2 ). The incidence of any significant immune deficiency disorder (excluding selective IgA deficiency) is 1 in 10 000.
IMMUNODEFICIENCY | CELLS AFFECTED | GENE DEFECT | INHERITANCE | NEONATAL PRESENTATION |
---|---|---|---|---|
Cell-mediated | ||||
SCID | ||||
T − B + NK − | T, NK | CgC | XL | Yes |
T − B + NK − | T, NK | JAK3 | AR | Yes |
T − B + NK + | T | IL7Ra | AR | Yes |
T − B − NK + | T, B | RAG 1,2 | AR | Yes |
T − B − NK + | T, B | Radiosensitive SCID (artemis, DNA PKcs) | AR | Yes |
T + B − NK + | T-B-SCID with MFE or Omenn syndrome | AR | Yes | |
Reticular dysgenesis | T, B+/−, NK, neutrophils | AK2 (deafness) | AR | Yes |
ADA deficiency | T, B, NK | Adenosine deaminase | AR | Yes |
PNP deficiency | T, B, NK | Purine nucleoside phosphorylase | AR | No |
CID | ||||
MHC II deficiency | Low T (CD4) | Defective MHC II expression | AR | No |
MHC I deficiency | Low T (CD8) | Defective MHC I expression | AR | No |
CD40L deficiency (XL hyper-IgM) | T | Defective CD40L | XL | No |
Wiskott–Aldrich syndrome | T, B, platelet | WAS | XL | Yes |
ZAP 70 kinase deficiency | T (low CD8) | ZAP 70 kinase | AR | No |
XL lymphoproliferative disease | T, B | SAP | XL | No |
DiGeorge syndrome | T, B | 22q11 deletion | Sporadic, occasional AD | Yes |
Cartilage hair hypoplasia | T, B | RMRP gene | AR | Yes |
Ataxia telangiectasia | T, B | ATM gene | AR | No |
Nijmegen breakage syndrome | T, B | NBS1 gene | AR | Yes |
Antibody deficiency | ||||
XL agammaglobulinaemia | Absent B | Btk | XL | No |
XL hyper-IgM (see CD40L deficiency) | ||||
AR hyper-IgM syndrome | B | AID, CD40 | AR | No |
AR agammaglobulinaemia | Absent B | m chain | AR | No |
Transient hypogammaglobulinaemia of infancy | Unknown | Unknown | No | |
Phagocyte defects | ||||
XL chronic granulomatous disease | Neutrophils | Killing gp91phox | XL | Yes |
AR chronic granulomatous disease | Neutrophil killing | Killing gp22phox, gp47phox, gp67phox, p40phox Killing gp67phox | AR | Unusual |
LAD I | Neutrophil adherence | CD18 | AR | Yes |
Chédiak–Higashi | Chemotaxis | AR | Yes | |
Griscelli | Chemotaxis | AR | Yes | |
Congenital neutropenia | Absent neutrophils | ELA2, HAX1 WAS | AR XL | Yes |
(Kostmann’s syndrome) | Sporadic | |||
Shwachman syndrome | Chemotaxis | SBDS | AR | Yes |
Hyper-IgE syndrome | Macrophages, neutrophils, lymphocytes | STAT3, TYK2 | AD, AR | Yes |
Mycobactericidal defect | Macrophages, T | IFN-γ receptor 1, 2 IL-12 receptor IL-12 | AR | Yes |
Complement deficiencies | ||||
C1–9 | C1–9 gene defects | AR | No | |
Properdin | Properdin gene | XL | No | |
Factor H, I | Factor H, I gene | AR | No |
In many of the primary disorders, the molecular basis is understood ( ). These discoveries have enhanced understanding of the immune response and led to more focused treatment of primary immune deficiencies. Precise genetic diagnosis has also aided antenatal diagnosis.
Diagnosis and investigation of immunodeficiency
A careful history and examination should precede any laboratory tests for immunodeficiency, as this will help indicate which children should be investigated further and determine the nature and extent of investigations ( Table 39.3 ).
History |
|
Examination |
|
Investigations |
|
History
Pregnancy and birth history may suggest possible congenital infection, intrauterine growth retardation or prematurity, all of which are associated with immune defects. Delayed separation of the umbilical cord, in the absence of local infection, may suggest a neutrophil defect. Risk factors for human immunodeficiency virus (HIV) in the parents should be sought. A family history may reveal other children with unusual or fatal infectious complications, suggesting an autosomal-recessive (AR) or X-linked pattern of inheritance. A history of consanguinity is important. In some disorders, such as IgA deficiency, there may be a family history of collagen vascular or other immunopathological disease. Older relatives who are carriers of, or who are affected by, milder variants of primary immune defects may have autoimmune manifestations (e.g. mouth ulcers and SLE variant in chronic granulomatous disease (CGD)) or have a history of malignant disease (lymphoma in X-linked lymphoproliferative disease).
Examination
Examination should be directed towards potential sites of infection, including the throat, ears and mouth, and nappy area for candidiasis ( Table 39.3 ). The presence or absence of lymphoid tissue should be noted. In more severe immunodeficiency states such as SCID and X-linked agammaglobulinaemia, there is a lack of tonsils and lymphoid tissues, although visualising tonsillar tissue in neonates and small infants can be very difficult. Some diseases may have specific physical signs, such as oculocutaneous albinism in Chédiak–Higashi syndrome, typical facies and/or cleft palate in DiGeorge syndrome, and disproportionate short stature in some forms of combined immune deficiency, such as cartilage hair hypoplasia. Microcephaly with developmental delay may be seen in some DNA repair disorders associated with immunodeficiency ( ). Neonatal pustulosis may be seen in the hyper-IgE syndrome due to STAT3 deficiency, or in chronic granulomatous disease (CGD).
Radiological evaluation
Radiological evaluation, directed by findings from history and examination, may be useful. Bony abnormalities may be seen in adenosine deaminase (ADA) deficiency ( ), in Shwachman–Diamond syndrome, or in other dysplasias associated with immune defects. Absence of a thymus on anteroposterior and lateral chest radiographs is consistent with a combined immune defect in infants and young children, but thymic atrophy may also occur in response to stress (e.g. infection), and this finding is not diagnostic.
Laboratory investigation
The following should trigger investigation:
- •
family history consistent with immune deficiency
- •
single infection with an unusual/opportunistic organism (e.g. Pneumocystis jiroveci )
- •
single infection which is atypically severe or has an atypical course
- •
more than one episode of serious bacterial infection ( Table 39.3 ).
Haematology
A full blood count and blood film examination will readily detect neutropenia. Bone marrow aspiration will distinguish between failure of production and increased peripheral destruction, and exclude a myelodysplastic or malignant process. Neutrophilia in the absence of overt infection may suggest a neutrophil adhesion defect or functional problem (e.g. CGD). Lymphopenia, using appropriate age-related ranges, is highly suggestive of a combined immune deficiency of primary or secondary aetiology ( ), but a normal lymphocyte count does not exclude SCID. A manual differential will differentiate nucleated red cells in infants and abnormal leukocyte morphology in sick children. Abnormal leukocyte granules are characteristic of Chédiak–Higashi syndrome. Platelet volume is universally low in Wiskott–Aldrich syndrome (WAS). Eosinophilia may be marked in Omenn syndrome, immunodysregulation polyendocrinopathy enteropathy X-linked (IPEX) syndrome and hyper-IgE syndrome.
Tests of innate immunity
Complement function should be assayed by testing the ability of patient serum to lyse sensitised red blood cells, thus testing the whole pathway. Deficiency in any one component will result in a failure of lysis. Samples must be separated and frozen within 2 hours of venesection. If repeat testing shows a persistent abnormality, evaluation of individual complement components should be performed. Mannan-binding lectin deficiency is detected by analysing the genotype; complete deficiency may be associated with severe infection.
Neutrophil function tests are technically difficult, as neutrophils activate and then quickly die after venesection. The nitroblue tetrazolium (NBT) test is a rapid and sensitive test for CGD, but false normal results can be seen when the test is performed infrequently; it is now being superseded by the more reliable flow cytometric evaluation of the neutrophil oxidative burst.
Adaptive immune system
Test of humoral immunity
Immunoglobulins
IgG is the predominant circulating immunoglobulin, and in the neonate, most is maternal in origin. Smaller amounts of IgA, IgM, IgD and IgE are found in serum. Results must be compared with age-specific normal ranges ( Appendix 6 ), as production of all five classes of immunoglobulin is low at birth and gradually matures over the first 5 years of life. Immunoglobulin can be lost via the gut or urinary tract. Low levels of immunoglobulin can only be attributed to a production defect if gut or renal losses have been excluded and the serum albumin is within the normal range. Measurement of IgE is indicated if hyper-IgE (Job’s) syndrome, IPEX syndrome or Omenn syndrome is included in the differential diagnosis; however, in hyper-IgE syndrome the level may be normal in the neonatal period.
Measures of in vivo antibody responses
Functional antibody is more important than the amount of circulating antibody. Functional tests of IgG production rely on measuring antibody titres to antigens to which the child is known to have been exposed either naturally or by vaccination. Responses to protein antigens such as tetanus and the conjugated Hib vaccine are widely available.
Cell-mediated immunity
Cell-mediated defects are likely to result in poor antibody responses, as these depend on T-lymphocyte help in making an antibody response with memory.
Quantification of cell numbers
Lymphocytes can be enumerated using flow cytometry, characterised by their cell surface markers and expressed either as a percentage of the lymphocyte pool or as an absolute number. Proportions of different lymphocytes and absolute numbers vary with age, and age-related normal ranges should be consulted ( ).
In vitro lymphocyte proliferation assays
Culturing lymphocytes for a defined time period with an appropriate non-specific stimulus and using the incorporation of tritiated thymidine or a non-radioactive marker such as bromodeoxyuridine into the DNA of dividing cells acts as a surrogate measure of cell proliferation. An alternative method utilises the stable incorporation of the intracellular fluorescent dye 5-carboxyfluorescein diacetate succinimidyl ester (CFSE) into cells to quantify cell division, because of the sequential decrease in fluorescent labelling in daughter cells. CFSE labelling enables a specific lymphocyte subpopulation proliferation to be analysed.
Definition of molecular defects
The genetic basis of an increasing number of immune deficiencies is well defined. Usually, a gene defect coding for the protein results in no protein expression, expression of low amounts or expression of abnormally sized protein, and can be detected by Western blotting and flow cytometry ( ). In the presence of an appropriate history or abnormal protein expression, genetic analysis may be undertaken.
Carrier testing
When a primary immunodeficiency is diagnosed, families should be offered genetic counselling. Parents can be tested for carrier status by mutation analysis if the mutation has been defined. Carrier status can also be determined by other tests such as the NBT, in which intermediate numbers of neutrophils will reduce NBT in mothers who carry the mutation.
Antenatal diagnosis
Appropriate counselling by an individual conversant with the outcome of immune deficiencies in the modern era should be undertaken before antenatal diagnosis is offered. Testing confers a small risk of miscarriage, and so screening should be offered only to mothers in whom the result would materially influence the outcome of the pregnancy, although antenatal tissue typing can be performed, and potential donors identified if the disease is treatable by haematopoietic stem cell transplantation.
Neonatal screening
Outcome of stem cell transplantation is much better for patients with SCID transplanted in the neonatal period when infection-free, than for older infants ( ). Screening programmes measuring molecular markers of T-lymphocyte production, absent in SCID, have been successfully developed from the Newborn Bloodspot Screening Program in the USA ( ).
Specific primary immunodeficiencies
The complete spectrum of primary immunodeficiency is beyond the scope of this chapter, which will focus on those disorders that present very early in life. More comprehensive texts are recommended for those requiring more information ( ; ).
Disorders of cell-mediated immunity: severe combined immunodeficiencies
Failure to develop normal T and B lymphocytes is usually due to a specific gene defect affecting early lymphocyte development or subsequent signalling pathways, but as T lymphocytes are critical for the maturation and function of B lymphocytes, the more severe T-lymphocyte deficiencies are often accompanied by defective antibody responses and also result in combined deficiency. Combined immunodeficiency results from a large number of disorders with X-linked or AR inheritance, most of which are now molecularly defined ( Table 39.2 ). The most severe phenotype is SCID ( Fig. 39.5 ) associated with a profound T lymphopenia and panhypogammaglobulinaemia, with early death from infection. Whilst the usual clinical features of this group of diseases are well characterised, atypical presentations and ‘leaky’ forms with an attenuated phenotype are increasingly recognised. Circulating T-lymphocyte numbers are usually low or absent but may be normal. In the classic SCID presentation, lymphocyte responses to mitogen are absent. Patients usually have a limited diversity of T-lymphocyte receptor and immunoglobulin gene rearrangements. Identifying the molecular defect in specific patients with combined immunodeficiency or SCID is important for prognosis, treatment and genetic counselling.

General features of severe combined immunodeficiency
Affected infants appear well at birth but within the first few months of life fail to clear infection and present with persistent respiratory tract or gut infection, failure to thrive and, sometimes, apparent food intolerance ( ). Persistent respiratory tract infection with respiratory syncytial virus or parainfluenza viruses is common, with failure to clear virus accompanying persistent bronchiolitic-like signs. An insidiously progressive persistent respiratory infection with radiological evidence of interstitial pneumonitis should raise the suspicion of Pneumocystis jiroveci infection, often a co-pathogen with respiratory viruses ( ). Other presentations include prolonged otitis media and invasive bacterial infections, particularly staphylococcal or Pseudomonas septicaemia and pneumonia, which may respond poorly to appropriate treatment. Severe invasive fungal infection is rare, but often fatal. Extensive persistent superficial candidiasis is more common. Occasionally infants present with disseminated bacille Calmette–Guérin (BCG).
Congenital graft-versus-host disease (GvHD) occurs because many infants with SCID are unable to reject foreign lymphocytes, acquired either from the mother in utero ( ) or from a non-irradiated blood transfusion. When the disease is clinically apparent, there is typically a mild reticular skin rash, sometimes with abnormal liver function tests. GvHD following transfusion with non-irradiated blood or white cell or platelet concentrates is generally more severe and can be fatal. In these cases, the skin rash is severe and lymphadenopathy and hepatosplenomegaly may be present. The clinical picture may be indistinguishable from Omenn syndrome, but identification of maternal cells by karyotype or DNA fingerprinting will distinguish maternofetal GvHD from Omenn syndrome.
Examination usually reveals a wasted child who has dropped through the growth centiles, with evidence of candidiasis and other infections. Skin rashes may indicate infection or GvHD. There is no clinically detectable lymphoid tissue. There may be hepatomegaly.
Investigation usually shows severe lymphopenia from birth. It should be remembered that in infancy a normal lymphocyte count is >2.7 × 10 9 /l (unlike adults, where it is >1.0 × 10 9 /l). Lymphocytic phenotyping shows severely depleted T-lymphocyte numbers; B lymphocytes and NK cells may be present or absent, depending on the type of SCID. Occasional variants show unusual patterns of immature T-lymphocyte markers; in such cases, maternal engraftment should be excluded. Mitogen responses are usually absent. Immunoglobulin estimations show low levels of IgG, IgA and IgM but residual maternal IgG may give a falsely reassuring result and it can be difficult distinguishing IgA and IgM levels in SCID from the low levels seen in normal infants. Isohaemagglutinins are a useful measure of in vitro IgM production, and absence is significant. If SCID is suspected, lymphocyte phenotyping is more reliable than immunoglobulin estimation. Chest radiographs show an absent thymus and, if infection is present, hyperinflation and/or interstitial pneumonia.
In children who die, postmortem examination reveals severely depleted lymphoid tissue, with nodes and thymus showing no lymphoid follicles and absent Hassall’s corpuscles in the thymus. Without treatment, patients die from infection by about 12 months of age. Currently, the only curative treatment is haematopoietic stem cell transplantation (HSCT), although clinical gene therapy trials for common gamma chain and ADA deficiency are in progress. Supportive interim treatments include antibiotic prophylaxis with co-trimoxazole as anti- Pneumocystis treatment, antifungal prophylaxis and immunoglobulin replacement. Live vaccines, including BCG, must be avoided. The diagnosis of SCID is a paediatric emergency, and suspected cases should be urgently referred to a designated treatment centre for assessment and treatment.
Newborns suspected of having a severe immunodeficiency disorder should be protected using isolation techniques, including limitation of the numbers of persons involved with care; individuals with respiratory or gastrointestinal symptoms of infection should avoid contact. If the mother is CMV-negative, breastfeeding should be encouraged; otherwise it should be discontinued to prevent neonatal CMV infection being transmitted through the milk. Wherever the child is managed, the importance of strict hand-washing procedures cannot be overemphasised. Blood products should be CMV-negative and irradiated to avoid the risk of transfusion GvHD.
Types of severe combined and combined immunodeficiencies
Severe combined immunodeficiency
SCID can be classified according to the presence or absence of T, B and NK lymphocytes, each phenotype being due to a number of distinct molecular defects, so enabling classification by mechanism ( Table 39.2 ). The genetic basis of a few disorders remains to be elucidated. Other rare phenotypes may be due to atypical presentation of known molecular defects.
Omenn syndrome
Omenn syndrome is characterised by a generalised erythematous rash, together with scaling and erythroderma, lymphadenopathy, hepatosplenomegaly, increased serum IgE levels with a marked eosinophilia and the usual clinical features of SCID ( Fig. 39.6 ) ( ). The syndrome is a ‘leaky’ form of SCID in that small numbers of very abnormal T lymphocytes ‘leak’ past the block in T-lymphocyte development. The underlying defect in many cases is a mutation in the RAG genes, although other gene mutations have been associated with the phenotype ( ). HSCT is the only curative treatment.

Netherton syndrome
This triad of generalised infantile erythroderma, diarrhoea and failure to thrive may be associated with a variable immunodeficiency, including mild lymphopenia. The clinical features are similar to those seen in Omenn syndrome and in SCID and maternofetal engraftment. Distinguishing these entities is important, as the other conditions are treated by HSCT, whereas Netherton syndrome is not ( ). Mutations in the serine protease inhibitor Kazal-type 5 (SPINK5) gene, are reported in most patients. Hair shaft abnormalities with trichorrhexis invaginata (bamboo hairs) are diagnostic.
Reticular dysgenesis
This rare AR-inherited form of SCID, due to a defect in mitochondrial adenylate kinase 2, is characterised by defective lymphoid and myeloid differentiation ( ). Neural deafness is an associated feature.
Adenosine deaminase deficiency
This disorder, due to a single gene defect, results in absence of the purine salvage pathway enzyme ADA, leading to accumulation of the toxic DNA breakdown products. Neurodevelopmental problems may also occur in some patients ( ). Treatment is by HSCT or by use of replacement polyethelene glycol-coupled ADA. Gene therapy trials are in progress for this form of SCID ( ).
Major histocompatibility complex class II deficiency
MHC class II antigens (HLA DR, DP, DQ) are expressed on a limited repertoire of cells and present antigen to CD4+ T lymphocytes, which, with the help of an appropriate second signal, leads to the activation of T-helper lymphocytes specific for that antigen and an effective immune response. Expression of MHC II in the thymus is also essential for positive selection of CD4+ T lymphocytes. Since HLA antigens are of vital importance for lymphocyte development and function, lack of MHC II expression results in a profound susceptibility to viral, bacterial, fungal and protozoal infections ( ).
Autosomal-recessive MHC II deficiency is rare and can result from mutations in several different genes which code for a complex of regulatory factors controlling transcription of MHC II genes.
The clinical picture resembles SCID. The diagnosis can be confirmed flow cytometrically by showing absent or significantly reduced levels of class II molecules on B lymphocytes and monocytes. Definitive treatment is HSCT, although this has had limited success in comparison with that achieved in other types of SCID ( ).
Combined immunodeficiency forming part of other syndromes
DiGeorge anomaly
This condition results from abnormal cephalic neural crest cell migration into the third and fourth pharyngeal arches in early embryological development. A microdeletion at chromosome 22q11.2 is present in 90% of cases, while the remainder are associated with other chromosomal anomalies, particularly 10p–. Whilst classically recognised by the triad of congenital heart defects, immunodeficiency secondary to thymic hypoplasia and hypocalcaemia secondary to parathyroid gland hypoplasia, an expanded phenotype is increasingly recognised. Conotruncal heart defects are most often associated with the syndrome, but other defects include tetralogy of Fallot, septal defects, pulmonary atresia and aberrant subclavian vessels.
Severe T-lymphocyte immunodeficiency presenting with a SCID phenotype of profound lymphopenia and poor lymphocyte proliferation is rare and accounts for <1.5% of cases. A similar clinical picture has been described in some patients with CHARGE syndrome (coloboma of the eye, heart defects, atresia of the choanae, retardation of growth and/or development, genital and/or urinary abnormalities and ear abnormalities and deafness) ( ).
All children suspected of having DiGeorge syndrome (with or without 22q11.2 deletion) should have an immunological evaluation including lymphocyte subset analysis, T-lymphocyte proliferative responses, immunoglobulin levels and specific antibody responses to vaccination antigens.
Blood products should be irradiated if T-lymphocyte mitogen responses are poor. Patients with severe immune deficiency have been successfully treated with HSCT: thymic transplantation may be a preferred option for some patients, although it is available in only a few centres worldwide ( ). In the partial phenotype with normal T-lymphocyte function, the usual infant vaccination schedule can be followed. It is safe to give live vaccines such as measles, mumps, rubella vaccine or varicella-zoster vaccine as long as the CD4 count exceeds 400 cells/ml, and there are normal T-lymphocyte mitogen responses. Demonstration of good antibody responses to tetanus and Haemophilus influenzae type B vaccination give further reassurance that live vaccines can be safely administered ( ). Prophylactic antibiotics and, occasionally, intravenous immunoglobulin (IVIG) can be helpful, particularly for young children with recurrent infection due to humoral deficiency.
Wiskott–Aldrich syndrome
Immunodeficiency, thrombocytopenia, eczema and an increased risk of autoimmune disorders and malignancy characterise this X-linked recessive condition. Mutations in the same gene are found in patients with X-linked thrombocytopenia and X-linked neutropenia.
Clinical features can be surprisingly mild, especially in the newborn. Thrombocytopenia with a low mean platelet volume (<5 fl) is always present and should raise suspicion of the diagnosis even if bruising and bleeding are minimal.
Acute bleeding episodes may require platelet transfusions (irradiated to prevent GvHD). IVIG, with or without prophylactic antibiotics, reduces infections, and splenectomy may suppress autoimmune phenomena. Without HSCT, the prognosis is variable, but median survival is between 3 and 15 years. The presence or absence of WAS protein correlates with outcome, with patients who have no expressed protein having a worse outcome than those with expressed protein ( ). The efficacy of HSCT for patients with WAS is long established, with good outcomes following related and unrelated donor HSCT ( ).
X-linked hyper-IgM syndrome (CD40 ligand deficiency)
X-linked hyper-IgM syndrome is a combined immunodeficiency caused by a T-lymphocyte defect and not an isolated antibody deficiency, as was previously thought. The defect is in the gene encoding for the CD40 ligand (CD154) expressed on T lymphocytes which activate B lymphocytes and monocyte/macrophage-derived cells. Without CD40L binding, B lymphocytes cannot switch from producing IgM to producing IgA, IgG and IgE. Pulmonary macrophages are not activated, resulting in Pneumocytis jiroveci pneumonia, and ineffective Kupffer cell function allows repeated infection with Cryptosporidium parvum and similar organisms, leading to sclerosing cholangitis, pancreatitis and malignancy. Absent IgA and IgG results in sinopulmonary and invasive bacterial infection. Neutropenia with oral ulceration is common and this, together with low or absent IgA and IgG, and normal or raised IgM, should suggest the diagnosis ( ).
Patients should receive co-trimoxazole, as Pneumocystis carinii pneumonia (PCP) prophylaxis, and immunoglobulin replacement therapy. With adequate replacement, the rising IgM levels may normalise. The neutropenia sometimes responds to granulocyte colony-stimulating factor (G-CSF) and IVIG. All drinking water should be boiled. Azithromycin prophylaxis may lessen the risks of Cryptosporidium parvum . HSCT should be considered for this condition ( ). A much rarer, but similar, AR disease, CD40 deficiency, presents with similar features; treatment is the same as for CD40 ligand deficiency ( ).
Immunodeficiency and short-limbed dwarfism
Cartilage hair hypoplasia is the best described form, inherited in an AR manner and associated with mutations in the RMRP gene, which encodes endoribonuclease Rnase mitochondrial RNA processing (MRP). Severe short-limbed short stature (211.8–22.1 sd ) with X-ray appearances of metaphyseal and spondyloepiphyseal dysplasia are always present, accompanied by sparse light hair in most patients. Severe anaemia and Hirschsprung disease are uncommon but well-recognised associations, as are malignancies, notably lymphoma and skin carcinoma. The immunodeficiency is surprisingly variable; most have T lymphopenia and impaired in vitro mitogen proliferative responses, but only half suffer recurrent infection. However, some have IgA and/or IgG subclass deficiencies, with frequent ear infections. Patients are excessively vulnerable to viral infections, particularly varicella-zoster virus, Epstein–Barr virus and other human herpesvirus infections, and the risk of infective death is 300 times greater than normal. Rare patients can present with a SCID immunophenotype, but no limb dysplasia ( ). Severely affected patients should be assessed for bone marrow transplantation (BMT), which has been successful in correcting the immunodeficiency ( ).
Abnormalities of T- and B-lymphocyte function are seen in a number of other osteochondrodysplasias, including Shwachman syndrome, in which there are neutropenia and pancreatic insufficiency and Schimke immuno-osseous dysplasia, which features radiographic changes of spondyloepiphyseal dysplasia, nephrotic syndrome and cellular immunodeficiency.
DNA repair defects and immunodeficiency
The immune system uses cellular DNA repair mechanisms to generate the diversity of specific immune responses by rearrangement of the variable (V), diversity19 (D) and joining (J) gene segments that code for T- or B-lymphocyte receptor genes (VDJ recombination). Inability to repair DNA damage causes cellular apoptosis or malignant proliferation, and so individuals with defective DNA repair mechanisms have a predisposition to neurodegeneration, developmental anomalies and cancer, as well as defective immunity ( ).
Single-gene defects cause a number of distinct clinical entities, of which ataxia telangiectasia is the best known, although the features are not apparent in the neonatal period. Nijmegen breakage syndrome, an AR disorder, is characterised by microcephaly, mental retardation, bird-like facies, immunodeficiency, clinical radiation sensitivity and chromosomal instability. It should be considered when investigating an infant with features of bird-headed dwarfism. Fanconi’s anaemia presents with progressive bone marrow failure leading to pancytopenia. There may also be skeletal malformations, and immunodeficiency can occur.
Immunoregulation disorders
Increasingly, patients with complex autoimmune manifestations are being recognised, often with coexistent infectious problems. Patients with classical primary immunodeficiency such as WAS or DiGeorge syndrome present with autoimmune manifestations, and it is logical to consider genetic disorders of immune regulation as primary immunodeficiencies in the broadest sense.
Defects of lymphocyte apoptosis
Autoimmune lymphoproliferative syndrome
Apoptosis (programmed cell death) regulates immune responses once an infection has been countered, to delete many antigen-specific lymphocytes that clonally expanded to deal with the infection. Apoptosis defects lead to marked autoimmune and lymphoproliferative features which characterise autoimmune lymphoproliferative syndrome (ALPS) ( ).
Chronic mucocutaneous candidiasis
Chronic mucocutaneous candidiasis (CMC) describes a heterogeneous group of primary or secondary disorders characterised by chronic infection of skin, nails and mucous membranes by organisms of the genus Candida , most commonly Candida albicans. The precise molecular defect is not known for most forms of primary CMC, although defects in cytokine signalling are apparent in some patients ( ; ). Recurrent and persistent Candida of the mouth, napkin area, skins and nails is the hallmark of this condition, but the severity varies considerably and invasive disease almost never occurs. Failure of usually effective antifungal drugs to clear Candida distinguishes primary CMC from other conditions that predispose to Candida, such as secondary immunodeficiency, steroid treatment or systemic antibiotics. Candidiasis is usually first noticed early in infancy and in severe cases gross oesophageal involvement causes dysphagia, gastro-oesophageal reflux and failure to thrive, whilst skin lesions may be extremely disfiguring and distressing. Cases may be familial or sporadic with recessive or dominant patterns of inheritance.
Treatment with azole antifungals, such as fluconazole, can be very effective, even in severe cases, but may not completely eradicate infection, and infection often recurs on stopping treatment.
IPEX syndrome
Immunodysregulation polyendocrinopathy enteropathy X-linked (IPEX) syndrome is characterised by early-onset type 1 insulin-dependent diabetes mellitus, infantile ichthyosiform dermatitis, protracted diarrhoea and severe total parenteral nutrition-dependent enteropathy and thyroiditis. Mutations in the FOXP3 gene have been described in affected patients ( ).
Other immunodeficiencies
A number of syndromes that include primary immunodeficiency as part of the phenotype have been described. Although most lack clear definition, the syndrome is well described in some, and the underlying molecular defect has been elucidated in some.
Hoyeraal–Hreidarsson syndrome
This X-linked disorder is characterised by microcephaly, cerebellar hypoplasia, aplastic anaemia and growth retardation. A progressive combined immunodeficiency, with hypogammaglobulinaemia and lymphopenia, is a well-recognised association.
ICF syndrome
Immunodeficiency, centromeric instability and facial anomalies (ICF) syndrome is an AR disorder characterised by structural chromosomal abnormalities in chromosomes 1, 9 and 16 in lymphocytes, easily seen on chromosome analysis. Other cells do not show these changes. Affected children develop severe recurrent infections and have immunoglobulin deficiency, often with agammaglobulinaemia but with normal numbers of T and B lymphocytes ( ). T-lymphocyte immunity is not normal and affected children can present with Pneumocystis carinii infection in infancy; severe viral warts and cutaneous fungal infection also occur. HSCT may be curative for selected patients.
Defects of antibody production
Isolated antibody deficiency does not usually present in the neonatal period because the presence of transplacentally acquired immunoglobulin obscures the affected child’s inability to make antibody. Neonates can present with severe or recurrent bacterial infection and may be found to have hypogammaglobulinaemia because of:
- •
failure of transfer of immunoglobulin due to prematurity
- •
failure of transfer of immunoglobulin due to unrecognised maternal hypogammaglobulinaemia
- •
immunoglobulin loss from the thoracic duct in chylothorax
- •
immunoglobulin loss from gut in lymphangiectasia.
Table 39.2 describes the main causes of primary hypogammaglobulinaemia; these can often be screened for in the neonatal period when there is a positive family history.
Disorders of phagocytic cells
Chronic granulomatous disease
Chronic granulomatous disease (CGD) results from inherited defects of the phagocyte nicotinamide adenine dinucleotide phosphate (NADPH) oxidase enzyme complex, which generates free oxygen species needed to kill microorganisms ingested into phagosomes. The X-linked form is more common than the AR form.
The disease has protean clinical manifestations, but the hallmark is acute, and potentially fatal, bacterial or fungal infection ( ). In the neonatal period, a non-specific generalised pustulosis can be seen, and the most common manifestation, acute suppurative lymphadenitis in the neck, axilla or groin, can sometimes be seen very early in life. Other frequent pyogenic infections include liver abscesses, osteomyelitis, arthritis, pneumonia, skin sepsis and perianal abscesses. Pathogens such as Staphylococcus aureus , Burkholderia cepacia , Aspergillus species and Serratia marcescens , are common. Infections with catalase-negative organisms, such as Streptococcus pneumoniae , are rare. Fungal infection often manifests as pneumonia, but disseminated infection is frequently seen, with osteomyelitis and hepatic involvement. Once established, fungal infection is hard to treat and frequently fatal.
Diagnosis is suggested by failure of reduction of NBT or dihydrorhodamine by neutrophils. Prophylactic antibiotics, particularly co-trimoxazole, which is concentrated in neutrophils, can significantly reduce morbidity and mortality from bacterial infections. Oral antifungal prophylaxis with an agent such as itraconazole probably reduces the incidence of fatal fungal disease.
Infections or unexplained fevers should be treated aggressively. IFN-γ is a useful adjunctive treatment in severe bacterial or fungal infections. White cell infusions may also be used as adjunctive therapy in severe infection. Despite these therapeutic advances, considerable morbidity and mortality still occur, and HSCT should be considered ( ).
Other neutrophil disorders
Neutropenia
Neutropenia may result from reduced production in the bone marrow or from increased peripheral destruction, distinguished by the findings on bone marrow examination. It most frequently follows decreased production induced by disease processes or drug treatments, as well as sepsis in preterm or stressed neonates. Increased consumption may occur in autoimmune states, including neonatal alloimmune neutropenia and those associated with immune deficiency. The degree of neutropenia will influence the clinical picture: neutrophil counts of less than 0.5 × 10 9 /l carry a major risk of infection, whilst counts below 0.2 × 10 9 /l are associated with a significant risk of life-threatening sepsis.
Severe congenital neutropenia (Kostmann’s syndrome)
This AR disease was originally described by . Onset is within the first year of life with recurrent and life-threatening infections. Symptoms include cellulitis, perirectal abscesses, peritonitis, stomatitis and meningitis. Bone marrow examination shows an arrest at the promyelocyte to myelocyte maturation stage. Kostmann’s syndrome is due to mutations in Hax1, but defects in other genes can lead to a similar clinical picture ( ).
Shwachman–Diamond syndrome
This rare AR disorder is characterised by exocrine pancreatic insufficiency, skeletal abnormalities, bone marrow dysfunction and recurrent infections. Neutropenia occurs in all patients, whilst 10–25% of patients also have pancytopenia. There is an increased incidence of malignancy.
Treatment of neutropenia with G-CSF results in increased counts and fewer infections in almost all patients with neutropenia not secondary to peripheral destruction. Concerns about the induction of leukaemias with prolonged use have not been realised, although pretreatment and annual bone marrow aspirates are recommended. BMT may be indicated in selected cases.
Haemophagocytic lymphohistiocytosis
Congenital neutropenia or neutrophil dysfunction, with or without associated hypopigmentation, is a feature of diseases with defects in proteins that control lysosomal secretion in melanocytes and immune cells. An important presentation of these diseases is haemophagocytic lymphohistiocytosis (HLH), a fatal inflammatory disease characterised by high swinging fevers, hepatosplenomegaly, jaundice and erythematous rash, respiratory distress and pancytopenia, consequent to hypercytokinaemia and organ infiltration by CD8+ lymphocytes and macrophages. Patients appear septic but blood cultures are usually sterile. Laboratory findings include an acute-phase response, elevated ferritin and elevated fasting triglycerides. Examination of bone marrow, cerebrospinal fluid, pleural effusions or ascitic fluid may demonstrate haemophagocytosis. Haemophagocytosis may occur secondary to a number of infections, in particular viral infections, and careful exclusion of infections by serology/polymerase chain reaction should be undertaken. Haemophagocytosis is seen in a number of immunodeficient states, including Chédiak–Higashi syndrome, Griscelli syndrome and X-linked lymphoproliferative disease (XLP) ( Table 39.2 ). Chédiak–Higashi syndrome is a rare AR disease with partial oculocutaneous albinism, recurrent bacterial infections and a high risk of developing HLH, which is usually fatal. Variable neurological manifestations are also recognised. Characteristic giant lysosomal granules are seen in the cytoplasm of all cells containing these organelles, and are easily detected on a peripheral blood film. Individuals with Griscelli syndrome resemble those with Chédiak–Higashi syndrome in that they have variable hypopigmentation of the skin and hair and recurrent pyogenic infections associated with absent delayed-type cutaneous hypersensitivity and impaired NK cell function. Hypogammaglobulinaemia can be seen as a secondary phenomenon. In contrast to Chédiak–Higashi syndrome, large lysosomal granules are not seen, and examination of the hair by electron microscopy shows large clumps of pigment, with the accumulation of mature melanosomes in melanocytes. Neurological abnormalities are absent in these patients. They are also at high risk of developing macrophage activation syndrome unless treated by BMT.
Leukocyte adhesion deficiency
Inherited defects in leukocyte cell surface molecules can prevent their egress into infected tissue, resulting in a characteristic clinical presentation. In leukocyte adhesion deficiency type I, deficiency of the 95-kD β chain (CD18), common to the β2 integrin family of cell surface adhesive molecules, leads to a profound immunodeficiency affecting the function of neutrophils, monocytes and certain lymphocytes, including T and NK cytotoxic cells ( ). Inheritance is AR. Chemotaxis, adherence and phagocytosis are markedly depressed. The phenotype varies according to the severity of the mutation. Leukocytes are attracted to areas of infection and become fixed to the vessel walls at sites of inflammation in the usual way but cannot pass out into the tissues, causing infarction of small vessels and rapidly expanding necrotic lesions without pus.
Individuals with the most severe phenotype (<1% expression) present in the first weeks of life with delayed umbilical cord separation (the cord fails to shrink down and may not separate until 3–4 weeks of age) and rapidly progressive erosive perianal ulcers. Delayed umbilical cord separation does not occur in patients with some expression of the molecule (usually in the range 2–10% of normal expression). In all forms there is excessive susceptibility to bacterial and fungal infections. Gingivitis and periodontitis are common, and more deep-seated infections of bone, respiratory tract and gastrointestinal tract are often seen. Non-infective inflammatory lesions, particularly affecting the skin and resembling pyoderma gangrenosum, can occur in the partial forms of the deficiency and are often responsive to steroids.
There is almost always a neutrophilia (because of failure of the cells to migrate out of the circulation) and a profound neutrophil chemotactic defect. Diagnosis is confirmed by demonstrating the absence of the cell surface markers recognised by anti-CD11/CD18 monoclonal antibodies.
In the severe form, early death from infection occurs unless a successful BMT can be performed. In the partial forms, supportive and expectant management is pursued in the first instance, but BMT may become necessary.
Hyper-IgE syndromes
These complex disorders are characterised by extreme elevation of the serum IgE level (usually in the range 2000–40 000 U/l), chronic dermatitis and repeated lung and skin infections ( ). Patients present in infancy with eczema, but in the neonatal period a vesicular rash may be present in the first few days of life. Staphylococcus aureus , the predominant pathogen, can cause pneumatoceles and chronic lung damage. Recurrent candidiasis may be a feature. Treatment is long-term antistaphylococcal antibiotic prophylaxis, usually with flucloxacillin. Three genes have been identified, leading to the clinical picture. Defects in STAT3 give rise to the classical autosomal-dominant clinical picture, whereas AR defects in TYK2 and DOCK8 are associated with severe molluscum contagiosum and herpesvirus infection.
Anhydrotic ectodermal dysplasia, incontinentia pigmenti and defects in the NEMO gene
Until recently, incontinentia pigmenti and X-linked anhydrotic ectodermal dysplasia were thought to be unrelated disorders. Incontinentia pigmenti is a rare X-linked dominant condition characterised by developmental abnormalities of the skin, hair, teeth and central nervous system. Affected female infants present with a characteristic rash, occurring in four phases of erythema and vesicles, hyperkeratosis, hyperpigmentation and finally pallor and atrophy. Previously thought lethal in male fetuses carrying the affected X chromosome, rare male infants with a progressive combined immunodeficiency have been described. Patients with X-linked anhydrotic ectodermal dysplasia present with sparse scalp hair, conical teeth and absent sweat glands. Some suffer from recurrent sinopulmonary infection, often with encapsulated organisms, and have poor antibody responses to polysaccharide antigens, or frank hypogammaglobulinaemia. Hypofunctional mutations in the NEMO gene encoding a protein required to activate the transcription factor NF-κB have been described in male patients with both incontinentia pigmenti and X-linked anhydrotic ectodermal dysplasia, suggesting that these conditions represent variants of the same disorder ( ). Other proteins in the same signalling pathway can cause a similar clinical picture, although inheritance is AR.
Congenital asplenia
Asplenia is seen when congenital cardiac defects are associated with right isomerism, and the presence of Howell–Jolly and Heinz bodies on a blood film should raise suspicion, asplenia being confirmed by abdominal ultrasound. Asplenic infants are at lifelong risk from invasive infection by organisms with polysaccharide coats, such as group B streptococcus and pneumococcus. Treatment is with lifelong prophylactic antibiotics. Penicillin is the antibiotic of choice, although in children less than 5 years of age we advocate using co-trimoxazole in order to prevent infection with the greater range of pathogens, including Haemophilus influenzae and Gram-negative organisms, to which younger children with asplenia are susceptible. Vaccination with conjugate pneumococcal and quadravalent meningococcal vaccines is recommended before 2 years of age, and thereafter with polysaccharide pneumococcal and meningococcal vaccines.
Treatment of immunodeficiency
Vaccination of the child with a potentially impaired immune response
Immunocompromised children are more in need of the protection vaccination can offer because of their greater risk from infection, and yet are less likely to be vaccinated because of concerns about the underlying immunodeficiency. Children with primary immunodeficiency should be immunised. Live vaccines should be avoided in those with T-lymphocyte-mediated immunodeficiency; all other forms of immunodeficiency can safely have live vaccine administered, except BCG in patients with CGD. Preterm infants should be vaccinated routinely, following the national immunisation programme. Children on pharmacological doses of steroids (an equivalent dose of prednisolone 2 mg/kg/day for >1 week, or 1 mg/kg/day for >1 month) or other immunosuppressive therapy should not be given live vaccines until 3 months after ceasing therapy ( ). At-risk infants should receive passive immunisation with appropriate antigen-specific immunoglobulin.
Supportive care
Children with immunodeficiency disorders often require the full spectrum of paediatric care. Particular attention needs to be paid to nutritional status and the management of dietary intolerances secondary to the gastrointestinal problems which frequently occur. Advice from paediatric gastroenterologists and respiratory paediatricians should be sought early, to minimise the impact of the disease on gut and lungs and to maximise supportive therapy. Supporting the emotional needs of the family is also very important. Prevention and treatment of infections are mainstays of supportive care.
Specific measures for prevention of infections
Co-trimoxazole as prophylaxis against Pneumocystis jiroveci should be given for defects involving cell-mediated immunity mechanisms. For PCP prophylaxis only, co-trimoxazole need only be given on 2 or 3 days a week. If this is not tolerated, alternatives include dapsone and atovaquone. Co-trimoxazole probably also reduces the incidence of pyogenic infections in these patients and those with phagocytic or humoral immune deficiencies, and for this purpose it is usually given daily (dose 30 mg/kg/day in one or two doses). In circumstances of poor nutrition, increased bone marrow turnover and in all cases after BMT, weekly folinic acid supplements are given to lessen the risk of bone marrow depression without compromising the antimicrobial efficacy. Antifungal prophylaxis should be used in combined immunodeficiencies or phagocytic cell defects. In CGD and other conditions where the risk of Aspergillus infection is high, itraconazole is preferred. Otherwise, fluconazole or non-absorbed agents such as nystatin are used. Antiviral prophylaxis with aciclovir is used in patients with cell-mediated immunodeficiency and previous herpes simplex infection. It is also used in the context of BMT to prevent herpes simplex and CMV reactivation.
Treatment of infections
A policy of vigorous and early antimicrobial treatment of infections should be observed. Unusual agents may cause infection, and broader-spectrum antimicrobial cover may therefore be needed. In neutrophil disorders, it is particularly important to cover Pseudomonas and other Gram-negative bacilli. Early treatment of an infection may be life-saving, but its initiation should not detract from full attempts at identification of the causative agent. Invasive diagnostic procedures, such as bronchoalveolar lavage or, if this fails to produce a diagnosis, open-lung biopsy, can be fully justified on the basis that they will facilitate precise and optimal therapy. The mainstay of treatment for systemic fungal infection remains amphotericin B, mostly used in its liposomal form to reduce toxicity and enable larger doses to be given. Promising new agents for treating invasive aspergillosis include voriconazole and caspofungin.
Antivirals such as the broad-spectrum agent ribavirin and the anti-CMV agents ganciclovir and foscarnet are particularly useful in the immunocompromised infant. Cidofovir is useful against resistant CMV, adenovirus and severe molluscum contagiosum.
Replacement immunoglobulin
Immunoglobulin is a blood product, and so its use is associated with all the risks of giving such products, such as hypersensitivity reactions (including anaphylaxis) during administration. Although modern manufacturing processes include steps which screen for and inactivate viruses such as hepatitis B and C, and HIV, transmission of infectious agents remains theoretically possible. Particular current concerns revolve around the issue of transmission of prion disease, although the risk of this is probably very small. Such factors mean that immunoglobulin treatment should be initiated only after due consideration of the risks and benefits, and in line with published national guidelines ( ). Before administration, liver function tests should be measured, and a sample of serum taken for long-term storage in case of future infective complications which may be attributable to the immunoglobulin. Many studies have examined the role of immunoglobulin in either the prophylaxis or treatment of infection in preterm or low-birthweight (LBW) infants. Taken overall, whilst IVIG prophylaxis gives a small (4–5%) reduction in the incidence of sepsis or serious infection, there is no difference in mortality, and only a trend towards shorter hospital stay. This has to be balanced against cost, risk of side-effects (including potential transmission of infection) and recurrent venous access. There is no justification for the routine use of IVIG as prophylaxis against Gram-negative infection in preterm or LBW infants ( ).
IVIG has also been advocated as an adjunctive treatment to antibiotics in sepsis or suspected sepsis. A recent meta-analysis showed a borderline statistically significant reduction in mortality in cases of suspected sepsis given immunoglobulin, although the difference was more significant in cases that were proven sepsis ( ). Immunoglobulin treatment for this indication is not recommended in the current national guidelines, and should only be administered in the context of a clinical trial. Pathogen-specific immunoglobulin such as palivizumab may have a role in preventing respiratory syncytial virus-associated pneumonitis in at-risk infants, particularly those with impaired T-lymphocyte immunity.
Granulocyte colony-stimulating factor
Septic sick or preterm neonates become neutropenic because neutrophil stores are rapidly depleted, and granulopoiesis is depressed. G-CSF mobilises preformed neutrophils from the marrow into the circulation, and enhances neutrophil precursor proliferation. Several studies have examined the effect of administering G-CSF to septic infants. Other studies have addressed the issue of using G-CSF as prophylaxis by prospectively stimulating neutrophil production. There is no evidence to support these treatment modalities ( ). G-CSF has a role in treating infants with congenital neutropenia.
Blood product support
All blood products except IVIG and fresh frozen plasma contain viable leukocytes, and so before being given to patients with combined immunodeficiency or to those receiving intense immunosuppression should be irradiated with 25–50 Gy to prevent possible GvHD ( ). In those with no evidence of previous exposure to CMV, blood products should be obtained from CMV antibody-negative donors. White cell infusions can benefit patients with CGD and other neutrophil disorders with invasive infections who respond poorly to antibiotic treatment, particularly if the leukocytes are harvested from G-CSF-primed donors.
Haematopoietic stem cell transplantation
Most primary T-lymphocyte and phagocyte immunodeficiency disorders can be corrected by HSCT, using stem cells from marrow, mobilised peripheral blood stem cells or those harvested from umbilical cord blood ( ). Best results are obtained using HLA-matched sibling donors, but these are available for only about 20% of patients. Since the early 1980s, techniques have been developed to allow HLA-mismatched parent-to-child (haploidentical) grafts, by removing mature T lymphocytes that would otherwise cause fatal GvHD. Broadly matched volunteer-unrelated donor transplants are being increasingly used, particularly for non-SCID immunodeficiencies, and peripheral blood or umbilical cord stem cells are increasingly used instead of bone marrow. Gene therapy has been used for selective specific disorders, and clinical trials are ongoing ( ).
Infection in the newborn
- Alison Bedford Russell
- David Isaacs
In spite of significant advances in neonatal intensive care and the development of broad-spectrum antibiotics, sepsis remains a leading cause of morbidity and mortality in the newborn, especially those born prematurely ( ; ) and with low birthweight ( ; ; ).
The incidence of sepsis is inversely proportional to birthweight. In very-low-birthweight (VLBW) infants, infection is associated with significant risk of death and morbidity, including prolonged ventilation and hospital stay, and increased risk of chronic lung disease (CLD) ( ; ; ), interventricular haemorrhage and death ( ). Perinatal infection has also become recognised as an important aetiological factor in the pathogenesis of cerebral cortical lesions ( ) and subsequent neurodevelopmental delay in both preterm ( ; ) and term infants ( ; ; ). Chemical mediators of this damage include proinflammatory cytokines, e.g. tumour necrosis factor-α (TNF-α) and interleukin-6 (IL-6), as well as free radicals generated by activation of the neutrophil respiratory burst ( ). Infection has been shown to induce damaging activation of glial cells, with infiltration of macrophages, leading ultimately to neuronal apoptosis ( ). High concentrations of proinflammatory cytokines are associated with an increased risk of cardiovascular compromise, septic shock and death ( ; ). Proinflammatory cytokines also have effects on the developing brain during sepsis episodes, and are strongly associated with the development of brain lesions, even in the absence of meningitis ( ; ).
The origins of nosocomial infection related to feeding practices
The gut of a baby admitted to a neonatal unit becomes colonised with abnormal bacteria as a result of obstetric practices, exposure to the neonatal unit bacterial flora and antibiotic administration. Recent evidence points to the huge importance of specific components of the gut flora in programming both intestinal function and immunological responses (both mucosal and systemic) in the host ( ; ; ; ). Thus abnormalities of initial colonisation patterns induced by preterm delivery, caesarean section and antibiotic administration may have potential delayed effects upon the infant, although so far there has been little focused study of this ( ).
Faecal flora in preterm babies is dominated by coagulase-negative staphylococci (CoNS) ( ), which are the most common organisms causing late-onset sepsis (LOS) ( ; ; ), and enteric Gram-negative bacilli ( ). It is likely that bacteria from the gut translocate across immature gut mucosa ( ; ) as the gut mucosa presents a poor barrier to infection, particularly in a non-fed newborn baby. Translocating bacteria may colonise indwelling devices such as catheters and give rise to systemic infection ( ).
The amniotic fluid-induced growth and development of the fetal gut are interrupted by preterm birth. Unit practices, such as delaying the introduction of maternal breast milk, may reduce the ability to undergo postnatal adaptation, and compromise normal immune development. Timing of introduction of colostrum is likely to be a key factor in postnatal intestinal development. Animal studies have demonstrated that delaying the introduction of milk feeds for even 2–3 days is associated with significant gut atrophy ( ; ; ) and necrotising enterocolitis (NEC) ( ). Formula feeding is associated with an increased risk of gut atrophy, reduced gut function and NEC-like lesions ( ; ).
Because abnormal gut colonisation patterns may have very broad effects on immune development ( ; ) and postnatal gut adaptation, delaying the introduction of human milk feeds may also contribute to an increased risk of infection. The introduction of any milk feed may be delayed for up to a week or more as a result of concerns regarding medical stability and the risk of necrotising enterocolitis (NEC) in particular ‘high-risk’ groups such as those with intrauterine growth retardation (IUGR). However this practice in babies has not been validated ( ; ; ), except for babies receiving formula feeds; the giving of human breast milk has not been associated with increased risk of NEC ( ; ). Indeed, breast milk has only ever been shown to protect babies from NEC ( ; ). Breast milk has also been shown to protect babies from late-onset infection ( ; ; ; ).
Conversely, the introduction of even very small quantities of oral nutrients prevents intestinal mucosal atrophy and bowel villi flattening ( ; ). This effect is most marked following exposure to colostrum ( ; ; ). In vitro studies have demonstrated increased intestinal mass and deoxyribonucleic acid synthesis rates as a result of gut exposure to human breast milk ( ). Epidemiological studies indicate that the risk of infection is greatly increased for every day a baby is not enterally fed ( ; ; ; ). Conversely the earlier enteral feeds are commenced, and the sooner a baby is receiving full enteral feeds, the less likely that baby is to develop nosocomial (late-onset) infection and NEC ( ; ). In one study of babies <1000 g, early breast milk feeding (initiated in 98% of babies within 3 days of life) was the most significant factor in reducing the risk of nosocomial sepsis, without increasing the risk of NEC ( ). Indeed, the incidence of all NEC was low, at a rate of 4% (published ranges 5.6–7.2%: ; ). The effect of early feeding is independent of the increased risk of infection with indwelling catheters (long lines, umbilical venous and arterial lines), and total parenteral nutrition (TPN) ( ; ). However the nutritional needs of a baby have to be balanced against risk of infection, so that almost all babies <1 kg are likely to require at least a short period of TPN to ‘feed the brain’, and this is most safely delivered by a central venous catheter.
Introducing even 0.5 ml/kg of trophic maternal breast milk (and only breast milk) in the first hours of life at infrequent intervals of up to daily, in very immature babies, will facilitate the colonisation of the naïve gut with normal bacteria (lactobacilli and bifidobacteria). Although many of the potential benefits of trophic feedings still require confirmation by good clinical studies, clinical benefits may include improved milk tolerance, greater postnatal growth, reduced systemic sepsis, reduced NEC risks and shorter hospital stay ( ). There is currently no evidence of any adverse effects following trophic feeding with human breast milk ( ).
Recent findings emphasise the role of the normal flora in conditions such as NEC, and have shown benefit from use of probiotic organisms ( ; ). The links between trophic feeding, breast milk, the gut flora and the mucosal immune system are complex but highly important.
Neonatal skin and infection
Neonatal skin has innate immune functions, and provides a physical barrier, albeit fragile, as well as serving as a repository of antimicrobial proteins. In utero, the human fetus is encased in vernix caseosa and bathed in amniotic fluid, both of which are highly enriched with host defence proteins (HDPs) such as lysozyme and lactoferrin ( ; ; ). The latter permit a homeostatic balance between the presence of normal commensal bacteria and deterrence of pathogenic organisms ( ; ). In the immediate postnatal period an acid mantle covering the skin surface is rapidly established, and may enhance host defence mechanisms against pathogenic bacterial colonisation and infection ( ). There has been much debate about the potential harmful effects versus the presumed benefits of bathing newborns ( ; ; ; ). Bathing may remove HDPs in vernix as well as reduce pathogen load ( ; ). The World Health Organization (WHO) recommends that newborns should not be bathed for at least 6 hours after birth to prevent deleterious effects of hypothermia ( ). Term newborn skin is replete with HDPs, which may not be significantly affected by a single bath ( ), but may be affected by frequent bathing with soap. Skin colonisation on the neonatal intensive care unit (NICU) is mainly by CoNS, which can be isolated from over 90% of all positive cultures ( ).
A clearer understanding of the role of HDPs in the cutaneous innate immune system, and of the impact of neonatal skin adaptation and skin care practices, such as bathing and the use of soap, will be important in the quest to reduce newborn infections. The benefits of frequent bathing with soap need to be properly evaluated in order to avoid potential harm.
Incidence of infection and causative organisms
Neonatal infection surveillance networks
When a neonate has suspected sepsis, antibiotic therapy is empirical, and should be commenced without delay, before knowing the exact nature of the infecting organism and its antimicrobial susceptibility. Since the aim of empirical therapy is to target the most likely infecting organism(s), it is crucial for neonatal units to survey the profile of causative organisms and their susceptibilities, in order to direct antimicrobial treatments effectively. Microbial pathogens, their antimicrobial sensitivities and patterns change over time ( ; ; , ) and between countries ( ; ).
Neonatal infection surveillance networks have been established in a number of countries, including the Australasian Study Group for Neonatal Infections (ASGNI) ( ), the Israeli National VLBW Infant Database ( ), the Canadian Neonatal Network ( http://www.canadianneonatalnetwork.org ), the US National Institute of Child Health and Human Development Neonatal Research Network ( http://www.nichd.nih.gov.easyaccess2.lib.cuhk.edu.hk ), the Vermont Oxford Network ( http://www.vtoxford.org/home.aspx ), and the German Krankenhaus-Infektions-Surveillance-Systäm ( ). Apart from the ASGNI and Canadian groups, these neonatal infection networks focus on infection rates in VLBW babies.
In the UK, a Neonatal Infection Surveillance Network (NeonIN) was established in 2004 to collect clinical and microbiological data on episodes of neonatal infection longitudinally. The incidence, pathogens and antibiotic resistance profiles of infections captured in NeonIN in the 3 years between January 2006 and December 2008 have recently been reported ( ).
Additionally the Health Protection Agency has a voluntary surveillance scheme in England and Wales, whereby bacteraemias but not clinical data are reported. The British Paediatric Surveillance Unit has also continued to conduct studies of the epidemiology of infections over defined time periods, such as herpes simplex virus (HSV) ( ), group B streptococcus (GBS) ( ), meticillin-resistant Staphylococcus aureus (MRSA) ( ), fungal infections in VLBW babies ( ) and neonatal meningitis ( ), which have provided invaluable data. Surveillance of human immunodeficiency virus (HIV) infections in children is ongoing, and current specific studies include surveillance of congenital syphilis and congenital rubella.
A variety of data are collected on culture-proven sepsis, as well as presumed and possible sepsis ( ). Definitions of sepsis vary between surveillance networks but all consist of a combination of clinical and laboratory parameters ( ). These networks have been able to monitor changes in pathogens ( ) and their antibiotic resistance over time ( ; ), so can be used to inform antibiotic policy. They provide data for comparisons between different countries, and have been used to document changes in clinical practice and their effects ( ; ), and provide a system to identify what constitutes effective practice, and thus improve quality of care ( ). Surveillance systems can also be used to provide data for the planning of interventional studies, as well as systems for data capture, following interventions ( ; ).
Incidence of infection
The UK NeonIN data demonstrate that, with the inclusion of CoNS, the incidence of all neonatal infection is 0.8% of live births and 7.1% of neonatal admissions. The incidence of culture-confirmed neonatal sepsis in the USA is in the region of 0.7% ( ). One study in Australia reported an incidence of 0.22% for early-onset sepsis (EOS) and 0.44% for late-onset sepsis (LOS), with mortality rates 15% and 9% respectively ( ). In resource-poor countries, neonatal sepsis rates are as high as 5–10%, with case-fatality rates of between 23% and 52%. Septicaemia accounts for between 11% and 30% of all neonatal deaths ( ).
Classification of neonatal infections
Neonatal infection is broadly classified into early- or late-onset neonatal sepsis.
Early-onset sepsis (EOS) is variably defined as sepsis (e.g. bacteraemia and meningitis) within 48–72 hours of birth (some define it as <7 days) and usually presents as a fulminating septicaemic illness, often complicated by pneumonia. The main routes of infection are vertical transmission from the mother via transplacental or ascending vaginal routes, and postnatally from the environment. Other terms used for this pattern of infection are ‘vertically transmitted’ (meaning from mother to infant) and ‘perinatally acquired’. The pattern of presentation is similar with most of the causative bacteria.
Late-onset sepsis
Late-onset infection in neonates is defined as infection becoming clinically evident more than 48–72 hours after birth, and is usually the result of nosocomially acquired organisms, hence the term ‘healthcare-acquired infection’.
Early-onset sepsis
The risk of sepsis and mortality increases with decreasing gestational age and birthweight and is highest in babies <1000 g. The reported incidence of EOS in VLBW infants ranges from 1.5% ( ) to 2.7% ( ), with high case-fatality rates of 26–36% ( ).
The overall incidence of EOS in the UK is 0.9/1000 of all live births and 0.9% of all neonatal admissions. GBS and Escherichia coli are the most common causative organisms, if CoNS are excluded, and account for 58% and 18% of bacteraemias respectively in the UK ( ; ).
In the USA, widespread universal screening for maternal GBS colonisation was introduced in the mid-1990s, and intrapartum antibiotic prophylaxis (IAP) was adopted as routine practice. Since then, there has been a significant decline in GBS-EOS ( ; ), and an accompanying change in the distribution of pathogens, from predominantly Gram-positive organisms to Gram-negative Enterobacteriaceae, particularly E. coli ( ; ; ; ). In the USA, there has also been an increase in frequency of multiply resistant E. coli isolates in VLBW infants ( ). The reason for this shift in causative organisms, although tentatively linked to IAP use in VLBW babies ( ), is uncertain since many factors affect the maternal vaginal flora (including intrapartum antibiotic treatment, both in the community and in hospital, ethnic group, underlying medical condition and age). In Australia, the introduction of IAP for GBS prophylaxis has not been accompanied by the same increase in resistant Gram-negative infections ( ). Recent studies in the UK report national incidence rates of GBS infection in infants similar to those seen in the USA following the introduction of universal screening and IAP ( ). Hence in the UK the National Screening Committee has not recommended the introduction of a UK GBS screening programme. Pathogens associated with EOS are summarised in Table 39.4 .
USA: NICHD 2002–2003 Incidence/1000 VLBW | UK: NeonIN 2007–2008 Incidence/1000 VLBW | P | |
---|---|---|---|
All infections | 17 | 22 | |
Gram-positive | 8 | 17 | 0.002 |
Group B streptococcus | 2 | 11 | 0.06 |
Listeria | 0.3 | 5 | |
CoNS | 2 | 8 | 0.005 |
Other | 3 | 3 | |
Gram-negative | 9 | 4 | 0.002 |
Escherichia coli | 7 | 3 | 0.02 |
Other | 2 | 0.4 | |
Fungal | |||
Candida albicans | 0.3 | 0.4 |
Group B streptococcus
GBS is an important cause of serious infection in neonates, infants and immunocompromised or pregnant adults. The overall incidence of GBS infection in the UK is 0.72/1000 live births, though there are regional variations, with the highest incidence being in Northern Ireland and the lowest in Scotland ( ). GBS is a commensal of the gastrointestinal tract and vagina, and colonises some 10–30% of pregnant women in the UK. There are numerous identifiable serotypes of GBS, based on capsular polysaccharide antigens: Ia, Ib, II, III, IV, V, VI, VII and VIII. All are implicated in early-onset disease, with national variations in prevalence. In the UK, enhanced surveillance has demonstrated that GBS serotypes III, Ia and V are the most commonly identified isolates causing early-onset infection ( ). Simultaneous infection with two distinct serotypes has been described ( ). In addition to the polysaccharide antigens there are surface-exposed protein antigens which may also contribute to the pathogenicity of particular strains ( ).
It is well established that GBS can cross intact fetal membranes. While prolonged rupture of fetal membranes (>18 hours) is a feature in 44% of newborns with invasive GBS infection, for 56% of affected babies, membrane rupture occurred less than 18 hours before birth ( ). Although GBS infection is uncommon, mortality and morbidity are high, especially for preterm babies ( ). This may partly be a consequence of the acute inflammatory response to GBS. GBS is able to invade pulmonary endothelial cells, leading to release of eicosanoids such as prostacyclin and prostaglandin E2 ( ). In animal models, GBS causes a dose-dependent increase in pulmonary arterial pressure and pulmonary and systemic vascular resistance, and decreases cardiac output and heart rate. The quantitative response of the pulmonary vascular resistance is GBS strain-dependent in animal models, with serotype Ib having a greater effect than serotype III ( ). Postmortem examination demonstrates that many infants develop alveolar hyaline membranes, as well as pneumonia ( ). In the preterm infant, this is likely to represent coexistent surfactant-deficient hyaline membrane disease of prematurity ( ), but in term babies the hyaline membranes are a non-specific change associated with GBS infection ( ), and may reflect a secondary surfactant insufficiency.
Most incidence figures are based on ‘culture-proven’ GBS whereby GBS is grown in blood or cerebrospinal fluid (CSF). Various factors may decrease the yield of blood and CSF cultures in the presence of true infection. Blood cultures are often negative in pneumonia ( ). Other possible explanations include prior use of antibiotics (e.g. when given to the mother as IAP) ( ), inadequate blood volumes obtained ( ) or low-level bacteraemia. In the case of GBS, ‘probable’ infection has been defined as an episode of clinical sepsis likely to have been caused by GBS, and indicated by GBS surface colonisation together with clinical features of sepsis but with negative blood cultures. Prospectively collected data in a UK tertiary neonatal unit from all neonates requiring a sepsis screen in the first 72 hours of life demonstrated a combined rate of definite and probable GBS infection of 3.6/1000 live births ( ). This suggests a much greater disease burden in the UK than the incidence based on culture-proven sepsis.
Prior to the introduction of a national GBS-screening programme and IAP, the reported prevalence of early-onset GBS sepsis in the USA was 1.8 per 1000 live births ( ); in Canada, 1.75 per 1000 ( ); in Australia, 1.3 per 1000 ( ); and in Spain, 1.2 per 1000 ( ). The prevalence in Finland is around 0.76 per 1000 ( ).
Risk factors for early-onset GBS infection can be identified in up to 60% of cases and include preterm delivery (<37 weeks), prolonged rupture of membranes (PROM ≥ 18 hours) or known genital carriage of GBS during pregnancy ( ), although GBS can cross intact membranes. Infection is the most common identifiable reason for spontaneous vaginal preterm delivery, so preterm delivery alone represents a risk factor for infection. The overall mortality is approximately 10% but is significantly higher among infants born prematurely ( ). Up to 7% of survivors of GBS infection may suffer some disability; the risk is obviously higher if there is meningitis. Opportunities to prevent GBS infection using a risk factor based approach to GBS-prevention are often missed ( ).
The mode of presentation and frequency of infection differ according to age at onset: GBS most commonly causes early-onset disease, defined as being <7 days (0.47/1000), manifesting as sepsis or pneumonia. Late-onset GBS disease can occur from 7 days to 3 months of life and is less common (0.25/1000); the more frequent presentation is with meningitis and sepsis. Late-onset GBS infection may result from invasion of colonising organisms acquired at birth or nosocomially or possibly from breast milk ( ; ).
GBS colonisation
GBS resides in the lower gut and vagina in 12–23% of mothers ( ; ; ). Such women are described as being ‘carriers’ of GBS or ‘colonised’ with GBS. GBS is passed from one person to another by skin-to-skin contact and can be exchanged between mother and father through sexual contact. However, there are no known harmful effects of carriage itself and, since the GBS bacteria do not cause genital symptoms or discomfort, GBS is not a sexually transmitted disease. Neither is GBS carriage a sign of ill health or poor hygiene. The likelihood of GBS isolation increases if samples are obtained from the lower vagina and rectum ( ) and placed in selective broth, and decreases if the sample is limited to a high vaginal swab.
Babies are far more frequently ‘colonised’ than ‘infected’ with GBS. If a term baby is well, has a normal examination (specifically a normal respiratory rate and heart rate) and no other risk factors for infection, the baby is most likely simply to be colonised with GBS. The National Institute for Health and Clinical Excellence (NICE) intrapartum care guideline recommends monitoring of general well-being, temperature, perfusion, respiration rate, pulse and heart rate for a minimum of 12 hours in babies whose mothers had PROM at term ( ) for management of babies at risk for GBS infection (see Fig. 21.1 in Ch. 21 for a suggested observation chart). A preterm baby, especially one delivered after spontaneous onset of labour, warrants an infection screen and treatment with antibiotics, including penicillin, until cultures are known to be negative. This is because infection is the most common identifiable reason for spontaneous preterm delivery, as stated above.
Presentation of GBS infection
Nearly 90% of neonates with early-onset GBS have signs of infection within 12 hours of delivery ( ; ). Early stages of infection may be very subtle. A baby may simply not be feeding well or be excessively sleepy. Tachypnoea, apnoea or other respiratory distress are the most common presenting signs ( ; ). GBS sepsis may alternatively present with respiratory failure, cyanosis and shock, and the baby’s condition at delivery may be clinically indistinguishable from perinatal hypoxic ischaemia. GBS infection should always be suspected in a baby who has more severe respiratory distress syndrome (RDS) than would have been anticipated, as RDS and GBS pneumonia are radiologically indistinct. In severe cases, persistent pulmonary hypertension of the newborn, hypotension, metabolic acidaemia, tachycardia and poor peripheral perfusion may develop and are poor prognostic features.
If infection is suspected in a baby, the baby should be promptly investigated (including a chest radiograph (CXR)), and treated with antibiotics. C-reactive protein (CRP) measurements may be normal in the early stages of infection ( ) as it takes at least 12 hours for a CRP level to become raised ( ). A normal CRP does not exclude GBS infection, but may rise after 12–24 hours in infection. A raised CRP may be due to infection or other causes of inflammation. Some use the CRP to monitor the response to therapy, but there is no evidence that this is superior to clinical response.
Benzylpenicillin is the antibiotic of choice for GBS. Any organisms present in blood usually require a minimum of 48 hours to culture. During this time babies are treated with antibiotics until the results of the cultures are known. It is not uncommon for a baby to have signs of infection but for the tests on blood, swabs or CSF to be negative. Pneumonia is the most common focal infection caused by GBS, and, as already stated, can mimic RDS radiologically, with bilateral ground-glass shadowing and air bronchograms ( Fig. 39.7 ). Signs of pneumonia may be minimal and only manifest on a radiograph and with clinical signs and raised inflammatory markers (raised CRP, alterations in absolute neutrophil count (ANC) and low platelet count). It is common practice to treat such babies with antibiotics for many days because of the difficulty in being certain about whether or not bacteria are causing their illness ( ). As a result not only do many infants receive antibiotics that are ultimately not required, but they may also receive them for a long time. In the prospective study referred to above ( ), <5% of infants treated for infection had either culture-proven or culture-negative clinical sepsis; thus >95% of babies receiving antibiotics did not need them. Excessive antibiotic use may alter antibiotic susceptibilities as well as the types of colonising microbial flora, especially in the gut. Possible consequences include the emergence of antibiotic resistance in common bacterial pathogens, such as E. coli ( ; ; ), the emergence of new pathogens, such as Enterobacter sp. ( ), and an increased risk of atopy and asthma in childhood ( ; ; ).

Recurrent GBS infection
Reccurrent GBS sepsis occurs in <1% of infants. In a series of nine cases ( ), the mean age at second infection was 42 days; six cases had septicaemia without a focus of infection and three had meningitis. The organism causing the first and subsequent infection was shown to be the same in more than half of the cases. Whether these cases represent a relapse of the initial infection or a reinfection is unclear. Rifampicin has been used, with limited success, to eradicate carriage in some children with recurrent GBS infection.
Detection of GBS with PCR
There are a number of studies in progress to evaluate polymerase chain reaction (PCR) as a diagnostic tool in the detection of GBS and E. coli in neonates undergoing sepsis screens in the first 72 hours of life. PCR can be used to detect specific bacterial proteins in body fluids and swabs. Such methods do not depend on having to culture bacteria. In animals PCR has been shown to result in a significantly more rapid detection rate of organisms than conventional culture methods ( ; ; ).
In future, PCR may be able to detect bacteraemia in cases of clinical sepsis with a greater sensitivity and frequency than is currently detected by conventional blood cultures. Furthermore for infants with GBS or E. coli bacteraemia, diagnosis will be made in hours rather than days.
Improvements in diagnostic techniques may thus better inform antibiotic management of the newborn ( ). Negative results should enable clinicians to have confidence in prescribing antibiotics for short periods of time, or not at all.
Prevention of GBS
In the absence of a vaccine, the most effective strategy for prevention of GBS disease is to give antibiotic prophylaxis to mothers during labour. In the USA, where GBS was very much more common than in the UK, all mothers have a vaginal swab to look for GBS at 35–37 weeks’ gestation. GBS swab-positive mothers are treated with a penicillin (usually ampicillin) during labour. This has resulted in a significant reduction in babies with invasive GBS disease. In the UK, approximately 625 women with one or more risk factors (intrapartum fever, PROM >18 hours, prematurity <37 weeks, previous infant with GBS) need to be treated to prevent one case of disease, and 5882 women need to be treated to prevent one death ( ). There is increasing concern that unnecessary use of antibiotics is associated with an increase in infections with resistant bacteria ( ). IAP may therefore be useful in the short term, but in the long term may facilitate the development of resistant Gram-negative organisms, which result in as much, if not greater, harm. Routine screening and antibiotic prophylaxis are not currently recommended in the UK ( ; ). Studies of GBS vaccines which may prevent GBS infection in the future are promising, but vaccine development is in the early phases, and at present there is no GBS vaccine available ( ).
Listeria
Listeria monocytogenes is a short Gram-positive intra- and extracellular rod. The most important reservoir for transmission to humans is probably food, especially dairy products, contaminated by infected farm animals ( , ). Neonatal infection in the UK is now uncommon ( Table 39.4 ). Women infected with HIV are considerably more susceptible to L. monocytogenes than are the general population ( ). Three main types of fetal and neonatal infection are found: (1) transplacental infection; (2) early-onset infection acquired intrapartum; and (3) late-onset infection, usually meningitis, probably due to nosocomial infection.
Transplacental infection
L. monocytogenes causes a non-specific influenzal or gastroenteritic illness in the pregnant woman, during which the organism may infect the fetus, either by haematogenous spread across the placenta or via infection of the amniotic fluid. First- or second-trimester infection may cause fetal death or miscarriage, and recurrent abortion due to Listeria has been recorded in humans ( ). Later in pregnancy, infection may precipitate preterm labour ( ), with spontaneous PROM, and fetal distress. Meconium staining of the liquor is not specific to Listeria infection ( ). Liveborn babies are often extremely ill at birth. They may have severe pneumonia, and hepatosplenomegaly and meningitis may already be present. Blood and stool cultures are invariably positive. Characteristically, small (2–3 mm) pinkish-grey cutaneous granulomas are present and, at autopsy, similar small granulomatous lesions are widespread in the lung, liver, central nervous system (CNS) and many other tissues and organs.
Early-onset infection, acquired intrapartum
Most cases of neonatal listeriosis are sporadic, but epidemics have been described. The mother of a case is likely to be carrying the organism in her stool and presents an infection risk in a maternity hospital. She and her baby should be isolated. At least two-thirds of infants who acquire Listeria infection intrapartum are preterm, and almost all become ill within 24 hours of birth ( ). Most have disseminated infection, with pneumonia, meningitis, thrombocytopenia, anaemia and sometimes conjunctivitis. Small cutaneous granulomas may be found in some babies.
Late-onset infection, usually meningitis, probably due to nosocomial infection
Nosocomial spread of Listeria is well documented, and asymptomatic mothers of affected babies should be isolated, as discussed above ( ; ). Late-onset Listeria infection is usually in the form of meningitis with or without concomitant septicaemia or colitis. The median age of onset is about 2 weeks. Associated brain abscess has been described ( ).
Investigation
The routine investigations of EOS and meningitis reveal the diagnosis. However, Listeria can pose problems for the microbiologist, as its uptake of the Gram stain can be variable and it may be slow-growing.
Therapy
The most effective antibiotic therapy is ampicillin plus gentamicin, although Listeria is sensitive to benzylpenicillin. Listeria is resistant to all third-generation cephalosporins.
Outcome
The mortality for neonatal listeriosis ranges between 5% and 15% ( ; ).
Late-onset sepsis
The majority of LOS occurs in premature (<37 weeks’ gestation) and low-birthweight (<2500 g) babies, the risk being greater for the smallest and most immature babies ( ; ; ; ; Makhoul et al. 2002; ; ; ).
The incidence of LOS among VLBW infants ranges between 16% and 30% ( ; ; Makhoul et al. 2002; ; ) and approaches 50% in infants with a birthweight <1000 g (Makhoul et al. 2002; ). Mortality rates vary between 17% and 21% ( ; ). The incidence of LOS in the UK is 0.7% of live births and 6.1% of neonatal unit admissions.
Organisms
The most common organisms causing late sepsis in industrialised countries are CoNS, which comprise 45–55% of isolates ( ; ; ; ) ( Table 39.5 ). CoNS predominate amongst gut flora of babies in neonatal units ( ; ) and may translocate across gut mucosa ( ) or invade via skin surfaces or indwelling catheters. CoNS can cause sepsis but are also frequent contaminants of blood and CSF cultures. Epidemiological studies vary in their definition of true CoNS infection and contaminants ( ; ). In one study, more than half of late-onset CoNS-positive neonatal bacteraemias were considered to be true infections ( ). Rapid time (≤36 hours) to blood culture positivity is a surrogate for organism load and has been used as a marker of true infection ( ). CoNS rarely cause fulminant infection or death, and empiric antibiotic regimens that include vancomycin to cover CoNS are no more effective than those that use a semisynthetic penicillin such as flucloxacillin ( ). That said, in the largest study of outcomes in extremely low-birthweight (ELBW) infants, CoNS were the predominant isolates, and all adverse neurodevelopmental outcomes, except hearing impairment, were higher among infected children regardless of type of pathogen ( ). This may be because the babies with Gram-negative bacillary infection were more likely to have died.
USA: NICHD 1998–2000 n = 1313 Incidence/1000 VLBW | UK: NeoNIN 2007–2008 n = 524 Incidence/1000 VLBW | Germany NeoKISS 2000–2005 n = 1844 Incidence/1000 VLBW | |
---|---|---|---|
All infections | 21 | 24 | 21 |
Gram-positive | 15 | 18 | |
CoNS | 10 | 13 | 6.6 |
Staphylococcus aureus | 1.6 | 1.9 | 1.2 |
Enterococcus | 0.7 | 2.0 | 0.5 |
Gram-negative | 3.7 | 4.4 | |
Escherichia coli | 1.0 | 1.6 | 0.6 |
Enterobacteriacae | 1.8 | 1.9 | |
Pseudomonas | 0.6 | 4.0 | |
Candida spp. | 2.6 | 0.8 | 0.4 |
Selection pressure due to intensive use of antimicrobials in neonatal units has led to changes in antimicrobial susceptibility patterns of organisms associated with LOS. Changes in the epidemiology of nosocomial infections and outbreaks of MRSA ( ), ampicillin-resistant E. coli , vancomycin-resistant enterococci and multiresistant Gram-negative bacilli are increasingly being reported from neonatal units. This is a major concern, especially since resistant Gram-negative bacteria make the choice of empirical therapy more difficult, and result in a poorer prognosis for the patient treated with antibiotics to which the organisms are resistant ( ).
Other isolates causing LOS include Enterobacteriaceae (9–21%) and Staphylococcus aureus (13–18%, 11% of which were MRSA), E. coli (Health Protection Agency, HPA), GBS (HPA) and fungi 9% (72% C. albicans ).
Systemic fungal infection has become a major problem for ELBW infants <1000 g, with published infection rates varying widely between countries at 1.5–20%.
Enterobacteriaceae
The family Enterobacteriaceae includes many genera and species ( Table 39.6 ). Members are Gram-negative, facultatively anaerobic bacilli, which may appear as coccobacilli on Gram stain. The virulence of these species is controlled by a number of factors, including the ability to adhere, colonise, invade tissues and produce various toxins. Different antigens can be used to differentiate different serologic groups. The capsular antigen K1, present in certain strains of E. coli , has been the most documented virulence factor associated with E. coli meningitis and sepsis. These strains are distinct from those associated with diarrhoeal disease. Some Enterobacteriaceae harbour plasmids providing antimicrobial resistance genes. An increasing number of E. coli and Klebsiella strains produce plasmid-mediated extended-spectrum β-lactamases, which can inactivate cephalosporins, penicillins and aztreonam. Family members are ubiquitous in nature but almost all share the gastrointestinal tract as a common niche, and, apart from Yersinia , Shigella and Salmonella , can be normal resident flora if confined to their natural environment. E. coli is so commonly isolated from colonic flora that it is used as a primary marker of faecal contamination in water purification. Although Enterobacteriaceae can cause opportunistic infection, they are often harmless commensals. Members are broadly divided into opportunistic and primary pathogens. Opportunistic pathogens are often part of the normal faecal flora. They may produce serious extraintestinal opportunistic infections, however; for example E. coli is a member of the normal bowel flora but can cause urinary tract infections (UTIs), septicaemia and meningitis. Primary pathogens include Salmonella , Shigella and Yersinia , and are not part of normal gut flora. They are true pathogens and will cause infections if ingested.
|
Gram-negative bacteraemia and antimicrobial non-susceptible Enterobacteriaceae
The Centers for Disease Control National Nosocomial Infection Surveillance System has reported a substantial increase in third-generation cephalosporin-resistant enteric Gram-negative bacilli causing nosocomial infections in intensive care units ( ; ). Gram-negative bacteraemia in children has also been positively associated with the use of antibiotics in addition to cephalosporins, including aminoglycosides and vancomycin, but also correlates with surgical interventions, central venous catheters, parenteral nutrition, antacids and dexamethasone. The strongest association in one study was with the use of vancomycin ( ), hence the recommendation that vancomycin should not be used for empirical treatment on the basis of suspicion of bacteraemia.
ELBW babies, <1000 g, who are colonised with antimicrobial non-susceptible Enterobacteriaceae (ANE) and those who receive prolonged exposure to antimicrobial agents are at increased risk of ANE infections ( ). Such ANE include E. coli, Enterobacter spp., Citrobacter spp., Klebsiella spp. and Serratia spp. They are defined as being ‘members of the Enterobacteriaceae family exhibiting non-susceptibility to ceftazidime or with evidence of extended spectrum beta-lactamase production’. Multiply resistant Enterobacteriacaeae strains (MRE, defined as ‘strains resistant to three or more classes of antibiotic’), have been found at high frequency in NICU infants, and persist in a significant proportion of infants for at least 6 months, even after discharge from hospital ( ). Such high prevalence of colonisation increases the probability of MRE infection in vulnerable infants and outbreaks are increasingly described within NICUs ( ; ; ; ; ; ).
Colonisation with MRE strains has been detected as early as on the first day of hospitalisation ( ), and may follow transmission from other NICU patients, or may evolve endogenously from selective pressures exerted by antimicrobial therapy.
In the UK, the majority of pathogens, other than CoNS, causing EOS (>94%) are susceptible to commonly used empiric first-line antibiotic regimens of penicillin/gentamicin or amoxicillin, amoxicillin combined with cefotaxime or cefotaxime monotherapy ( ; ). More than 95% of organisms causing LOS are susceptible to gentamicin with either flucloxacillin ( ; NeonIN), or amoxicillin and amoxicillin with cefotaxime, but only 79% are susceptible to cefotaxime monotherapy ( ). This supports the use of narrow-spectrum antimicrobials in the form of penicillin/gentamicin as ‘first-line’ therapy for EOS and flucloxacillin/gentamicin for LOS, with use of vancomycin restricted to situations where there is a serious risk of CoNS infection.
Supoptimal empiric antibiotic therapy potentially leads to worse outcomes ( ; ), but must be balanced against the hazards of routine use of broad-spectrum antibiotics for empiric therapy, since administration of these correlates with the development of resistant organisms ( ; ).
Enterococci
Previously classified as group D streptococci, enterococci are Gram-positive cocci that are natural inhabitants of the human intestinal tract. They have a survival advantage over other organisms in that they can grow in extreme conditions and are resistant to multiple antimicrobial agents. Enterococci are associated with nosocomial infection, most commonly bacteraemia and UTI. Prolonged hospitalisation, immunocompromise and prior surgical procedures are risk factors. Because of intrinsic or acquired resistance to several antimicrobial agents, including aminoglycosides, beta-lactams and glycopeptides, e.g. vancomycin and teicoplanin, differentiation of enterococci from other streptococci and susceptibility testing are important.
Fungal infection
Systemic candidiasis is a leading cause of death and morbidity, specifically poor neurodevelopmental outcome, in ELBW infants ( ). The incidence of Candida infection in ELBW babies in a recent surveillance study of 13 USA centres varied between 2% and 28% ( ), with an overall incidence across 19 units of 9%. Recent UK data estimated the annual incidence of invasive disease to be 1.0% (0.8–1.2%) amongst VLBW infants and 2.1% (1.65–2.57%) in ELBW infants ( ). The vast majority of cases are of late onset (>72 hours after birth). Mortality rates range from 25% to 40%, which is higher than those for bacterial infection. Risk factors include birthweight <1500 g, TPN, presence of indwelling catheters, not receiving enteral feeds, mechanical ventilation, exposure to broad-spectrum antibiotics, especially third-generation cephalosporins, and exposure to antenatal antibiotics ( ), H 2 receptor antagonists, abdominal surgery and peritoneal dialysis ( ).
Signs and symptoms may be very non-specific and include: temperature instability; respiratory distress; lethargy, apnoeas with or without bradycardia; abdominal distension, bilious aspirates and blood in stools mimicking NEC, but without pneumatosis coli; and glucose intolerance.
Clinical presentation, investigation and management of neonatal sepsis
Clinical presentation and assessment of the infant
Early recognition, diagnosis and treatment of serious infection in the neonate are essential because of the risk of permanent morbidity or mortality. Progression from mild symptoms to death can occur in less than 24 hours. Clinical signs may be subtle in the early stages of bacteraemia, but this is when treatment must be started if there is to be intact survival. This leads to a tendency to overinvestigate and overtreat. Over 95% of infants screened and treated for early-onset infection had no evidence of infection in one study ( ). However, the consequences of procrastination when infection is the cause of signs and symptoms can be fatal.
History
It is important to ask specific questions about maternal, perinatal and neonatal events. Apparently trivial episodes of pyrexia or skin rash may be significant, as well as contact with known cases of infectious disease; whether a mother was unwell or febrile; had a tender uterus or a vaginal discharge; the exact time of membrane rupture; potentially infectious lesions on her cervix or vulva; presence of a cervical suture; fetal tachycardia; raised maternal CRP or white cell count. The smaller and more preterm a baby, the higher the risk of infection and mortality ( ).
If the mother has overt intrapartum infection, 15.2% of preterm babies and 4.1% of term babies will develop infection. Maternal UTI is an important risk factor for neonatal sepsis.
Factors predisposing to nosocomial infection include a history of possible contact with an infected person or environment, not receiving enteral feeds ( ; ), indwelling catheters or receiving TPN ( ; Shinwell et al. 2004). Babies who have had gut surgery or gut-related problems also have a significant risk of nosocomial sepsis. Infection is more likely to occur in a baby in intensive care, and infection will prolong hospital stay and morbidity ( ; ; ; ).
Signs of neonatal sepsis
In the early stages, signs may be subtle and, although difficult to define, a mother or nurse may report that a baby is simply ‘ not right’. Isolated tachypnoea (respiratory rate sustained above 60 breaths/min and slight recession) and feeding difficulties (not feeding or poor suck) are the most frequent early signs of infection.
Temperature
After excluding the effect of pyrexia in the first 1–2 hours following birth in a baby of a pyrexial mother, which may be associated with epidural anaesthetic and an abnormal environmental temperature, temperature below 36°C or above 37.8°C, sustained for more than an hour, must be regarded as probably due to infection until proved otherwise. An unremitting fever is likely to be viral in origin.
Irritability
Infection may cause pain and may make the baby restless or whimper.
Skin
Petechiae may be present, or there may be septic spots, paronychia or omphalitis. Poor cutaneous circulation is indicated by mottling and delayed capillary filling (>3 seconds).
Jaundice
Unexplained jaundice may result from the effect of increased haemolysis.
Cardiovascular
Tachycardia of 160 beats/min may be a sign of sepsis and may be more marked in cases of myocarditis or endocarditis.
Gastrointestinal
The baby may vomit, have mild diarrhoea, or may develop an ileus with associated abdominal distension. The differential diagnosis includes NEC and structural malformations of the gastrointestinal tract.
Respiratory
Apnoea, cyanosis, grunting and dyspnoea are all signs of respiratory distress. Pneumonia is a possibility, but many other diagnoses are also possible, including generalised sepsis, meningitis and cardiac disease. Persistent moaning respiration is an ominous early sign.
For ventilated babies, an increase in ventilation requirements often accompanies generalised sepsis as well as pneumonia,
Central nervous system
A high-pitched cry, neck retraction, bulging fontanelle and convulsions are late features of neonatal meningitis.
Haemorrhagic diathesis
Disseminated intravascular coagulation (DIC) with petechiae and bleeding from puncture sites, the gut or the renal tract is a late sign of sepsis. Thrombocytopenia without evidence of DIC is a more common feature of infection and NEC.
Sclerema
This is often associated with Gram-negative infection but may be a non-specific feature of any serious neonatal illness ( Box 39.1 ).
It is important to examine the completely naked baby in a warm environment, as subtle signs will otherwise be missed. Inspect for the following:
- •
Tone – is the baby floppy?
- •
Irritability and responsiveness
- •
Signs of dehydration
- •
Colour and lesions of the skin, e.g. erythematous umbilicus or subcutaneous tissues, mottling (with cutis marmorata)
- •
Fontanelle tension, spinal column and skull for pits or other skin defects, which might be the entry site for meningeal infections
- •
Signs of respiratory distress, such as tachypnoea or grunting, recession, abnormal breath sounds
- •
Heart rate and pulses, and note pulse volume. Murmurs or a triple rhythm may suggest congenital cardiac disease or myocarditis or endocarditis
- •
Abdomen for distension, rigidity, masses and bowel sounds
- •
Hepatosplenomegaly, which may accompany generalised septicaemia as well as hepatitis
- •
Kidney enlargement
- •
Limbs for signs of osteomyelitis and septic arthritis
Investigation of neonatal sepsis
Any baby with a history and examination suggestive of infection should be promptly evaluated, investigated and treated if there is any suspicion that infection could be present. Although antibiotics are administered to a considerable number of non-infected infants ( ), a bacteraemic baby with early features of sepsis may deteriorate rapidly if not treated. New diagnostic methods, including PCR, continue to be evaluated but none so far has been of sufficient worth to replace conventional blood culture and clinical acumen. A number of additional investigations may provide information to support or refute a diagnosis of sepsis.
Microbiological investigation
Blood culture remains the ‘gold standard’ for diagnosing infection, though it has limitations ( ). The majority of significant blood cultures are positive by 48 hours ( ).
Collecting blood for culture
Blood for culture should be drawn from a freshly punctured blood vessel using strict aseptic technique and a closed system. The greater the volume of blood, the higher the yield of organisms, but 0.5 ml of blood is usually sufficient for a successful culture ( ). Taking two cultures from separate sites reduces false-positive diagnoses of infection.
Antibiotic therapy should be stopped after 36–48 hours if cultures are negative and there are no further signs of infection. Alternatively, when there is a suspicion that clinical progress is suboptimal, consideration should always be given to an empiric change of antibiotic therapy to include a broader spectrum of pathogens. Such analyses should be validated in other data sets but have the potential to improve neonatal outcome by ensuring appropriate antibiotics are used as early as possible. Once a bacterium is identified, the antibiotic regimen should be targeted appropriately.
Surface swabs, tracheal secretions, endotracheal tube tip culture and gastric aspirates
Routine swabbing of sites such as the umbilicus, groin, ear, nose, throat, pharynx and rectum are informative about colonisation, but numerous studies have shown that the results of surface cultures are of limited value in diagnosis ( ; ). Colonisation of babies, for example of endotracheal secretions or of skin and mucosal surfaces, without clinical signs of infection does not warrant antibiotic treatment ( ). The same applies to gastric aspirates and maternal vaginal swabs which are indicative of colonisation but not invasive infection. The latter may be helpful in a baby who is symptomatic of infection if positive for GBS.
Urine
A UTI is defined by there being a pure growth of at least 10 8 organisms per litre of urine (10 5 /ml) in a specimen of urine. In order to avoid the problems of contamination of urine specimens, suprapubic aspiration of urine or fresh catheter urine specimens should be obtained in the initial assessment of the sick baby who is thought to be septicaemic, though this may be impracticable. A clean-catch sample may be helpful if the genitalia have been adequately cleaned. The only value of ‘bag urines’ is that if they are clear they exclude UTI. Positive bag urines, even when the genitalia have been adequately cleaned, should always be viewed with suspicion, even in the presence of white cells, as babies may have sterile pyuria.
Vascular lines and thoracocentesis tubes
The tips of umbilical cannulae, central lines and thoracocentesis tubes are usually sent for culture, though they have a limited role for diagnosing infection as they are indicative of colonisation, including of the skin through which they are withdrawn.
Lumbar puncture
A lumbar puncture should be performed as part of the sepsis screen in most ill babies before antibiotics are started. Exemptions include babies with acute RDS ( ), pulmonary infection complicating long-term intermittent positive-pressure ventilation (IPPV), babies with overt localised infection, such as NEC or pneumonia, and babies who would not be able to tolerate the procedure ( ). A low platelet count is a contraindication to lumbar puncture; if the investigation is essential a platelet transfusion should be given first. Asymptomatic term babies undergoing investigation because of risk factors for sepsis do not require a lumbar puncture, nor is jaundice requiring treatment in a well baby an indication for lumbar puncture ( ; ). Meningitis is more frequently a feature of LOS than EOS ( ; ).
Lumbar puncture must be performed with strict sterile precautions, avoiding excessive flexion of the trunk, which may otherwise cause respiratory embarrassment or apnoea. It is safer to intubate and ventilate a very unstable baby before proceeding.
CSF analysis
High white blood cell counts in CSF have sometimes been reported in babies without meningitis, but a polymorphonuclear count higher than 20/mm 3 should be regarded with suspicion ( ), and counts above 30/mm 3 are strongly indicative of meningitis ( Appendix 8 ). For blood-stained CSF the ratio of red cells to white cells should be 500 : 1 in uninfected CSF.
The upper normal limit of CSF protein is 1.5–2.0 g/l in the term baby ( Appendix 8 ). Preterm babies may have higher CSF protein concentrations but rarely greater than 3.0 g/l. Protein levels are usually raised in meningitis. CSF glucose levels should be compared to a simultaneous measurement of blood glucose, and should be at least 50% of the blood glucose level. A low level, less than 30% of the blood glucose, suggests bacterial meningitis.
The CSF should be Gram-stained, examined under a microscope, and cultured for bacteria. Other tests which may be indicated include PCR tests for GBS, herpesvirus and enteroviruses.
Radiology
All babies with suspected sepsis should have a CXR. An abdominal radiograph may also be helpful in the differentiation between septic ileus and NEC. Radiographs may not detect bone or joint infection in the early stages.
Haematological investigation
Total white cell count
This is the least useful index, because the normal range is so wide ( Appendix 1 ), varies with gestation and postnatal age, can be confused by machines including nucleated red blood cells in the count, and can be 30–40% lower in blood obtained from a central catheter than in a capillary sample. Furthermore, the white blood cell count is raised in many non-infective conditions such as periventricular haemorrhage, convulsions and hypoxic–ischaemic encephalopathy (HIE).
Neutrophil count
Normal ranges for neonatal ANC are different from those of infants and children, and there are a variety of published ranges. The most widely used reference ranges for normal neonatal neutrophil counts are derived from those published by . These data are limited by the small numbers of babies of lower gestational age, with very few of 26 weeks’ gestation or lower. Revised Manroe reference ranges have been published by and . Although both neutropenia and neutrophilia (<5 × 10 9 /l or >20 × 10 9 /l respectively) have useful predictive power ( ), in neither case is the specificity or sensitivity greater than about 80%.
The ANC in babies born small for gestational age are lower than those born at weights appropriate for gestational age and may take up to 2 weeks of age to normalise ( ).
Similarly, infants born to mothers with pregnancy-induced hypertension (PIH) are more frequently neutropenic and may be at higher risk of infection ( ; ; ). The mechanism for this is thought to be related to there being a circulating inhibitor of granulocyte (G)-CSF in mothers with PIH ( ). Such infants are also more likely to be thrombocytopenic ( ).
Immature circulating neutrophils, known as band forms, are seen in the peripheral blood in response to infection. In the most severely affected infants band cell production becomes limited as the marrow becomes exhausted. There is a high mortality rate in neonates who fail to mount a neutrophil response to infection ( ; ).
The I/T ratio may be useful in diagnosing and monitoring infection ( ). The maximum normal value is 0.16 during the first 24 hours, 0.14 by 48 hours and 0.13 by 60 hours, where it remains until 5 days of age. Thereafter, the maximum normal I/T ratio is 0.12 until the end of the first month. Several studies have found that an I/T ratio of ≥0.2 is a useful marker of infection ( ). Another feature suggesting infection is the presence of toxic granulation in the neutrophils. found this in only 11% of normal infants, compared with 63% of infants with confirmed sepsis.
Lymphocyte count
Babies who have lymphocyte counts persistently below 2.8 × 10 9 /l should be investigated for severe combined immunodeficiency ( ).
Platelet count
In 50% of babies with bacterial infection, the platelet count will fall below 100 × 10 9 /l.
Thrombocytopenia is a common feature of NEC and infection but may also be a feature of non-infective conditions such as HIE. Thrombocytopenia as well as neutropenia is a feature of IUGR and PIH ( ; ; ). Viral infections, both congenital (e.g. rubella, cytomegalovirus (CMV) and herpes) and acquired (e.g. enterovirus, CMV, herpes), may cause a profound thrombocytopenia. Conversely, thrombocytosis can be a manifestation of chronic inflammation, particularly within the gut.
Abnormal liver function tests, jaundice or a bleeding tendency are further indications of viral infection, though they can also be features of bacterial infection.
Acute-phase proteins
C-reactive protein
CRP levels rise after inflammatory mediators such as IL-6 stimulate synthesis in the liver ( ). It is not uncommon for babies with positive blood cultures to have negligible CRP levels at birth but the CRP rises some 12 hours or more later. CRP may also rise in response to apparently non-infective inflammation such as with HIE ( ; ; ; ), which is consistent with data demonstrating elevation in IL-6 concentrations in babies with HIE ( ).
Intrauterine infection and inflammation giving rise to a fetal inflammatory response, and resulting in elevated IL6 in fetal plasma (and thus elevation of CRP), have been related to white-matter injury and cerebral palsy ( ; ). While there may be rises in CRP as a result of HIE, autopsy findings suggest congenital infection may mimic perinatal asphyxia, but are missed diagnostically, in the absence of placental histology and autopsy ( ).
Severe hypoxic respiratory failure has been associated with raised blood concentrations of CRP, IL-6 and IL-8 and a greater incidence of histological chorioamnionitis and funisitis ( ), which suggests that inflammation, whether infective or non-infective, contributes to the severity of hypoxic respiratory failure.
Meconium-stained liquor is by no means specific for infection ( ), yet may be associated with inflammation and hence a CRP response. Some babies never mount a CRP response. This may be related to genetic polymorphisms in the immune response ( ; ; ).
Serial measurements of CRP may be useful in monitoring the progress of infection or other inflammatory conditions such as NEC, and may help to guide treatment. Persistently elevated CRP during antibiotic therapy for presumed bacterial infection suggests ongoing infection or inflammation.
Serum procalcitonin
Procalcitonin is the prehormone of calcitonin, which is normally secreted by thyroid C cells, and rises within 3–6 hours of exposure to infection. Elevated serum concentrations of procalcitonin appear to be more sensitive and specific in the differentiation between neonatal infection and inflammation than CRP ( ) and may also differentiate between bacterial and viral infection ( ). Procalcitonin measurements are undertaken by some laboratories as routine clinical tests.
Cytokines
TNF-α and IL-6
Elevations of plasma TNF-α and IL-6 concentrations in plasma may provide an early indication of sepsis, but levels may also be raised as a result of non-infective inflammation. Plasma IL-6 but not TNF-α has been shown to increase in term newborns with HIE, and both CSF (TNF-α) and CSF (IL-6) are significantly higher in babies with HIE compared with sepsis and controls ( ). Because of difficulties in excluding any contributing infection without autopsy ( ), the exact mechanism of rises in proinflammatory cytokines in non-infective inflammatory conditions is unclear. IL-6 may be a better marker of EOS than CRP; however, these cytokine assays have largely been used in research ( ) and have not been introduced into routine clinical practice.
IL-8 is also an early indicator of sepsis, being involved in neutrophil release from bone marrow, and neutrophil activation.
Serum granulocyte colony-stimulating factor
Neonates are able to produce endogenous, immunoreactive G-CSF which is measurable in the plasma, and levels are inversely related to ANCs, but the relationship is variable. As with many other measurable cytokines and growth factors, plasma G-CSF is variably raised at birth. Plasma G-CSF rises in response to infection and inflammation, but responses are no more sensitive or specific then CRP measurements, and thus should not be relied upon as a marker of infection or inflammation ( ).
Mannose-binding lectin
Mannose-binding lectin (MBL) is a protein of the innate immune system which activates complement after binding to repeating mannose units present on a number of different pathogens. Binding results in opsonisation and individuals with low MBL levels are associated with a decreased ability to opsonise bacteria and hence an increased susceptibility to infection. MBL2 gene polymorphisms are present on approximately one third of white people, and thus deficiency of MBL presents a potential risk factor for infection. In a recent review of MBL studies, newborns with low MBL levels had a greater risk of culture-positive sepsis then those with normal MBL levels, but the results for MBL2 genotype and infection were contradictory ( ).
Immunological tests
Antigen detection tests
Rapid screening for GBS using latex particle agglutination is widely used, for detecting both maternal and neonatal colonisation ( ). The main value of the test may be in providing reassurance of the absence of GBS.
Antibody detection tests
These are of more value in viral infections, when a fourfold or greater rise in antibody titre in samples drawn 2 weeks apart is diagnostic. If there is suspicion of congenital infection, organism-specific immunoglobulin M (IgM) should be sought.
Genetic techniques
It is now possible to amplify highly conserved DNA sequences from a variety of Gram-positive and Gram-negative organisms, as well as many viruses, using PCR, while avoiding the simultaneous amplification of associated human DNA. This method has the potential to be automated and to provide rapid diagnosis of bacteraemia.
In a meta-analysis of various intrapartum group B streptococcus colonisation tests, the most accurate was the real-time PCR test, although it took 10 minutes longer to perform PCR as compared to the Optical immunoassay (OIA) test (40 versus 30 minutes) ( ) ( Box 39.2 ).
- •
End-organ evaluation to include ( ):
- •
Abdominal ultrasound (including renal ultrasound)
- •
Cerebral ultrasound
- •
Lumbar puncture
- •
Fundoscopy
- •
Echocardiogram
- •
- •
Blood cultures at 24–48-hourly intervals to ensure clearance
- •
Suprapubic or catheter specimen of urine
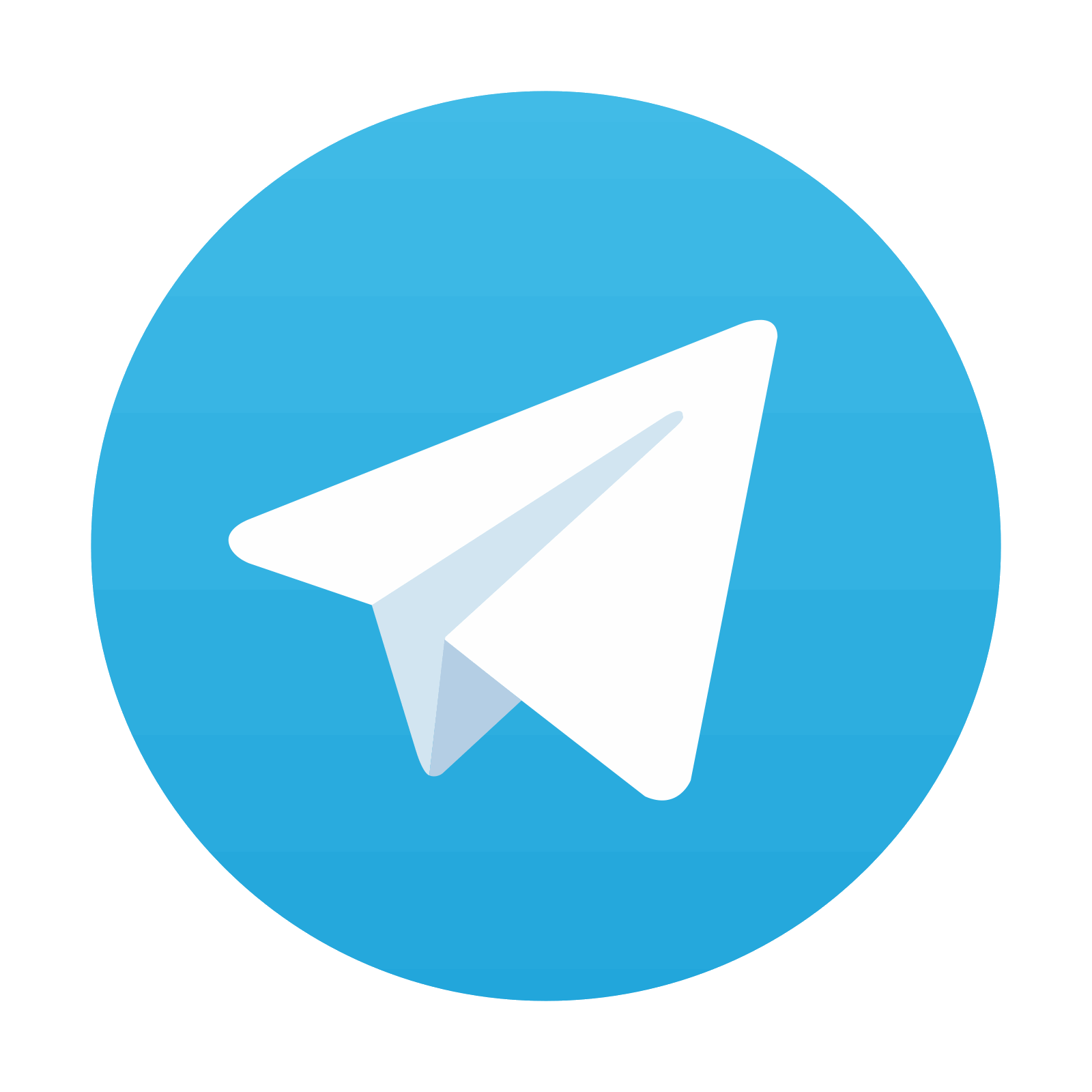
Stay updated, free articles. Join our Telegram channel
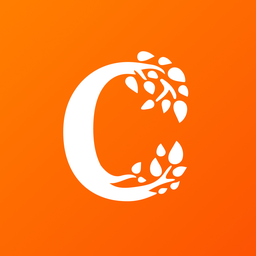
Full access? Get Clinical Tree
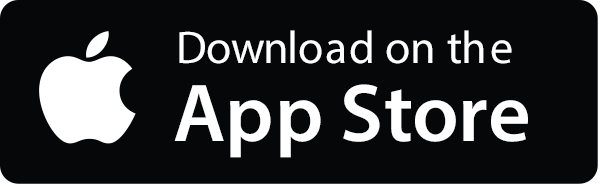
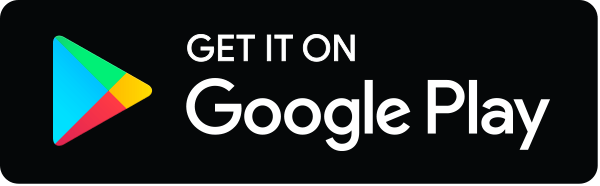