Despite the availability of a wide variety of antimicrobial agents and expansion of vaccination programs, infectious diseases remain a leading cause of childhood morbidity and mortality worldwide. A number of factors contribute to the increasing importance of infectious agents: rates of antimicrobial resistance continue to rise, global travel has become routine, and the number of individuals with altered immunity has increased. Furthermore, in recent years, microorganisms have been implicated in diseases previously considered noninfectious, and a variety of new, emerging, and reemerging pathogens have been recognized.
Pathogens are defined as microorganisms that are capable of causing disease. However, not all pathogens are equal with respect to their pathogenic potential (i.e., their virulence). Many pathogens are, in fact, commensal organisms that live in harmony with their host under most conditions, causing disease only when normal immune mechanisms are disrupted or absent. Other pathogens produce disease even in the setting of intact host defenses and almost always cause symptoms.
For a given microbe, pathogenic potential is often determined by the genomic content and regulation of virulence-associated genes. Some bacterial species are capable of natural transformation and readily acquire fragments of DNA from other organisms, thus expanding or altering their genetic composition, occasionally with consequences related to virulence or antimicrobial resistance. A number of microorganisms carry virulence-associated genes on mobile genetic elements, including plasmids, transposons, and bacteriophages. These elements may equip the organism with genetic information that facilitates rapid adaptation to an unfavorable or changing environment. Comparison of genomes from pathogenic and nonpathogenic bacteria within a single genus or species has led to the identification of pathogenicity islands , which are large blocks of chromosomal DNA that are present in pathogens and absent from related nonpathogens. These blocks are flanked by insertion sequences or repeat elements and differ in nucleotide composition relative to the surrounding genome, suggesting acquisition by horizontal exchange. Pathogenicity islands in bacteria encode a variety of virulence factors, including protein secretion systems, secreted effector molecules, adhesins, and regulatory proteins. In an analogous way, some viral pathogens such as influenza virus are capable of exchanging nucleic acid segments with other viruses, leading to changes in pathogenicity, host tropism, and transmissibility.
To be successful, a pathogen must enter the host, occupy an appropriate niche, and then multiply. Sometimes the pathogen will induce damage to the host and then spread to other tissues, either near the initial site of infection or more distant. Often the pathogen will stop short of causing death to the host, maintaining latent infection or producing symptoms such as cough or diarrhea that facilitate spread to another host. This chapter addresses several key steps in the pathogenic process, each illustrated with examples of pathogens and paradigms of relevance to infectious diseases in children.
Colonization
Most bacterial infections begin with microbial colonization of a host surface, typically the skin, the respiratory tract, the gastrointestinal tract, or the genitourinary tract. Although colonization is not sufficient for an organism to produce disease, it is a necessary prerequisite. The process of bacterial colonization requires specialized microbial factors, called adhesins, that promote adherence to host structures and enable these organisms to overcome local mechanical defenses such as mucociliary function, peristalsis, and urinary flow. The cognate receptors for these interactions are generally either carbohydrate or protein structures, in some cases expressed on host cells and in other instances present in mucosal secretions or in submucosal tissue.
Pilus Adhesins
Perhaps most common among bacterial adhesins are hairlike fibers called pili (also called fimbriae). Pili are heteropolymeric protein structures comprised largely of a major subunit usually ranging in size from 15 to 25 kDa. Because of their size and morphology, most pili can be visualized by negative-staining transmission electron microscopy.
The prototype example among adhesive pili is the P (or Pap) pilus, which is expressed by uropathogenic Escherichia coli (UPEC) and has been strongly associated with pyelonephritis. P pili recognize globoseries glycolipids, which are host molecules that are characterized by a core structure consisting of Gal-α1,4-Gal. The globoseries glycolipids are especially abundant in renal epithelium, thus accounting for the predilection of P-piliated E. coli to adhere to kidney tissue and cause pyelonephritis. Type 1 pili are analogous fibers expressed by UPEC and bind mannosylated uroplakin proteins in the mammalian bladder to initiate cystitis. As shown in Fig. 1.1 , P pili are composite structures and consist of two subassemblies, including a thick rod that emanates from the bacterial surface and a thin tip fibrillum that extends distally. The pilus rod is a right-handed helical cylinder and is composed of repeating PapA subunits, whereas the tip fibrillum has an open helical configuration and contains mostly repeating PapE subunits. The two subassemblies are joined to each other by the PapK adaptor protein. PapG contains the adhesive moiety and is located at the distal end of the tip fibrillum, joined to PapE by the PapF adaptor.

P pili are assembled through a canonical process termed the chaperone-usher pathway that involves a periplasmic chaperone (PapD) and an outer membrane usher (PapC) (see Fig. 1.1 ). Subunit proteins (e.g., PapA) are translated in the bacterial cytoplasm, enter the periplasm through the inner membrane Sec machinery, and are stabilized by interaction with the chaperone, which ferries them to the usher. Extrusion of the nascent fiber is controlled by a “plug” domain in the usher pore; because the periplasm is devoid of adenosine triphosphate (ATP), the assembly process is energetically driven by the entropically favorable final conformation of the incorporated subunits. More than 30 different bacterial adhesive structures are assembled via this chaperone-usher pathway, with distinct PapD-like chaperones and PapC-like ushers. The PapD-like chaperones can be divided into two distinct subfamilies based on conserved structural differences that occur near the subunit binding site. One subfamily is involved in the assembly of rod-like pili similar to P pili, whereas the second subfamily participates in the biogenesis of more atypical filamentous structures, such as Caf1 of Yersinia pestis (the plague bacterium), which forms an amorphous “mat” on the bacterial surface. Thus the nature of the chaperone is directly correlated with the architecture of the adhesive appendage that it helps to assemble.
Type 4 pili represent a second class of pili and are distinguished by a methylated first amino acid (usually phenylalanine); a short, positively charged leader sequence; a conserved hydrophobic N-terminal domain; and a tendency to form bundle-like structures. Type 4 pili have been identified in a number of gram-negative bacterial pathogens, including Neisseria gonorrhoeae, N. meningitidis, enteropathogenic E. coli (EPEC), Vibrio cholerae, Pseudomonas aeruginosa, Kingella kingae, Eikenella corrodens, Haemophilus influenzae , and Moraxella species. Although the mechanism of assembly of type 4 pili is still being elucidated, existing data suggest that the process is complex. For example, between 20 and 40 gene products are required for the assembly of P. aeruginosa type 4 pili, and at least 15 plasmid-encoded proteins are involved in the biogenesis of EPEC type 4 pili. Based on studies of P. aeruginosa, EPEC, Neisseria, and V. cholerae , the presence of an inner membrane prepilin peptidase appears to be a general prerequisite for type 4 pilus biogenesis. Type 4 pili are often glycosylated, with carbohydrate decoration affecting function in at least some cases and perhaps serving to obscure antigenic epitopes. However, despite marked differences in the assembly pathways for type 4 pili and P pili, shared structural themes exist. For example, gonococcal type 4 pili are composed predominantly of PilE structural subunits polymerized into a helical rod. A minor phase-variable adhesive protein called PilC is displayed at the tip of gonococcal pili and is essential for pilus-mediated binding to epithelial cells. These observations suggest that N. gonorrhoeae pili may be composite structures with a tip-associated adhesin, analogous to P pili and other pili assembled by the chaperone-usher pathway.
Although adhesive pili are more prevalent in gram-negative bacteria, they are also found in some gram-positive species. One example is Streptococcus parasanguinis , an oral pathogen and a member of the S. sanguinis family. This organism binds to calcium phosphate (the primary mineral component of tooth enamel) and also to other oral bacteria, epithelial cells, platelets, and fibronectin. Several adhesins mediate these binding functions, including pili referred to as long fimbriae . Based on studies of S. parasanguinis strain FW213, long fimbriae are fashioned primarily from Fap1, a 200-kDa protein that includes an unusually long (50 amino acids) signal sequence and a cell-wall sorting signal typical of other gram-positive bacterial surface proteins. Specific glycosylation of Fap1 appears critical to the adhesive function of this fimbrial protein. Interestingly, similar to gram-negative bacterial pili, long fimbriae appear to have a composite structure with a pilus tip. The tip contains an additional adhesin called FimA, which in purified form is capable of blocking bacterial adherence to saliva-coated hydroxyapatite. In work by Burnette-Curley and coworkers, disruption of the fimA gene resulted in a 7- to 20-fold reduction in the incidence of endocarditis after intravenous inoculation of rats. Other gram-positive organisms capable of expressing pili include Streptococcus pneumoniae (a common cause of respiratory tract and invasive disease), Streptococcus agalactiae (group B streptococcus; a common cause of neonatal pneumonia, sepsis, and meningitis), and Enterococcus faecalis (a cause of endocarditis and urinary tract infections).
Nonpilus Adhesins
Beyond pili, a variety of nonpilus adhesins exist. In most cases, nonpilus adhesins are surface-expressed monomeric or oligomeric proteins, although isolated examples of carbohydrate- and lipid-containing adhesive structures have been identified. In general, these molecules are more difficult to visualize by electron microscopy, reflecting their smaller size. Similar to pili, for the most part nonpilus adhesins can be classified according to their mechanism of secretion and presentation on the bacterial surface.
Among the best-characterized bacterial nonpilus adhesins is filamentous hemagglutinin (FHA), a surface protein expressed by Bordetella pertussis and other Bordetella species. The export of FHA to the surface of the organism occurs via the so-called two-partner secretion (TPS) pathway , a conserved strategy in which a secreted protein (TpsA) interacts with a cognate outer membrane transporter (TpsB). In B. pertussis , the TpsA-type protein FHA is transported by a TpsB-type outer membrane protein called FhaC, which has β-barrel pore-forming properties and facilitates translocation of FHA across the outer membrane. Homologous TpsB proteins in other species export the hemolysins of Serratia marcescens, Proteus mirabilis , and Haemophilus ducreyi ; the H. influenzae heme:hemopexin binding protein (HxuA); and the H. influenzae HMW1 and HMW2 adhesins, among others. The crystal structure of FhaC reveals a 16-stranded β-barrel that is occluded by an N-terminal α-helix and an extracellular loop and a periplasmic module composed of two polypeptide-transport-associated (POTRA) domains. Functional studies have demonstrated that the N terminus of FHA interacts with the FhaC POTRA 1 domain, illuminating what appears to be a general feature of interactions between TpsA and TpsB proteins.
Examination of purified FHA by transmission electron microscopy and circular dichroism spectroscopy showed that the FHA molecule is 50 nm in length and adopts the shape of a horseshoe nail. It has a globular head, a 37-nm-long shaft that averages 4 nm in width but tapers slightly from the head end, and a small flexible tail ( Fig. 1.2 ). In the crystal structure of the N terminus of FHA (the so-called TPS domain that interacts with FhaC), a series of 19-residue repeat motifs form a β-helix that is central to the overall structure of full-length FHA. Consistent with its large size, FHA contains at least five separate binding domains, four of which have been localized. The region involved in adherence to sulfated saccharides has been mapped to the N terminus of the FHA molecule. Sulfated saccharides such as heparin and heparan sulfate are a major component of mucus and extracellular matrix in the respiratory tract and are also found on the surface of epithelial cells. The region that recognizes lactosylceramides and promotes adherence to ciliated respiratory epithelial cells and macrophages has been localized to amino acids 1141 to 1279 (the carbohydrate recognition domain). An arginine-glycine-aspartic acid (RGD) tripeptide is located at amino acids 1097 to 1099 and interacts with leukocyte response integrin (LRI), a leukocyte integrin that stimulates upregulation of complement receptor type 3 (CR3). The C terminus of mature FHA has been demonstrated to interact with epithelial cells and macrophage-like cells and appears to modulate the immune response to Bordetella infection. Finally, FHA recognizes CR3 (CD11b/CD18), allowing organisms to be ingested by macrophages without stimulating an oxidative burst. The location of the CR3-binding domain is currently unknown.

A growing number of nonpilus adhesins belong to the so-called autotransporter family. These proteins are synthesized as precursor proteins with three functional domains, including an N-terminal canonical signal sequence, an internal passenger domain, and a C-terminal outer membrane domain. The signal sequence directs the protein to the Sec machinery and is cleaved after it facilitates transport of the polypeptide from the cytoplasm to the periplasm. The C-terminal domain inserts into the outer membrane and forms a β-barrel with a central hydrophilic channel. Ultimately, the passenger domain is presented on the surface of the organism and influences interaction with host molecules. Recent studies have established that autotransporter proteins can be separated into two distinct groups, designated conventional autotransporters and trimeric autotransporters ( Fig. 1.3 ). In conventional autotransporters, the C-terminal outer membrane domain contains roughly 300 amino acids and is a monomeric β-barrel with a single N-terminal α-helix spanning the pore ( Fig. 1.4A ). In trimeric autotransporters, the C-terminal outer membrane domain contains approximately 70 amino acids and forms heat- and detergent-resistant trimers in the outer membrane. Each trimer forms a β-barrel with four strands from each of the three subunits and with three N-terminal α-helices spanning the pore ( Fig. 1.4B ).


One example of a conventional autotransporter adhesin is the H. influenzae Hap protein, which was discovered based on its ability to promote adherence and low-level invasion in assays with cultured human epithelial cells. Hap also promotes bacterial binding to extracellular matrix proteins and bacterial microcolony formation. Examination of chimeric proteins and studies with purified protein have demonstrated that the adhesive activity responsible for Hap-mediated adherence, invasion, binding to extracellular matrix proteins, and microcolony formation localizes to the passenger domain, referred to as Hap S . More detailed characterization of Hap S has established that the region responsible for interaction with host epithelial cells and microcolony formation resides in the C-terminal 311 amino acids and may have utility as a vaccine antigen. This region folds into a triangular prism-like structure that can mediate Hap-Hap dimerization and higher degrees of multimerization, thus facilitating interbacterial interaction and microcolony formation. A prototype member of the trimeric autotransporter subfamily is the H. influenzae Hia adhesin. This protein is expressed in a subset of nontypable H. influenzae strains and contains two homologous high-affinity trimeric binding domains, creating the potential for stable multivalent interaction with respiratory epithelial cells.
Another group of nonpilus adhesins is typified by intimin, a protein expressed by enteropathogenic E. coli (EPEC), enterohemorrhagic E. coli (EHEC), and the murine pathogen Citrobacter rodentium. Intimin contains a flexible N terminus, a central β-barrel domain that integrates into the outer membrane, and a C-terminal binding domain that interacts with the translocated intimin receptor (Tir). Tir is an interesting example of a pathogen-derived receptor that is inserted into target host cells. After initial cell attachment mediated by type 4 pili, EPEC employs a type III secretion system (discussed in detail later in this chapter) to inject Tir into the host cell cytoplasm, from where it is then inserted into the host cell membrane. The subsequent interaction between intimin (on the bacterial surface) and Tir (now present on the host cell surface) triggers receptor clustering, dramatic rearrangement of the actin cytoskeleton, and formation of a distinctive pedestal referred to as an attaching and effacing (A/E) lesion ( Fig. 1.5 ). The bacterial genes essential for formation of A/E lesions reside within a 35-kb region of the EPEC chromosome called the locus of enterocyte effacement (LEE), an example of a pathogenicity island. This locus is highly conserved in content and organization across all A/E pathogens and contains the genes encoding intimin, Tir, and the requisite type III secretion system. The interactions of Tir and other type III secreted effectors with host proteins influencing actin polymerization are beginning to be understood. Tir contains domains analogous to host immunoreceptor tyrosine-based inhibition motifs (ITIM) important for regulation of eukaryotic signaling. On this basis, Tir recruits certain host proteins to regulate actin dynamics and inhibit proinflammatory signaling pathways. Given its central role in EHEC/EPEC pathogenesis and its immunogenicity, intimin is also being examined as a target for the development of antivirulence therapeutics or vaccines in A/E diseases.

In recent years, investigators have identified a large family of nonpilus adhesins involved in adherence to host extracellular matrix proteins including fibronectin, laminin, vitronectin, collagen, fibrinogen, and a variety of proteoglycans. These adhesins have been classified as microbial surface components recognizing adhesive matrix molecules (MSCRAMMs) and are especially prevalent among gram-positive bacteria. In gram-positive organisms these proteins are covalently anchored to the cell wall peptidoglycan and have a characteristic primary amino acid sequence. In particular, the C terminus contains a segment rich in proline and glycine residues, an LPXTG motif (involved in sorting and covalently anchoring the protein to the cell wall), a hydrophobic membrane-spanning domain, and a short positively charged segment that resides in the cytoplasm and serves as a cell wall retention signal. Adhesive functions are typically located near the N terminus.
Staphylococcus aureus is a common gram-positive pathogen in children and is capable of producing a variety of MSCRAMMs, including collagen-binding protein (CNA), fibronectin-binding proteins A and B, and clumping factors A and B. Recent work indicates that although these proteins mediate typical binding interactions with host proteins, they are not monospecific, and a given MSCRAMM may bind multiple host connective tissue components or multiple motifs within a single host fiber type. In addition, many are capable of provoking platelet activation. S. aureus strains recovered from patients with septic arthritis commonly express CNA, which mediates binding to cartilage in vitro and appears to play a key role in the pathogenesis of septic arthritis in experimental mice. Fibronectin-binding protein A (FnBPA) shares homology with S. pyogenes protein F and mediates binding to fibronectin and the γ chain of fibrinogen, as well as to elastin and tropoelastin. Accordingly, this protein is important in S. aureus endocarditis and in infections of implanted biomaterials, which become coated with fibrinogen and fibrin soon after implantation. Clumping factor (ClfA) was named based on the observation that it mediates bacterial clumping in the presence of soluble fibrinogen. Similar to FnBPA, ClfA mediates binding to fibrinogen-coated surfaces in vitro and probably contributes to infections of artificial surfaces.
Other Mechanisms of Adherence
Candida albicans is a common inhabitant of mucosal surfaces and an important cause of systemic disease, especially in patients with compromised immunity. Candida blastospores are capable of efficient adhesion to epithelial cells, leading to budding and division. In addition, germ tube formation occurs, facilitating penetration through the epithelial barrier and then dissemination to distant sites. In recent years, several candidate C. albicans adhesins have been identified. Of particular interest is a protein called INT1, which shares functional homology with the vertebrate integrin family. Integrins are normally expressed by cells of the human immune system (neutrophils, monocytes, macrophages) and mediate cellular binding and shape-changing functions. Each integrin is a heterodimer of an α chain and a β chain. There are a number of distinct α and β chains, and each combination displays a unique binding specificity. INT1 is an α integrin–like protein that recognizes the RGD sequence of the C3 fragment iC3b on epithelial cells. In in vitro assays, short peptides encompassing the RGD sequence are capable of inhibiting C. albicans adherence by 50%, confirming that INT1 plays a significant role as an adhesin and suggesting that other adhesins also exist. Beyond promoting adherence to epithelium, INT1 disguises organisms as leukocytes, allowing evasion of phagocytosis. Of note, introduction of INT1 into Saccharomyces cerevisiae confers a capacity for adherence and also results in germ tube formation, indicating a role for this protein in morphogenesis.
The adhesive properties of C. albicans are closely tied to its morphologic state. For example, adherence to buccal epithelial cells is greater by organisms bearing germ tubes than by yeast forms. With this information in mind, Staab and coworkers searched a germ tube cDNA library and identified a putative adhesin called hyphal wall protein 1 (Hwp1) encoded by the hwp1 gene. Examination of the predicted amino acid sequence of Hwp1 revealed similarity to proteins that are substrates for mammalian transglutaminase enzymes. These enzymes form a cornified envelope on squamous epithelial cells (including buccal epithelial cells) by cross-linking relevant substrates. Interestingly, the interactions of germ tubes with buccal epithelial cells resist stresses (e.g., heating or treatment with sodium dodecylsulfate) capable of dissociating most typical microbe-host adhesive pairs, and elimination of expression of Hwp1 results in a marked reduction in adhesion to buccal epithelial cells. Thus Hwp1 represents a unique adhesive strategy, employing host transglutaminase enzymes to cross-link Hwp1 (via a glycosylphosphatidylinositol remnant anchor) directly to surface proteins on buccal epithelial cells. More recently, Hwp1 has been shown to be important for Candida biofilm formation, indicating that a similar mechanism may also support interactions between candidal cells.
Tissue Tropism
Most microorganisms demonstrate restriction in the range of hosts, tissues, and cell types that they colonize. This restriction is referred to as tropism and generally reflects the specificity of the interaction between a given microbial adhesin and its cognate receptor. Accordingly, tropism is determined by the distribution of the relevant host receptor.
P pili of uropathogenic E. coli serve as the platform for presentation of one of three different PapG variants, referred to as class I, class II, and class III PapG. All three variants recognize globoseries glycolipids, but each binds with a distinct specificity to the globoseries glycolipid isotypes. For example, class I PapG preferentially recognizes globotriosylceramide (GbO3, Gal-α1,4-Gal-β1,3-Glc-ceramide), class II PapG preferentially recognizes globoside (GbO4, GalNAc-β1,3-Gal-α1,4-Gal-β1,3-Glc-ceramide), and class III PapG preferentially interacts with Forssman antigen (GbO5, GalNAc-α1,3-GalNAc-β1,3-Gal-α1,4-Gal-β1,3-Glc-ceramide). Globoside is the dominant globoseries glycolipid expressed in human kidney, and most human isolates of E. coli associated with pyelonephritis express class II PapG. In contrast, Forssman antigen is the most abundant globoseries glycolipid in dog kidney, and more than 50% of canine urinary isolates of E. coli express class III PapG. E. coli– expressing P pili with class II PapG are not found as a cause of urinary tract infection in dogs. Thus the specificity of the PapG variant at the tip of the P pilus influences host range, favoring infection of either human or dog.
The crystal structure of class II PapG bound to Gal-α1,4-Gal was solved by Dodson and coworkers, uncovering the structural basis of PapG binding specificity. Of particular interest, the PapG receptor binding site is located on the side of the molecule and must be oriented with its N- to C-terminal axis parallel to the host cell membrane to allow docking to the receptor. This orientation may be facilitated by the flexibility inherent in the tip fibrillum. The PapG binding site consists of two regions. The first forms a β-barrel, and the second is composed of a central antiparallel β-sheet that is flanked on one side by two 2-stranded β-sheets and on the other side by an α-helix. When class II PapG interacts with GbO4, the arginine residue at position 170 in PapG makes contact with the GbO4 side chain. Interestingly, in class I PapG, a histidine residue occupies position 170, interfering with potential contact with the GbO4 side chain. Similarly, class II PapG and class III PapG differ in amino acids required for interaction with the GbO5 side chain.
Group A streptococcus (S. pyogenes) is a common cause of infections of skin and soft tissue, including impetigo, cellulitis, and necrotizing fasciitis. Adherence to host cells by S. pyogenes is influenced by nonpilus adhesins called M protein and protein F. M protein forms a fiber and consists of a C-terminal region that anchors the protein in the cell wall, a coiled-coil rod region extending approximately 50 nm from the cell wall, and a short nonhelical domain extending more distally. Protein F is a 120-kDa protein that is notable for a tandem repeat element consisting of up to six repeats of 32 to 44 amino acids adjacent to the C terminus. M protein promotes adherence to human keratinocytes via interaction with the CD46 molecule (also called membrane cofactor protein, or MCP), whereas protein F mediates adherence to epidermal Langerhans cells, which are located in the basal layer of the epidermis. Thus both M protein and protein F contribute to group A streptococcal adherence to the skin, but each protein directs interaction with a different population of epidermal cells.
Early studies demonstrated that human immunodeficiency virus type 1 (HIV-1) infects CD4 + cells and interacts with the CD4 molecule but that CD4 alone is not sufficient to permit infection. More recent observations have established that a number of host cell chemokine receptors, especially CCR5 and CXCR4, serve as coreceptors for HIV-1 and are required for viral entry into CD4 + target cells. These coreceptors appear to influence the cellular tropism displayed by different HIV-1 variants. All HIV variants are able to replicate in primary T cells, but only some can also replicate in primary macrophages or in immortalized T-cell lines. Asymptomatic HIV-infected individuals carry strains that generally use CCR5 as a coreceptor (termed M5 strains ) and are non–syncytium-inducing in vitro. Such strains have classically been described as macrophage tropic (M-tropic), but recent experiments have demonstrated that these M5 strains can also infect CD4 + T cells and peripheral blood mononuclear cells. Rapid viral mutation due to the error-prone HIV polymerase and HIV reverse transcriptase leads to the production within the host of syncytium-inducing, T-cell–tropic (T-tropic) HIV-1 strains, which predominate in the circulation of patients with acquired immunodeficiency syndrome. These variants are generally restricted to CXCR4 (expressed on T cells) as a coreceptor, although some primary syncytium-inducing variants can use both CCR5 and CXCR4. T-tropic, syncytium-inducing strains are characterized by positively charged residues at fixed positions of the V3 loop and changes in charge and length of the V2 region of the viral envelope glycoprotein gp120, which binds to CD4 and coreceptors before viral entry into host cells. Thus cellular tropism is closely aligned, but not synonymous, with HIV coreceptor usage.
New HIV-1 infection is selectively established by M-tropic HIV-1 strains, even if the transmitting host harbors more pathogenic non–M-tropic strains as well. CCR5 is also expressed on the surface of rectal and vaginal epithelial cells, which may be sites of initial encounter between HIV-1 and the human host. The importance of CCR5 in HIV-1 binding to CD4 + cells is underscored by the observation that individuals homozygous for a 32-bp deletion in CCR5 (the Δ32 allele) are resistant to infection with HIV-1. The Δ32 heterozygous state does not necessarily protect against HIV-1 acquisition, although HIV disease in heterozygous patients may follow an attenuated course. This allele is surprisingly frequent (10%–14%) in white populations, leading to speculation that it provided a survival advantage during one or more historical epidemics of infectious diseases. However, more recent data suggest that the Δ32 allele may actually confer immune deficiency in the presence of challenge with certain viral pathogens, such as West Nile virus. Of note, CCR5 may have a role in controlling the development of malignancy, including lymphoma, raising some concern about developing anti-HIV pharmacologic agents that target CCR5 function. Finally, co-evolution of viral determinants and host cell receptors may determine the spectrum of tissue and organ involvement within the host. For example, the chemokine receptor CCR8 may facilitate the entry of neurotropic HIV-1 strains into brain cells, and envelopes derived from brain isolates of HIV are adapted to infect cells with low-level CD4/CCR5 expression, such as neuroglia and brain macrophages.
Other viruses also demonstrate tropism for specific cells or tissues within the host. Hepatitis C virus (HCV) has been demonstrated to use multiple cell surface molecules in sequence to locate and gain entry into target cells. HCV is bound to low-density lipoprotein (LDL) in serum and first binds to scavenger-receptor B1 (SR-B1), which is enriched on liver cells and serves to bind lipoprotein molecules. After this initial binding event, HCV E2 protein interacts with the CD81 molecule, the critical receptor for free virus. Once virus has bound to CD81, this virus-receptor complex traffics to the gap junction, where the virus interacts with two key gap junction proteins, claudin-1 and occludin-1, to enter cells. Human occludin-1 recently has been shown to be necessary for HCV entry into mouse cells, an advance that will facilitate disease modeling in the laboratory. Although the liver does not exclusively express any of these four molecules, the combination of these four, the structural organization of liver cells, and other yet-undetermined intracellular factors account for the tropism of HCV for the liver.
Similar observations have been made for coxsackievirus isolates, which bind to the coxsackie-adenovirus receptor (CAR) molecule located in the tight junction for entry into cells. Brain, heart, and muscle cells are enriched for CAR in the fetal and neonatal period, whereas adult cells from these tissues express significantly lower levels of the receptor. This developmental difference in CAR density is the likely explanation for severe coxsackievirus infections that are disproportionately seen in infants and young children compared with adults. CAR then allows for an interaction with the tight junction that is mediated by occludin-1, which allows for viral co-opting of host trafficking pathways.
Among eukaryotic pathogens, tissue tropism can also be a major determinant of virulence. Cerebral malaria is a life-threatening consequence of infection with the protozoan parasite Plasmodium falciparum and results from adherence of parasite-infected erythrocytes to cerebral vascular endothelium. During erythrocyte infection, the malaria parasite exports a variety of surface receptors to the host plasma membrane. The P. falciparum erythrocyte membrane protein 1 (PfEMP1) multigene family represents a highly variable set of such receptors, only one variant of which is expressed at any given time. A substantial body of work has established that different forms of PfEMP1 possess distinct tissue adherence patterns. Specific classes of PfEMP1 types (Group A DC8 and DC13) are associated with cerebral vascular adherence in vitro and are correlated with severe malaria in clinical populations. More recent studies suggest that these PfEMP1 forms mediate adherence through interaction with the endothelial protein C receptor.
Biofilms
After attachment to a particular surface, a number of pathogens are capable of forming biofilms , which can be defined as structured communities of microbial cells enclosed in a self-produced exopolysaccharide matrix. Although most studies of biofilms have involved a single species, it is likely that biofilms relevant to human infection often involve multiple species sharing the advantages of biofilm existence. Human infections associated with biofilms include dental caries, lower airway infection with P. aeruginosa and other organisms in patients with cystic fibrosis, and foreign body infections in patients with prostheses and implanted devices. In addition, biofilm formation likely occurs during osteomyelitis and endocarditis.
P. aeruginosa is a model organism for the study of biofilms and forms pillars of stationary (sessile) bacteria held together by an extracellular polysaccharide called alginate. Interposed among these pillars are channels that facilitate the flow of nutrients and provide pathways for motile (planktonic) organisms to move about ( Fig. 1.6A ). In experiments directed at defining the early steps of P. aeruginosa biofilm formation, O’Toole and Kolter established that flagella are required for initial bacterial attachment, presumably because these appendages promote movement toward the relevant surface. After attachment, type 4 pili and pilus-mediated twitching motility promote formation of microcolonies in which transcription of algC, algD, and algU is activated, resulting in synthesis of alginate. Pulmonary isolates from patients with cystic fibrosis often form highly mucoid colonies (reflecting expression of alginate) or can form tiny colonies on agar plates, the so-called small-colony variant (SCV) phenotype associated with biofilm formation and increased antibiotic resistance.

Development of the complex community present within a biofilm requires intercellular communication to coordinate the metabolic and other activities of members of the community. P. aeruginosa employs several identified quorum-sensing systems, which involve the production of small molecules that are sensed by neighboring organisms and regulate gene expression in these neighbors. One well-studied system is based on the acyl-homoserine lactone called N -(3-oxododecanoyl)- l -homoserine lactone (3OC12-HSL). 3OC12-HSL is synthesized in a reaction catalyzed by LasI and accumulates with increases in population density. Ultimately, 3OC12-HSL reaches a critical concentration and then interacts with LasR, serving to activate transcription of a number of genes. Host inflammatory pathways are also induced directly by accumulated 3OC12-HSL. Organisms with a mutation in lasI are capable of attachment and microcolony formation, but the resulting microcolonies remain thin, undifferentiated, and sensitive to dispersion by detergents. Addition of the missing lactone signal to the lasI mutant restores development into structured, thick, biocide-resistant biofilms, as are observed with wild-type organisms. In vivo, mutation in lasI impedes establishment of pulmonary infection in mice.
Biofilms also play a prominent role in human infections with Candida species, with examples including oral thrush and catheter-associated infections. Although several pathogenic Candida species can form biofilms in the host, the ultrastructure and the molecular strategies underlying biofilm formation vary from one species to another. C. albicans is the best studied Candida species and relies on the expression of certain cell wall proteins (including Hwp1), a regulated yeast-to-hyphal switch described earlier, and quorum-sensing molecules such as E,E -farnesol, which represses filamentation and can suppress the growth of other nearby bacterial and fungal species.
Biofilms constitute a protected mode of growth that allows survival in a hostile environment—for example, in the presence of host immune mechanisms or antimicrobial agents. Based on studies of P. aeruginosa , sessile bacteria release antigens and stimulate production of antibodies, but these antibodies are ineffective in killing organisms within biofilms. Similarly, sessile P. aeruginosa stimulate a diminished oxidative burst and are relatively refractory to phagocytic uptake. In addition, fungi and bacteria within biofilms are resistant to the effects of a number of antimicrobial agents, in part because these agents are unable to diffuse into the biofilm and in part because these organisms may exist in a slow-growing or otherwise protected phenotypic state. Biofilm-like microbial communities have also been described within host epithelial cells, as with uropathogenic E. coli in the mammalian bladder. Recently, new approaches to antimicrobial therapy include novel natural products and other small molecules that inhibit quorum-sensing and biofilm formation.
Cell Entry and Intracellular Life
After adherence to a host surface, many pathogenic bacteria are able to invade and survive inside epithelial cells and other nonprofessional phagocytes (i.e., M cells in intestinal Peyer’s patches). In addition, some pathogens are able to survive inside professional phagocytes (macrophages and neutrophils). Invasion may represent a mechanism to breach host mucosal barriers and gain access to deeper or more distant tissues. Alternatively, invasion may provide the organism with a special niche (e.g., protecting it from host immune mechanisms). In the case of viruses, cell entry ensures access to the cell machinery required for viral replication.
Generally the process of bacterial invasion involves a class of molecules called invasins that mediate adherence and entry. For many bacteria, invasion is an active event that relies on underlying host cell functions and is associated with rearrangement of the host cell cytoskeleton. Once inside the host cell, the invading or internalized organism usually is localized within a membrane-bound vacuole that contains lysosomal enzymes. In some cases the pathogen escapes from this vacuole and enters the cytoplasm, a more permissive environment. In other cases, the pathogen remains in the vacuole and neutralizes lysosomal enzymatic activity. The processes of invasion into cells, survival within cells, cell-to-cell spread, and entry into the circulation define the extent of infection and dissemination.
Invasion
In considering the molecular mechanism of bacterial invasion, perhaps best characterized are the enteropathogenic Yersinia species—namely, Y. pseudotuberculosis and Y. enterocolitica . These organisms are usually acquired by ingestion of contaminated food or water and typically cause self-limited enteritis or mesenteric adenitis. In infants and other individuals with compromised immunity, they sometimes produce systemic disease. The primary determinant of Y. pseudotuberculosis and Y. enterocolitica invasion is an adhesive outer membrane protein called invasin, which is encoded by a chromosomal locus called inv and binds tightly to a family of β1 integrins expressed on host cells, including α3β1 integrin on the surface of intestinal M cells. The interaction between invasin and β1 integrins initiates a cascade of signaling steps in the host cell, resulting in actin rearrangement and formation of large complexes of cytoskeletal elements (talin, vinculin, α-actinin, and others) termed focal adhesions. Bacterial entry into the host cell occurs via a “zipper-like” mechanism, with the plasma membrane zippering around the invading organism.
Beyond invasin, two additional proteins called YadA and Ail also influence invasion by enteropathogenic Yersinia species. YadA is a 45-kDa surface protein that is encoded by the 70-kb Yersinia virulence plasmid. It is highly expressed under environmental conditions (e.g., temperature of 37°C) in which invasin is repressed. YadA reaches the bacterial surface via the autotransporter pathway and exists in a trimeric form that is essential for its adhesive activity. Like invasin, YadA promotes invasion through binding to β1 integrins on the host cell surface, but its binding occurs indirectly via extracellular matrix molecules, including collagens, laminin, and fibronectin. Based on studies using a mouse oral infection model, in Y. enterocolitica YadA is essential for survival and multiplication in Peyer’s patches, whereas in Y. pseudotuberculosis YadA is dispensable for full virulence. Ail is a 17-kDa outer membrane protein that also is encoded by a chromosomal locus (ail) and mediates high levels of adherence and low levels of invasion in assays with cultured epithelial cells. In addition, Ail mediates resistance to complement-mediated serum killing, independent of an effect on invasion.
Similar to these pathogenic Yersinia species , Listeria monocytogenes invades epithelial cells via a zipper-like mechanism. Invasion is mediated by proteins called internalin A (InlA) and internalin B (InlB), which are required for virulence in animal models. InlA interacts with E-cadherin, a host cell transmembrane protein with an intracellular domain that interacts with the cytoskeleton. InlB interacts with C1q on host cells and promotes invasion by activating the PI-3 kinase pathway. Uropathogenic strains of E. coli also invade epithelial cells via a zipper-like mechanism mediated by the FimH adhesin expressed on the tip of type 1 pili. In experiments with cultured bladder epithelial cells, FimH is both necessary and sufficient for entry, as demonstrated by examination of a fimH − mutant and of latex beads coated with purified FimH. In vitro experiments further suggest that FimH-mediated bacterial binding to a mannose-coated surface may be strengthened by shear forces, such as fluid flow over the surface. After FimH-dependent invasion into superficial epithelial cells of the murine bladder, UPEC multiply rapidly to form intracellular bacterial communities, which display some features of biofilms, including community behavior, differential gene expression, and protection from antimicrobial agents (see Fig. 1.6B ). A subset of internalized bacteria ultimately form a quiescent bacterial reservoir within the uroepithelium that resists immune clearance and antibiotic therapy and may serve as a seed for recurrent infections.
Salmonella enterica serovar typhimurium (S. typhimurium) is an example of a pathogen that invades cells by a mechanism distinct from zippering. On contact with the epithelial cell surface, S. typhimurium triggers a dramatic host cell response characterized by actin rearrangement, calcium and inositol phosphate fluxes, and a “splash” of membrane ruffling surrounding the point of entry. Bacterial internalization into the cell occurs rapidly, with organisms appearing in membrane-bound vacuoles within a few minutes of initial contact with the host cell. The determinants of S. typhimurium invasion are encoded by a pathogenicity island called SPI-1, located at centisome 63 on the bacterial chromosome. Especially important in this region is a prototypical type III secretion system, which forms a needle-like complex on the bacterial surface that breaches the host cell membrane and serves to translocate bacterial proteins directly into the host cell, altering the host cell cytoskeleton and influencing immune responses. The base of the needle complex spans both the inner and outer membranes and is about 40 nm in diameter, whereas the needle itself is 8 nm in width and approximately 80 nm in length ( Fig. 1.7 ).

The proteins secreted through the S. typhimurium needle complex (and other type III secretion systems) and into the host cell are referred to as effector proteins. SopE is an effector protein that mediates the initial rearrangement of actin and ruffling of the host cell membrane. It functions as a guanyl-nucleotide exchange factor (GEF) and activates two host cell Rho GTPase proteins called Rac and Cdc42. SptP is an effector protein that functions as an antagonist of SopE, mediating reversal of actin rearrangement by converting Rac and Cdc42 to the inactive forms (GDP forms). Consistent with these functions, SopE and SptP directly antagonize each other when coinjected into cells. Other effector proteins secreted by the S. typhimurium SPI-1 type III secretion system include the inositol phosphate phosphorylase SopB, which disrupts normal host cell signaling mechanisms, and AvrA, which interferes with the nuclear factor κB (NF-κB) signaling pathway in host cells, thereby downregulating host inflammatory responses.
Important accessory and regulatory genes are also present within SPI-1. As an example, the sicA gene is just upstream of the sipB and sipC genes and encodes an accessory protein with chaperone activity essential for stabilization and translocation of SipB, SipC, and SopE. Other chaperones encoded by SPI-1 are involved in the stabilization and translocation of other effector proteins. The genetic and environmental factors that regulate the expression of type III secretion machinery and secreted proteins represent an area of ongoing study.
In Plasmodium falciparum , the mechanisms of both host erythrocyte invasion and immune evasion are tightly linked. A mature parasite may release as many as 32 daughter parasites, called merozoites, into the bloodstream. In less than 30 seconds, merozoites attach to and invade new erythrocytes. High-titer antibodies to invasion proteins, as are present in the serum of semi-immune individuals living in endemic areas, can block these processes. For this reason, the molecular invasion machinery of P. falciparum is of considerable interest for vaccine development. The initial contact between Plasmodium merozoites and host erythrocytes is weak and is thought to be mediated through merozoite surface proteins (MSPs) present along the entire merozoite surface. Stronger interactions are mediated by several additional receptors, notably the erythrocyte binding antigens EBA-175 and EBA-140, which engage the erythrocyte-specific receptors glycophorin A and glycophorin B, respectively. Recently, an additional parasite protein called PfRH5 was recognized as an indispensible mediator of parasite invasion via interaction with the human receptor basigin. Anti-RH5 antisera have potent invasion-inhibiting activities, and an RH5-based vaccine showed efficacy in an Aotus monkey infection model. On the basis of these data, PfRH5 has emerged as a strong candidate vaccine antigen to prevent severe malaria.
Intracellular Survival
Once an organism invades a nonprofessional phagocyte or is ingested by a professional phagocyte, several potential outcomes exist. Often, the organism is killed. However, some pathogens have developed strategies to survive and replicate inside host cells, in some cases within a vacuole and in others by escaping from the vacuole.
There is general agreement that S. typhimurium resides within a membrane-bound vacuole in both professional and nonprofessional phagocytes. However, the vacuole lacks several lysosomal markers typical of the main endocytic pathway (the mannose-6-phosphate receptor pathway) and appears to be distinct from this pathway. Insight into the molecular determinants of intravacuolar survival came when two independent groups reported the discovery of a second Salmonella pathogenicity island, now called SPI-2. This island maps to centisome 31 and encodes another type III secretion system, including structural proteins ( ssa locus), effector proteins ( sse locus), and accessory proteins ( ssc locus). In addition, this region encodes a two-component regulatory system consisting of a membrane-located sensor kinase (SsrA) and a transcriptional regulator (SsrB). Mutations in SPI-2 result in reduced survival inside macrophages, with no effect on adherence and invasion in assays with intestinal epithelial cells. Salmonella SPI-2 mutants demonstrate reduced virulence in experimental mice (up to a 104-fold reduction in 50% lethal dose), suggesting that survival inside macrophages is a key factor in the pathogenesis of disease. Expression of SPI-2 genes within the macrophage vacuole depends at least in part on the acidic intravacuolar environment. Inhibition of macrophage vacuolar acidification using bafilomycin A1 (an inhibitor of the vacuolar proton ATPase) results in a sharp attenuation in transcription of SPI-2 genes. This effect is not reproduced by low pH alone outside the vacuole, suggesting that other environmental effects within the vacuole influence SPI-2 expression. Recent work indicates that Salmonella SPI-2 transcription is activated before invasion, apparently preparing the pathogen for the hostile intracellular environment. As a group, the SPI-2 genes appear to modulate host endocytic and exocytic transport mechanisms and inflammatory signaling.
A third Salmonella pathogenicity island called SPI-3 also promotes survival inside macrophages. This island is located at centisome 82 and was discovered by examining the Salmonella selC locus, a tRNA gene where pathogenicity islands reside in some strains of E. coli. SPI-3 contains the mgtBC operon, which permits S. typhimurium growth in environments with low concentrations of Mg 2+ , including macrophages. In particular, mutation of the mgtBC operon abolishes the ability of S. typhimurium to replicate in low-Mg 2+ liquid media and in macrophages, and addition of Mg 2+ to the medium after phagocytosis restores the ability to survive intracellularly. Homologous mgtBC genes have been found in other organisms with intracellular lifestyles, such as Brucella melitensis and Yersinia pestis. In Salmonella, the mgtBC genes are expressed after internalization into host cells under control of the PhoP-PhoQ two-component regulatory system, a complex that directs expression of a number of virulence determinants.
The ability to survive within phagocytic cells may provide Salmonella with a means to exploit an intrinsic host pathway and disseminate to distant sites. In particular, certain phagocytes express the β2 integrin CD18, which mediates leukocyte migration in response to various stimuli. During S. typhimurium infection, CD18-expressing phagocytes carry organisms from the intestine to the spleen. Indeed, bacterial loads in the liver and spleen are reduced after oral inoculation in CD18-deficient mice when compared with infection in wild-type mice. On the one hand, this function of CD18 facilitates initiation of a systemic immune response and benefits the host. However, at the same time, it provides bacteria with a mechanism of transit from the gut to organs of the reticuloendothelial system and elsewhere.
Mycobacterium tuberculosis is another intracellular pathogen, and it uses an array of mechanisms to ensure intracellular survival. The M. tuberculosis vacuole lacks the usual amounts of the vesicular proton ATPase responsible for mediating acidification and fails to acidify to normal levels. In addition, M. tuberculosis blocks fusion of the vacuole with acidic lysosomes, further preventing acidification. Similar to intracellular gram-negative bacterial pathogens, M. tuberculosis contains an mgtC gene, and mutation of this gene results in impaired virulence in cultured human macrophages and in mouse spleen and lung. Low Mg 2+ concentration and mildly acidic pH inhibit the growth of the mgtC mutant, suggesting that the gene is important for survival in the phagosome, where such conditions may exist. Another factor that influences M. tuberculosis survival within macrophages is isocitrate lyase, an enzyme of the glycolytic shunt that is essential for metabolism of fatty acids. Expression of isocitrate lyase is upregulated during infection of activated macrophages and is required for full virulence in a murine model of infection, independent of an effect on bacterial growth. The crystal structure of M. tuberculosis isocitrate lyase has been solved and may provide a target for new drug therapies against persistent infection because this enzyme is absent from vertebrates.
During the course of interaction with macrophages, M. tuberculosis (at a low to moderate multiplicity of infection) is capable of stimulating caspase-1 and inducing macrophage apoptosis. Interestingly, less virulent strains of M. tuberculosis are more potent inducers of apoptosis, perhaps resulting in benefit to the host by preventing systemic spread of infection. At the same time M. tuberculosis possesses at least two anti-apoptotic mechanisms that further influence the outcome of macrophage encounters. First, M. tuberculosis infection enhances host macrophage production of soluble TNFR2, a protein that binds to tumor necrosis factor alpha (TNFα) and interferes with apoptosis. Second, M. tuberculosis infection activates production of NF-κB, a transcriptional regulator that activates anti-apoptotic pathways within the host cell. Of note, higher multiplicities of infection with virulent strains of M. tuberculosis can induce caspase-independent cell death in macrophages, a mechanism proposed to contribute to the formation of necrotic lesions during tuberculous disease.
L. monocytogenes is an example of an organism that escapes from the phagocytic vacuole in macrophages and epithelial cells and moves into the cytoplasm. This organism causes meningitis and focal brain abscesses in humans and exhibits tropism for the fetoplacental unit. In pregnant women, listeriosis results in fetal loss in 30% of cases. Intravacuolar replication and escape from the vacuole are dependent on listeriolysin O, a hemolysin encoded by the hly gene. Listeriolysin O interacts with cholesterol in host cell membranes and forms pores, leading to lysis of the phagosome. Host enzymatic activities may also contribute to Listeria escape from the phagosome. In human epithelial cells the contributions of a broad-range phospholipase C (called PC-PLC) and a metalloproteinase called Mpl are most important for vacuolar escape in the absence of listeriolysin O.
Intracellular survival of the protozoan parasite Toxoplasma gondii is thought to rely on parasite virulence factors that directly counter innate host defenses. In mice, interferon gamma (IFNγ) production is required to limit replication of T. gondii , in part through induction of immunity-related GTPases (IRGs). In mammalian cells, successful T. gondii strains replicate within a protected parasitophorous vacuole (PV) that does not fuse to the host lysosome. During infection with relatively nonpathogenic parasites, recruitment of IRGs to the PV results in disruption of the PV and parasite death. In contrast, highly pathogenic T. gondii express an active serine-threonine kinase called ROP18, which is exported into the host cytoplasm and prevents the recruitment of IRGs. The ROP kinase family is highly expanded in T. gondii , comprising 44 other proteins, many of which lack critical catalytic residues and are thought to function as regulatory “pseudokinases.” Interestingly, ROP kinases are absent from the related apicomplexan Plasmodium spp., presumably because prevention of IRG recruitment is unnecessary within the protected niche of the host erythrocyte.
Viral Cell Entry
Viruses have developed a specialized family of proteins that specifically function to engage host cell proteins and fuse with cell membranes and that allow for transfer of viral genetic material. The details regarding structure and function of these proteins are reviewed elsewhere. There are two known classes of viral fusion proteins: class I proteins form a hairpin structure with a known α-helix domain (e.g., HIV gp41, influenza HA2), and class II proteins exist in β-sheets and transition from a moderately stable dimeric form to a very stable trimer (e.g., dengue E protein). These fusion proteins undergo conformational changes when transitioning from the prefusion to the postfusion state, resulting in a more stable form that favors the process of viral fusion and entry.
Cell-to-Cell Spread
Movement from one cell to another may help an organism gain a stronger foothold in host tissues. L. monocytogenes is one example of a pathogen capable of cell-to-cell spread. Once this organism is free in the cytoplasm, actin begins to polymerize on the bacterial surface. Eventually the condensed actin forms a polar tail or comet, which propels the organism through the cytoplasm and into adjacent cells. The rate of bacterial movement within a cell correlates with actin tail length. Actin accumulation and condensation is mediated by the L. monocytogenes ActA protein, which is tightly anchored to the bacterial surface and is expressed asymmetrically over the length of the organism. ActA is the sole Listeria factor required for actin polymerization because actin tails form in Xenopus cytoplasmic extracts containing ActA-coated beads. However, in these experiments, motility occurs only when ActA is distributed asymmetrically on the beads. ActA appears to interact directly with actin and also with a variety of other host cytoskeletal proteins. Cytochalasin D is an inhibitor of actin polymerization and inhibits the cell-to-cell spread of L. monocytogenes in epithelial monolayers.
On reaching the plasma membrane, bacteria protrude from the cell in filopodium-like structures (called listeriopods), which are then engulfed by neighboring cells. This engulfment may be part of a normal host process because MDCK cells demonstrate low-level endocytosis of adjacent cell membrane fragments even in the absence of bacteria. The formation of listeriopods and the engulfment of these structures by neighboring cells are independent of listeriolysin O, PI-PLC, and PC-PLC. Once inside a nascently infected cell, Listeria escapes from the double-membrane vacuole via the action of PI-PLC, PC-PLC, and Mpl. On arrival in the cytosol, bacteria can enter another cycle of actin-based motility and cell-to-cell spread, although one or two bacterial generations may be necessary to regain motility.
A second pathogen capable of actin-based motility and cell-to-cell spread is Shigella flexneri. In Shigella, a single protein called IcsA is sufficient to induce formation of an actin tail, similar to that observed in L. monocytogenes. IcsA is an autotransporter protein that is encoded on the Shigella virulence plasmid and is distributed on the bacterial surface in a polarized fashion, possibly as a result of specialized machinery for autotransporter protein secretion near the poles of some gram-negative bacteria. Initially, IcsA is distributed over the whole bacterial surface, with a predominance at one pole. However, over time a secreted bacterial protease called IcsP cleaves roughly half of the surface IcsA, mostly at the opposite pole, further polarizing distribution. Elimination of expression of IcsP leads to increased quantities of IcsA and increased actin-based motility, suggesting that IcsA (rather than host factors) is rate limiting in the motility process. Like ActA, IcsA is necessary and sufficient to induce polymerization of the actin tail, and the tail forms at the end where IcsA concentration is highest. Despite the functional similarities between IcsA and ActA, there is no significant sequence homology between the two proteins. In contrast to ActA, no direct interaction between IcsA and actin has been demonstrated, and IcsA is found throughout the actin tail, not only at the bacterial pole–actin tail junction.
When considering cell-to-cell spread of viruses, it is helpful to return to the example of HCV. Claudin-1 and occludin-1 are the gap junction proteins that serve as the final entry point for this virus. It has been demonstrated that HCV can infect neighboring liver cells directly via these gap junction proteins, likely bypassing the extracellular release of virus and subsequent SR-B1 and CD81 binding steps. Experiments have demonstrated that antibodies blocking the E2–CD81 interaction inhibit infection from virus-infected media but do not affect infection of naïve cells when co-cultured with previously HCV-infected cells. It has also been shown that after infecting a liver cell, HCV promotes breakdown of typical apical to basal organization of liver cells, exposing the gap junction complexes and presumably facilitating cell-to-cell spread.
Damage to the Host
Damage to host cells and host tissues represents a fundamental mechanism by which a pathogen is able to survive at a given site and then spread within a host. Generally, damage is induced by microbial toxins. Most toxins are released extracellularly and are capable of inducing damage at very low concentrations (exotoxins). Microbial attachment and invasion facilitate toxin delivery to target cells and target tissues and serve to enhance toxicity.
Historically, microbial toxins have been classified according to a variety of criteria, including cellular target of action (e.g., enterotoxins, leukotoxins, neurotoxins), mechanism of action (e.g., adenosine diphosphate [ADP]–ribosylating toxins, adenylate cyclase toxins, pore-forming toxins, proteolytic toxins), and major biologic effect (e.g., hemolytic toxins, edema-producing toxins). In recent years the term toxin has been applied more broadly to include enzymes that mediate damaging effects via phospholipase or hyaluronidase activity.
Bordetella pertussis Toxins
Whooping cough ( B. pertussis infection) is a classic example of a toxin-mediated disease and involves the interplay of multiple toxins. The pathogenesis of whooping cough begins with B. pertussis colonization of the trachea, which is facilitated by a molecule called tracheal cytotoxin (TCT). TCT is a naturally occurring disaccharide-tetrapeptide fragment of peptidoglycan and belongs to the family of muramyl peptides. Many gram-negative organisms produce an analogous fragment during normal turnover of cell wall components, but significant extracellular release appears to occur only in Bordetella species and gonococci. In most other species an inner membrane protein called AmpG recycles this fragment back into the bacterial cell. TCT is toxic to tracheal epithelial cells in vitro, stimulating nitric oxide synthase and local production of interleukin-1 and causing inhibition of ciliary motility, inhibition of DNA synthesis, and cell death. During natural infection, TCT is thought to paralyze the mucociliary escalator and thereby interfere with clearance of B. pertussis and respiratory mucus.
Pertussis toxin is believed to be a key determinant of the clinical manifestations of whooping cough. This toxin belongs to a family of bacterial ADP-ribosyltransferase enzymes. The target of pertussis toxin is host cell G proteins, resulting in disruption of normal signaling processes. A number of biologic effects have been ascribed to pertussis toxin, including induction of lymphocytosis, stimulation of insulin release, sensitization to histamine, and disruption of phagocytic cell function; however, the specific relationship between the effects of pertussis toxin and the symptoms of whooping cough remains unclear. Of note, B. parapertussis is closely related to B. pertussis and produces a similar cough illness but fails to produce pertussis toxin because of mutations in the ptx promoter region.
B. pertussis also elaborates a toxin called adenylate cyclase toxin (CyaA), a member of the RTX (repeat-in-toxin) family of bacterial cytolysins whose prototype is the E. coli hemolysin HlyA. These toxins cause target cell lysis by creating pores in the host cell plasma membrane, but at sublytic concentrations many of these toxins also manipulate host enzymatic and signaling pathways within the host cell. In the case of B. pertussis, CyaA inhibits host adenylate cyclase, resulting in accumulation of cyclic adenosine monophosphate (cAMP); elevated levels of cAMP within phagocytic cells inhibit oxidative activity and induce apoptosis, thus disabling this arm of the immune system. In respiratory epithelial cells, elevated cAMP may result in increased fluid and mucus secretion, further impairing mucociliary function.
Among other examples of these dual-function RTX toxins, the prototypic HlyA of uropathogenic E. coli induces the degradation of host actin-associated proteins, resulting in exfoliation of the superficial epithelial layer in the bladder. The α-hemolysin of contemporary community-associated S. aureus strains activates a host epithelial cell surface molecule called ADAM10, which cleaves E-cadherin at cell–cell junctions to permit access of the pathogen across the epithelial layer.
Hemolytic-Uremic Syndrome and Shiga Toxins
A number of intestinal pathogens produce Shiga toxins, including Shigella dysenteriae, enterohemorrhagic E. coli (including E. coli O157:H7), and Citrobacter freundii, among others. Shiga toxins are classic A-B toxins, consisting of an A subunit that has toxic activity and five B subunits arranged in a pentameric ring-like structure that promotes binding to host cells and delivery of the A subunit. The B subunits interact with host cell globoseries glycolipids, especially the Pk trisaccharide moiety of globotriaosylceramide (GbO3). The A subunit is endocytosed by the host cell and traverses the cytoplasm in membrane-bound vesicles. Some of these vesicles travel in a retrograde fashion to the Golgi apparatus and then to the endoplasmic reticulum. Shiga toxin then co-opts the function of the endoplasmic reticulum proteins HEDJ and BiP to enter the cytosol, where it enzymatically inhibits host 28S ribosomal RNA by cleaving a single adenine residue, resulting in inhibition of protein synthesis and cell death.
In humans, E. coli O157:H7 is an important cause of hemorrhagic colitis and sometimes produces hemolytic-uremic syndrome. Infection begins with adherence to epithelial cells via intimin and other proteins encoded by the locus of enterocyte effacement (LEE), resulting in formation of attaching and effacing lesions analogous to those observed in EPEC infection. After adherence, the organism releases Shiga toxin, which traverses the intestinal epithelial cell and enters the bloodstream. Toxin circulates to distant organs and mediates damage via toxicity to endothelium. Diarrhea likely results from damage to endothelium in small mesenteric vessels, leading to ischemia and sloughing of the intestinal mucosa. The renal effects observed in human hemolytic-uremic syndrome arise from microvascular and glomerular damage with luminal occlusion by fibrin and platelets. Hemolysis and thrombocytopenia likely develop as a consequence of microangiopathy.
Tissue-Degrading Toxins
A number of toxins have enzymatic activity and are capable of degrading tissue components. One example is hyaluronidase, which degrades hyaluronic acid, a repeating disaccharide glycosaminoglycan involved in cell motility, adhesion, and proliferation in normal hosts. Hyaluronic acid contains alternating N -acetylglucosamine and glucuronic acid moieties, connected by β linkages. It is prominent in extracellular matrix when cell turnover and tissue repair are prominent—for example, in embryogenesis, wound healing, and carcinogenesis. The primary host receptor for hyaluronic acid is CD44, which undergoes post-translational modification that varies according to host cell type. Interactions between hyaluronic acid and CD44 are critical to T- and B-cell stimulation, growth of certain lymphoid malignancies, and propagation of certain inflammatory responses.
In S. pyogenes, hyaluronidase is a 96-kDa protein that is encoded by the hylA gene and is released extracellularly. It is proposed to promote invasion through cell layers and tissue planes and is considered one of several S. pyogenes spreading factors. Interestingly, S. pyogenes also produces a thick “capsule” of hyaluronic acid that can interact with other host cellular and extracellular matrix proteins to contribute to tissue invasion by the organism. Other pathogens that produce a hyaluronidase include S. agalactiae (group B streptococcus), Treponema pallidum, Candida spp., Entamoeba histolytica, and Ancylostoma braziliense.
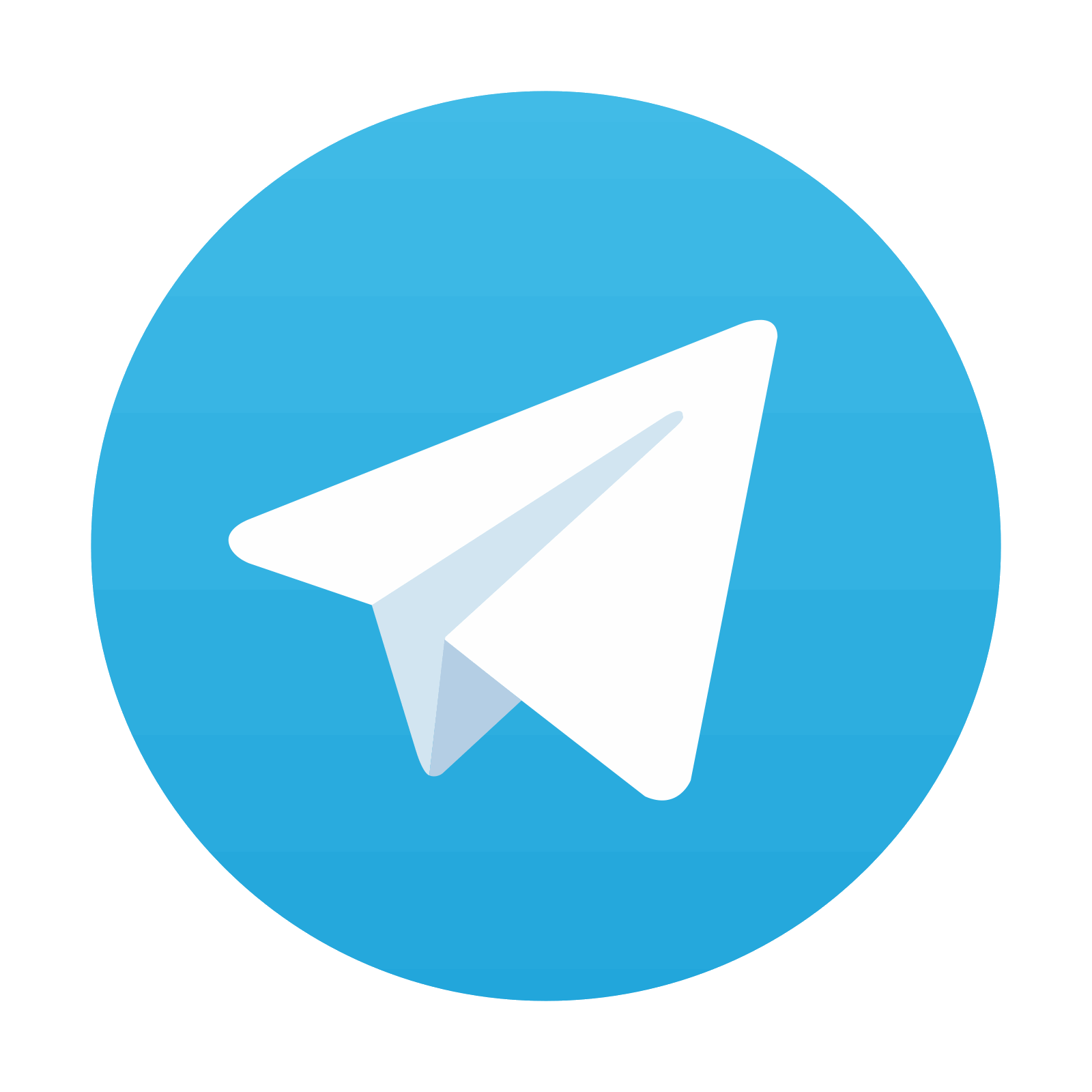
Stay updated, free articles. Join our Telegram channel
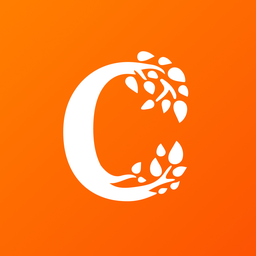
Full access? Get Clinical Tree
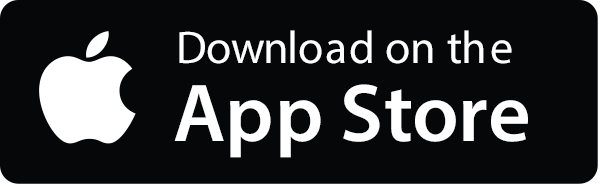
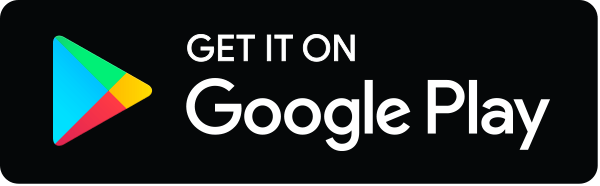