Chapter Contents
Part 1: Disorders of metabolic homeostasis in the neonate 850
Introduction 850
Glucose homeostasis in the healthy fetus and neonate 851
Fetal metabolism 851
Metabolic changes at birth 851
Neonatal metabolism 851
Differences between neonatal and adult metabolism 852
Hypoglycaemia 853
Clinical significance 853
Definition 853
Diagnosis 854
Prevalence of neonatal hypoglycaemia 855
Mechanisms and at-risk groups 856
Prevention and management of neonatal hypoglycaemia 858
Hyperglycaemia 861
Summary 864
Part 2: Endocrine disorders 868
Hypothalamus and anterior pituitary 868
Normal function 868
Hypothalamic and anterior pituitary disease 868
Resistance to the actions of anterior pituitary hormones 871
Posterior pituitary 871
Normal function 871
Cranial diabetes insipidus 871
Excess vasopressin secretion (syndrome of inappropriate antidiuretic hormone secretion) 872
The hormonal regulation of appetite and feeding behaviour 872
Thyroid 872
Normal function 872
Congenital hypothyroidism 873
Neonatal screening for congenital hypothyroidism 873
Neonatal screening and the preterm infant: the delayed TSH rise 876
Other abnormalities of thyroid function in the neonatal period 876
Peripheral abnormalities of thyroid hormone binding, transport or action 877
Hyperthyroidism 877
Disorders of calcium metabolism 880
Adrenal 885
Normal development and function 885
Inherited disorders of steroidogenesis: the congenital adrenal hyperplasias 886
Other causes of adrenocortical insufficiency 890
Glucocorticoids and adrenal function in the neonate 891
Biochemical assessment of adrenal functionin the neonate 892
Adrenal hyperfunction 892
Disorders of sex development 892
Normal sexual differentiation 892
Disorders of sex development 893
Disorders of urogenital and gonadal development 894
Genes involved in the formation of the bipotential urogenital (gonadal) ridge 894
Disorders of androgen production or action: incomplete virilisation in a baby with an XY karyotype 895
Patients with an XX karyotype who have been exposed to excess androgen 896
The clinical approach to problems of intersex 897
Part 3: Inborn errors of metabolism in the neonate 906
Introduction 906
The organisation of clinical and laboratory services 907
Pathogenesis of inborn errors of metabolism 908
Clinical presentation of inborn errors of metabolism 908
Investigation of a baby who may have an inborn error of metabolism 909
General approach 909
Specific tests: ‘the metabolic screen’ 909
Management while awaiting results 911
Management of babies with severe acute inborn errors of metabolism 911
Long-term management after the acute phase has passed 912
Management when no diagnosis can be made 912
Further management when death is inevitable 912
Special management when an inborn error of metabolism can be anticipated 912
Detection of inborn errors of metabolism by mass screening 912
Consideration of specific inborn errors of metabolism 914
Amino acid disorders 914
Urea cycle disorders 915
Organic acid disorders 916
Defects in carbohydrate metabolism 916
Fatty acid oxidation defects 917
Disorders of subcellular organelles 917
Miscellaneous disorders 918
Part 4: Metabolic bone disease 920
Background 920
Diagnosing metabolic bone disease in preterm infants 920
Aetiology 920
Disturbed mineral metabolism in the neonatal period 920
Reduced or static bone mineral accretion 921
Radiological changes 922
Fractures 922
Long-term outcome 922
Effects of drugs and immobilisation 922
Treatment: prevention is better than cure 923
Monitoring and adjusting treatment in affected infants 923
Inherited metabolic bone disease 923
Disorders of metabolic homeostasis in the neonate
- Jane Hawdon
Introduction
Neonatal hypoglycaemia has been recognised for a century ( ; ; ), although over these years there have been wide swings of opinion regarding the definition of the condition, its clinical significance and its optimal management. For example, in the era when routine postnatal management involved the withholding of feeds from healthy infants for up to 24 hours, and even longer in sick or small babies, many were found to have low blood glucose concentrations, and this became accepted as a normal finding ( ). Healthy term babies have a number of protective mechanisms to prevent the postnatal physiological fall in blood glucose causing harm and these mechanisms are discussed below. However, some babies have impaired protective responses and display clinical signs of hypoglycaemia. The risk of reduced glucose availability to the brain in such circumstances is acknowledged ( ; ). wrote: ‘Certainly the risk of such complications forms a cogent incentive to all concerned to make the diagnosis as early as possible in every case’.
More recently, practice swung towards the treatment of large numbers of infants, often unnecessarily, with intravenous glucose, resulting in separation from their mothers and jeopardising breastfeeding. It is important to identify those infants most at risk of adverse effects of hypoglycaemia and determine the most effective and least invasive regimens for prevention of hypoglycaemic brain injury ( ). To date, no controlled study has addressed either of these issues.
Hyperglycaemia was also recognised as a neonatal complication over a century ago ( ) and until recent times was a rare phenomenon. However, it is now commonly seen in the increasing numbers of extremely low-birthweight infants who are cared for in our neonatal units. Despite this history and current frequency, uncertainty as to its clinical significance and optimal management remains.
To manage these disorders of blood glucose homeostasis it is essential to understand the metabolism of the fetus and neonate and the changes that occur at birth in the healthy infant. This chapter will summarise the current knowledge of the disorders of blood glucose homeostasis, and aims to provide a practical and pragmatic approach to the management of babies with hypoglycaemia and hyperglycaemia.
Glucose homeostasis in the healthy fetus and neonate
Fetal metabolism
During pregnancy the human fetus receives from its mother, via the placental circulation, a supply of substrates necessary for growth, for the deposition of fuel stores which are essential after birth (see below) and for energy to meet the basal metabolic rate and requirements for growth. Glucose is transported across the placenta by facilitated diffusion, but during maternal starvation or placental insufficiency the fetus is capable of endogenous glucose production ( ). Glucose metabolism accounts for 65% of fetal energy production, with lactate probably accounting for most of the remainder ( ). Glucose is not the only fuel utilised by the fetal brain. Studies of perfused human fetal brain have demonstrated that uptake of ketone bodies, the products of β oxidation of fatty acids, is greater than that of glucose and it is likely that the fate of ketone bodies is both incorporation into brain lipids and for use as a cerebral energy source ( ). Lactate may also be metabolised.
The fetus is usually capable of regulating its circulating glucose concentration independently of maternal glucose level and placental transfer. This capacity is seen in some cases of placental insufficiency when fetal gluconeogenesis is activated at the expense of growth and storage, and in the fetus of the mother with suboptimal control of diabetes ( Ch. 22 ) when the fetal response to the high placental transfer of glucose is increased secretion of insulin, in turn resulting in greater than normal fetal growth and storage. However, the healthy fetus differs from adults in that there is a blunted insulin response to high glucose concentrations, and that insulin secretion is more sensitive to amino acids than glucose ( ). It appears that insulin has a greater role in fetal growth than in fetal metabolic control. Similarly, the fetus is less sensitive than the neonate to the glucose-mobilising actions of glucagon, although sensitivity increases with gestational age ( ).
Under extreme circumstances fetal blood glucose control fails; for example, in cases of severe and prolonged placental insufficiency ( ). It must be questioned whether it is possible that such profound and prolonged fetal hypoglycaemia has adverse effects on the developing brain and may explain some of the disability following severe intrauterine growth restriction (IUGR), even when there have been no postnatal complications.
Metabolic changes at birth
When the continuous flow of nutrients from the placenta is abruptly discontinued, immediate postnatal metabolic changes preserve fuel supplies for vital organ function. Oxygen supply temporarily fails during delivery and anaerobic metabolism must occur: this requires higher substrate availability than aerobic metabolism. In addition, the newborn infant must adapt to the fast-feed cycle and to the change in major energy source, from glucose transfer across the placenta to using fat from adipose tissue stores and milk feeds. After birth, plasma insulin levels fall and there are rapid surges of catecholamine and pancreatic glucagon release ( ; ). These endocrine changes switch on the essential enzymes for glycogenolysis (the release of glucose stored as glycogen in liver, cardiac muscle and brain), for gluconeogenesis (glucose production from 3-carbon precursor molecules by the liver), lipolysis (release of fatty acids from adipose tissue stores) and ketogenesis (the β oxidation of fatty acids by the liver). Although glucose is the major metabolic fuel for most organs in the immediate postnatal period, there is evidence that lactate may be the preferred cerebral fuel over glucose and ketone bodies at this time ( ).
Neonatal metabolism
The metabolic switch at birth is repeated on a smaller scale during the milk-fed infant’s fast-feed cycles. Immediately after a feed there is availability of metabolic fuels, namely fatty acids and, to a lesser extent, sugars from milk. Some tissues, for example the kidney, are obligate glucose users, but others burn fatty fuels and the overall respiratory quotient falls after birth, reflecting the fact that fat oxidation accounts for about 75% of oxygen consumption. Of the organs that utilise alternative fuels to glucose, the brain is the most important in that it takes up and oxidises ketone bodies at higher rates than seen in adults, and the neonatal brain uses ketone bodies more efficiently than glucose ( ).
Any excess glucose available after a feed is stored as glycogen in the liver or converted to fat for deposition in adipose tissue, along with fatty acids absorbed after milk feeds.
Some time after each feed, the blood glucose level starts to fall and glycogenolysis and gluconeogenesis are again activated to ensure energy availability for organs which are obligate users ( Fig. 34.1 ). Glycogenolysis is an exhaustible source of glucose whose capacity varies according to fetal growth and maturity ( ) and around 2 hours after birth gluconeogenesis must become the major glucose-providing process. Stable isotope turnover studies have shown that neonatal glucose production rates are 4–6 mg/kg/min ( ). Between feeds, lipolysis and ketogenesis provide alternative fuels to glucose for organs such as the brain, which are not obligate glucose utilisers ( ). The process of ketogenesis also provides energy and cofactors which are utilised in gluconeogenesis, again highlighting the importance of fatty fuels.

The control of neonatal metabolism is dependent first on the synthesis of key enzymes, such as hepatic phosphorylase for glycogenolysis, phosphoenolpyruvate carboxykinase for gluconeogenesis and carnitine acyltransferases for ketogenesis, and, secondly, on the induction of enzyme activity by hormonal changes. Glucagon is the major neonatal glucoregulatory hormone ( ). Its concentration increases when blood glucose levels fall, and it induces activity of the enzymes of glycogenolysis, gluconeogenesis and ketogenesis in the liver. The glucoregulatory role of insulin in the neonate is less clear and may well differ from that in the adult. In most neonates, insulin does not appear to have a major influence on normal blood glucose homeostasis, but in some extreme cases (see below) high insulin concentrations may result in hypoglycaemia. Finally, it is unlikely that other hormones, such as the catecholamines, cortisol, thyroid hormones and growth hormone, are important regulators in the fast-feed cycle of the healthy neonate, but rare cases of hypopituitarism or cortisol deficiency (see below) may present with neonatal hypoglycaemia, which suggests that minimum basal levels are needed to maintain normoglycaemia.
Finally, the change from fetal to neonatal metabolism must take into account the important role of gastrointestinal adaptation. The introduction of enteral feeding has been shown to trigger the secretion of gastrointestinal regulatory peptides and hormones, which in turn induce the features of gut adaptation, namely gut growth, mucosal differentiation, induction of motor activity and the development of digestion and absorption ( ; ).
Differences between neonatal and adult metabolism
The differences between neonatal and adult metabolism are most likely to be evolutionary protective responses. Milk-fed neonates during their normal fast-feed cycle produce and utilise ketone bodies to the extent seen in adults only after a prolonged fast. Other fuels such as lactate may also be used in addition to glucose and ketone bodies. Insulin plays a lesser role in neonatal glucoregulation than in the adult, in that its release in response to glucose is blunted and delayed when compared with the adult, and that there may be end-organ insensitivities to its action ( ). In fact, healthy neonates have insulin–glucose relationships that differ markedly from those of older subjects ( ). Therefore, when investigating a neonate for possible impaired neonatal glucoregulation it is essential to have reference data from healthy infants, rather than comparing the neonatal concentrations and interrelationships of fuels and hormones with those of adults. Also, it is impossible to consider glucose alone – the availability of alternative fuels must be established. Therefore, hypoglycaemia cannot strictly be applied as a pathological diagnostic term and it is preferable to consider a diagnosis of impaired metabolic adpatation.
Hypoglycaemia
Clinical significance
The continuing controversy regarding the clinical significance of neonatal hypoglycaemia arises from a failure to consider the changes of metabolic adaptation in their totality ( ). Blood glucose levels fall immediately after birth but rise after a few hours either spontaneously or in response to feeding in healthy full-term babies ( ; ). In many babies, blood glucose levels then remain below what would be the normal range for an adult. This physiological pattern in the healthy baby with no risk factors for impaired metabolic adaptation and no clinical signs cannot be considered to be of clinical significance.
A factor not commonly recognised is that babies vary in their ability to mount protective metabolic responses when blood glucose levels are low. Indeed, there is evidence that glucose utilisation by the neonatal brain is less than in subsequent months, because of utilisation of alternative fuels ( ; ). Low blood glucose concentrations (less than 2.6 mmol/l) are commonly found during the first postnatal days in healthy appropriate-for-gestational-age (AGA) and small-for-gestational age term neonates, particularly those who are breastfed. However, these infants have high ketone body levels when blood glucose concentrations are low, and it is likely that this protects them from neurological sequelae ( ; ; ; ; ).
Therefore, it is not always appropriate to consider low blood glucose levels to represent a pathological process. However, in some circumstances, such as following preterm delivery, IUGR, perinatal hypoxia–ischaemia or suboptimal control of diabetes in pregnancy, there may be impaired ketone body production and in these babies circulating blood glucose concentrations acquire greater clinical significance and hypoglycaemia, if present, must be diagnosed and treated effectively ( ; ; ).
No study has yet satisfactorily addressed the duration of absent or reduced availability of metabolic fuels which is harmful to the human neonate. Studies in neonatal rats have demonstrated that prolonged insulin-induced hypoglycaemia, but not starvation-induced hypoglycaemia and not short-period hypoglycaemia, resulted in neurodegenerative changes ( ). A study of rhesus monkeys has shown that a duration of insulin-induced neonatal hypoglycaemia (blood glucose <1.5 mmol/l) of 6.5 hours had no demonstrable long-term effects, whereas 10 hours of hypoglycaemia was associated with ‘motivational and adaptability problems’ but no motor or cognitive deficit on testing at 8 months of age ( ).
In the light of the paucity of human neonatal data and the variability between babies with regard to exacerbating factors and protective mechanisms, it is impossible to state the duration of hypoglycaemia that is harmful to human neonates. It is very unlikely that brief self-limiting episodes are of neurological significance if not accompanied by clinical signs or coexisting clinical complications, but for some babies protective metabolic responses will fail after a period of hypoglycaemia (measured in hours rather than minutes) resulting first in abnormal neurological signs and then, in the untreated baby, brain injury ( ).
Acute neurophysiological changes at low blood glucose levels have been demonstrated in human neonates and the newborn of other species ( ; ). However, the long-term significance of these acute changes is not clear. There is no doubt that a number of infants have fits or a reduced level of consciousness when blood glucose levels are low and protective metabolic responses fail or are exhausted, and adverse long-term outcomes have been reported when neurological signs have been present ( ; ). In extreme cases, profound hypoglycaemia, usually the result of serious inborn errors of metabolism, may even result in ‘cot death’ or apparent life-threatening events. A multitude of clinical signs have often been associated with hypoglycaemia, namely tremor, irritability, ‘jitteriness’, apnoea, hypotonia, abnormal cry, tachypnoea, pallor and feeding difficulties. However, these are as likely to be the result of coexisting clinical complications, such as perinatal hypoxia–ischaemia, or the cause of hypoglycaemia (e.g. poor feeding) as the specific effects of hypoglycaemia.
No study has clearly demonstrated the independent contribution of hypoglycaemia (with or without clinical signs) to neurodevelopmental outcome because all studies to date are of neonates who had other adverse clinical factors ( ; ; ; ). There is also a paucity of information regarding the histopathological and neuroradiological changes associated with neonatal hypoglycaemia, and the reports of past studies are conflicting. However, there is evidence from case reports that profound and prolonged hypoglycaemia is associated with both transient and permanent structural changes in the brain ( ; ; ; ; ; ; ; ; ). Grey-matter damage is most commonly reported, with the parieto-occipital regions being most affected.
Impaired metabolic adaptation is likely to be most common following or coincident with other insults, such as hypoxia–ischaemia. Although concurrent hypoglycaemia and hypoxia–ischaemia are more damaging than either insult alone, there is no evidence that hypoglycaemia following cessation of a hypoxic–ischaemic insult worsens hypoxic–ischaemic injury ( ). For infants with multisystem problems (for example, after preterm delivery or perinatal hypoxia–ischaemia) all potential causes of neurological dysfunction should be prevented and treated as far as possible, and in practice the prevention of hypoglycaemia is often the easiest of clinical issues.
Unfortunately, even in cases where clinical significance is tenuous, hypoglycaemia has acquired legal and costly significance ( ). This can be avoided on many occasions by following local guidelines and paying close attention to documentation, especially of normal findings ( ; ).
Finally, the impact of hypoglycaemia and its treatment on the mother and baby must be considered. The early neonatal period is an emotionally sensitive time, and the diagnosis of hypoglycaemia may create or add to anxiety for the parents. Treatment of the infant with intravenous glucose involves separation of the baby and mother, and may be perceived as invasive or painful. The implications for the establishment of breastfeeding must also not be forgotten, especially as there is evidence that separation disrupts breastfeeding and in turn breastfeeding and avoidance of formula supplementation augment ketogenesis ( ; ; ). Therefore, emphasis should be on the early prevention of hypoglycaemia and strategies of management that do not involve the separation of mother and baby ( ; ).
Definition
Much controversy and confusion has surrounded the definition of hypoglycaemia. Some authors ( ; ; ) demonstrated that the definition varied widely not only among standard paediatric textbooks but also among neonatologists, with values given ranging from below 1 mmol/l to below 4 mmol/l. wrote: ‘The definition of clinically significant hypoglycaemia remains one of the most confused and contentious issues in contemporary neonatology.’
Previous widely used definitions were based on cross-sectional samples from newborn babies, with the assumption that the lowest blood glucose levels were abnormal. However, these definitions were proposed at a time when, unlike the present, infants were starved for considerable periods after birth, and small and preterm infants received less milk than healthy term infants. Therefore, it is not surprising that many infants on the first postnatal day had very low blood glucose levels. The current practice of early feeding of infants has been associated with a more rapid increase in blood glucose concentration after the immediate postnatal fall ( Fig. 34.2 ) ( ; ). Fortunately, the long-standing belief that the brains of preterm infants were more able to withstand low blood glucose levels than those of term infants is now less widely accepted ( ).

Later, this statistical definition of hypoglycaemia was challenged and a ‘functional’ definition was proposed, which is ‘at what level of blood glucose is the body’s function, particularly that of the brain, compromised?’ In reality, this threshold level will vary between babies, and is only of significance when protective metabolic responses are impaired and there are no alternative fuels to glucose. It is not possible to describe a functional definition of hypoglycaemia in terms of the blood glucose level at which symptoms occur because, unlike adults and older children, babies cannot complain of symptoms and by the time blood glucose levels have fallen so low that clinical signs of cerebral dysfunction have been allowed to persist, there is a risk that brain injury may have been sustained. There is no doubt that any low blood glucose concentration of any duration which causes clinical signs, such as fits or coma, is too low, regardless of its numerical value, and must be treated. In fact, the rapid clinical resolution of the signs after the intravenous administration of glucose confirms the diagnosis of acute cerebral dysfunction secondary to hypoglycaemia.
Only two studies have attempted to define the ‘safe level’ for blood glucose concentrations in neonates without apparent clinical signs ( ; ). In a neurodevelopmental follow-up study of very-low-birthweight preterm infants, found that neonatal blood glucose concentrations below 2.6 mmol/l on at least 3 days were associated with a poor neurodevelopmental outcome. The neurophysiological study of demonstrated that, in a group of subjects which included five neonates of varying birthweights and gestations, no baby with a blood glucose level above 2.6 mmol/l had abnormal sensory-evoked brainstem potentials. No differences were found in the blood glucose threshold for abnormal sensory-evoked potentials between subjects who had signs of hypoglycaemia and those who did not. Both studies concluded with the suggestion that blood glucose levels less than 2.6 mmol/l are associated with abnormal acute and prolonged neurological function, and that levels above this could be considered safe. However, these conclusions are not entirely supported by data from the studies and cannot be extrapolated to other groups of neonates ( ).
An evidence-based definition of hypoglycaemia should include the blood glucose concentration considered to be the minimum safe level, the duration beyond which the low blood glucose level is considered to be harmful, the presence of clinical signs, the group of infants studied, the consideration of alternative fuel availability, the conditions of sampling and the assay methods. Most of these criteria have never been adequately addressed by previous studies or publications ( ; ; ). This paucity of data has resulted in a pragmatic approach proposed by a group of clinicians based on thresholds for intervention rather than attempting to define hypoglycaemia as a single numerical term ( ). This group proposed that, regardless of the blood glucose concentration, neurological signs in association with low blood glucose levels should prompt investigations to establish a firm diagnosis of hypoglycaemia and its underlying cause, and the institution of urgent treatment. For infants without clinical signs but at risk of neurological sequelae by virtue of their impaired ability to mobilise ketone bodies at low blood glucose levels ( Table 34.1 ), intervention to raise blood glucose should be considered if two consecutive blood glucose levels are below 2 mmol/l (measured using accurate device) or a single blood glucose level is below 1 mmol/l.
Increased glucose utilization |
|
Inadequate glucose supply |
|
Diagnosis
The accurate measurement of blood glucose levels is essential in the diagnosis of hypoglycaemia. It is well known that glucose reagent strips, commonly used in neonatal and maternity units, are insufficiently reliable for the diagnosis ( ; ; ). Therefore, if these strips are used for neonatal screening all low values should be confirmed by accurate measurement. These samples should be assayed promptly as blood glucose levels diminish with time, even in fluoridated tubes ( ). Accurate determination may be conveniently performed using a blood glucose analyser sited in a neonatal unit laboratory. Usually whole blood samples are taken. Plasma glucose sample results are 13–18% higher than in whole blood. In terms of safety, accurate blood glucose measurements in whole blood samples will not underestimate the severity of hypoglycaemia, except in polycythaemia.
Interesting new techniques of glucose monitoring by subcutaneous microdialysis may in time reduce the need for venepuncture and heelpricks ( ). However, the clinical significance and validity of glucose measurements using these techniques are not fully evaluated.
In addition to diagnosing hypoglycaemia, the underlying cause must be determined. This is usually self-evident from the obstetric history or clinical examination, but if this is not the case and the hypoglycaemia is profound or persistent despite treatment, further investigations must be performed to identify rare but serious inborn errors of metabolism or hormone deficiencies ( Table 34.2 ). As these tests are most informative when carried out at the time of hypoglycaemia, it is important to take the necessary blood and urine samples during such episodes and process and store them if necessary out of laboratory working hours. Each unit should devise an appropriate protocol for this in liaison with local and regional specialised laboratories.
AT-RISK GROUP | MECHANISMS | MANAGEMENT |
---|---|---|
Preterm (≤36 weeks) | Low substrate stores | Early, frequent, adequate feeds |
Immature hormone and enzyme responses | Intravenous glucose if necessary | |
Fluid/energy restriction | Thermoprotection | |
Feeding difficulties | ||
Poor temperature control | ||
Intrauterine growth restriction (birthweight ≤2nd centile or clinically wasted) | Low substrate stores | Early, frequent, adequate feeds |
Immature hormone and enzyme responses | Intravenous glucose if necessary | |
Feeding difficulties | ||
Poor temperature control | ||
Infant of diabetic mother (poor antenatal control) | Hyperinsulinism | Early, frequent, adequate feeds |
Beckwith–Wiedemann syndrome | Intravenous glucose if necessary | |
Rhesus haemolytic disease | Early, frequent, adequate feeds | |
Congenital hyperinsulinaemic hypoglycaemia | Hyperinsulinism | Intravenous glucose, often >8 mg/kg/min |
Islet cell adenoma | Diazoxide | |
Somatostatin | ||
Surgery (if necessary) | ||
Moderate to severe perinatal hypoxia–ischaemia | Low substrate stores | Adequate energy provision |
‘Exhausted’ stress response | ||
Enzyme dysfunction | ||
Hyperinsulinism | ||
Fluid/energy restriction | ||
Feeding difficulties | ||
Maternal beta-blocker medication | Suppressed catecholamine release | Early, frequent, adequate feeds |
Septicaemia | ‘Exhausted’ stress response | Adequate energy provision |
Enzyme dysfunction | ||
Fluid/energy restriction | ||
Feeding difficulties | ||
Pituitary/adrenal insufficiency | Impaired cortisol response | Early, frequent, adequate feeds |
Intravenous glucose (if necessary) | ||
Inborn errors of metabolism | Defects of enzymes of glycogen production, glycogenolysis, gluconeogenesis, fatty acid oxidation | Investigate |
Adequate energy provision |
Prevalence of neonatal hypoglycaemia
Because clinical practices have changed to such an extent since the risks of hypoglycaemia were first identified, and because of the controversy surrounding definition, it is difficult to ascertain the prevalence of hypoglycaemia in at-risk groups. For example, using the definition proposed by , prevalences ranged from 5% to 7.9% in term infants and from 3.2% to 15% in preterm infants ( ; ; ; ). Using the suggested ‘safe’ level for blood glucose concentrations (2.6 mmol/l) proposed by , this group reported a prevalence of 67%, whereas a more recent study of clinically stable, AGA, term and preterm neonates reported prevalences of 10% and 4%, respectively ( ).
Mechanisms and at-risk groups
Hypoglycaemia may be secondary to increased utilisation of glucose, to inadequate endogenous or exogenous supply of glucose, or to a combination of the two ( Table 34.1 ).
Increased glucose utilisation
The most common cause of excessive utilisation of glucose is neonatal hyperinsulinism. Hyperinsulinism should be confirmed by the use of a highly specific insulin assay for plasma insulin concentrations and its interpretation with reference to normal neonatal insulin–glucose relationships ( ) ( Fig. 34.3 ). Clinical features are that glucose requirements to maintain normoglycaemia are high, in excess of 8 mg/kg/min, as compared with 4–6 mg/kg/min usually required by neonates, and the infant may be macrosomic if hyperinsulinism was of fetal origin ( Fig. 34.4 ). Investigation of suspected hyperinsulinism will demonstrate low fatty acid and ketone body concentrations during hypoglycaemia, but this feature is not specific to hyperinsulinism as some infants who are not hyperinsulinaemic, such as some who are preterm or have IUGR, also fail to mount lipolytic and ketogenic responses.


Self-limiting hyperinsulinism
Hyperinsulinism may be a temporary phenomenon when the fetus has been rendered hyperglycaemic by poorly controlled maternal diabetes (see Ch. 22 ), antenatal administration of thiazide diuretics or the administration of glucose to the mothers in labour, and in infants shortly after abrupt discontinuation of intravenous glucose infusions, after bolus doses of glucose or if glucose has been infused through an umbilical arterial catheter whose tip is close to the coeliac axis ( ; ). Rhesus haemolytic disease and perinatal asphyxia have also been associated with transient fetal and neonatal hyperinsulinism, although the aetiological link is not known ( ; ; ). It has been suggested that hyperinsulinism contributes to hypoglycaemia after intrauterine growth retardation, but normal insulin–glucose relationships (using neonatal reference data) have been demonstrated in IUGR neonates ( ; ; ).
Iatrogenic or factitious hyperinsulinism
Hyperinsulinism may result from erroneous or malicious administration of insulin. Although rare, these conditions should be suspected if hypoglycaemia is unexpected, profound or resistant to treatment.
Beckwith–Wiedemann syndrome
This condition, described independently by and , is characterised by exomphalos, macroglossia, visceromegaly, earlobe abnormalities and an increased later incidence of malignancies. Hyperinsulinism is a common but not invariable feature causing high glucose requirements in the early neonatal period; it usually resolves some time after birth. It is likely that the previously reported long-term developmental difficulties were related to undiagnosed and untreated hypoglycaemia, and it is anticipated that awareness of the condition and prevention of hypoglycaemia should result in improved outcome.
Congenital hyperinsulinaemic hypoglycaemia
Although a rare condition, this is the most common cause of recurrent and persistent hypoglycaemia in infancy and childhood ( ). It is usually associated with macrosomia and always with extreme hyperinsulinism and high glucose requirements. The condition may be self-limiting in the neonatal period but more often extends beyond this time. As there is no protective ketone body response to hypoglycaemia, there are usually neurological signs and the sk of brain injury is high. Therefore, urgent treatment is required (see below). Many descriptive terms, such as ‘nesidioblastosis’, ‘islet-cell dysregulation syndrome’ or ‘persistent hyperinsulinaemic hypoglycaemia of infancy’, have been applied to the condition over the years. Currently, histological classification is into diffuse and focal forms ( ).
The condition is more often familial than sporadic. Several underlying pathologies have been demonstrated ( ). In severe cases of hyperinsulinaemic hypoglycaemia there are mutations in the genes encoding for subunits of the K + ATP channel and the involvement of the pancreas is diffuse. The functional loss of this channel results in dysregulation of calcium fluxes and thus unregulated insulin release. Other forms of hypersinsulinism, which tend to be milder or present later, are linked to defects in genes coding for glutamate dehydrogenase and glucokinase, with activation of these genes in turn affecting the function of the beta cell. The former is the second most common form of congenital hyperinsulinaemic hypoglycaemia and is associated with hyperammonaemia, so ammonia levels should always be measured when hyperinsulinaemia is suspected ( ). Other mutations are those coding for hepatocyte nuclear factor-4 α protein and HADH (a mitochondrial enzyme of fatty acid metabolism) ( ).
Rarely, an isolated islet-cell adenoma may present with neonatal hyperinsulinaemic hypoglycaemia.
Recognition of hyperinsulinism and early prevention and treatment of hypoglycaemia, with referral to a specialist centre, are essential to reduce the incidence of permanent neurological damage, which has been widely reported ( ; ; ; ).
‘Leucine-sensitive hypoglycaemia’
This was previously described as a distinct entity, but it is more likely that hypoglycaemia in response to leucine administration represents underlying hyperinsulinism sensitive to protein and should be investigated and treated as such ( ).
Insufficient supply of glucose
Hypoglycaemia is most often the result of reduced delivery of glucose into the blood. In the enterally fed infant all the circulating glucose is provided either by the absorption and conversion of sugars or by glycogenolysis and gluconeogenesis, and in some babies there may be a contribution from intravenous glucose infusion. Thus, if the infant fails to switch on glycogenolysis or gluconeogenesis in response to falling blood glucose levels, or clinicians prescribe insufficient intravenous glucose, hypoglycaemia may occur. This will be most significant when production of alternative fuels to glucose is also impaired. Three possible mechanisms may cause the failure of glucose production: (1) reduced availability of gluconeogenic precursors; (2) reduced activity of enzymes of glycogenolysis and gluconeogenesis; or (3) impaired counterregulatory hormone response.
Reduced availability of gluconeogenic precursors
Glycogenolysis and gluconeogenesis may be limited by availability of glycogen, gluconeogenic precursors or the energy provided by fatty acid oxidation. This may occur after preterm delivery, IUGR, maternal alcohol abuse or perinatal hypoxia–ischaemia, or as a consequence of inadequate substrate intake after birth ( ; ; ).
Reduced activity of enzymes of glycogenolysis and gluconeogenesis
There may be failure of synthesis and activation of the key enzymes described above, and enzymes of lipolysis and ketogenesis will also be affected. This may be the result of a specific inherited metabolic disorder, in which case hypoglycaemia is usually severe, and recurrent or persistent, or there may be generalised immaturity of enzymes, as in preterm infants. The infant is resistant to the effects of the postnatal surges of counterregulatory hormones. Finally, enzyme activity may be suppressed by acquired conditions, such as perinatal bacterial infection or impaired liver function. Defective gluconeogenesis may also be the cause of hypoglycaemia complicating cases of congenital heart disease and cold injury ( ; ).
Impaired counterregulatory hormone response
This will result in failure to activate enzymes of glycogenolysis, gluconeogenesis, lipolysis and ketogenesis. Hyperinsulinism has a dual mechanism in that glucose utilisation is increased (see above) but also counterregulatory hormone release is inhibited. Failure of release of counterregulatory hormones may play a role in hypoglycaemia in preterm and IUGR babies, and after maternal medication with beta-blockers in pregnancy ( ; ). Finally, there may be rare permanent disorders which result in insufficiency of counterregulatory hormones, for example low growth hormone and cortisol levels in septo-optic dysplasia and congenital hypopituitarism, and low glucocorticoid levels in adrenocortical deficiencies ( ; ; ).
Mechanisms of hypoglycaemia vary among groups of infants, and for some there may be more than one aetiological mechanism ( Table 34.2 ). This is most applicable to neonates who have been subject to IUGR, which for many reasons may result in failure of glycogenolysis and gluconeogenesis after birth. Animal and clinical studies have demonstrated that IUGR may reduce the availability of alternative fuels for cerebral metabolism ( ; ). However, another clinical study has shown that healthy breastfed IUGR babies can mount a ketogenic response, and that excessive formula milk supplementation may be the cause of the suppressed response ( ). It is important to note that not all IUGR infants will be ‘small for gestational age’ (see Ch. 10 ), and clinical examination is important for the identification of the ‘wasted’ neonate with disproportionate birthweight and head circumference centiles. Conversely, not all small-for-gestational-age infants will have been subject to placental insufficiency – they may be constitutionally small and will not experience impaired postnatal metabolic adaptation. In summary, the early identification of at-risk neonates and the understanding of underlying mechanisms of hypoglycaemia are important for the diagnosis and treatment of the disorder.
Prevention and management of neonatal hypoglycaemia ( Table 34.3 )
Normal babies
As described above, healthy full-term AGA neonates often have low blood glucose concentrations in the first postnatal days, but are protected by the presence of ketone bodies and lactate as alternative fuels. Thus, it is now recognised in Europe and North America that, for this group, it is not appropriate to carry out routine blood glucose monitoring, to label low blood glucose as a pathological entity or to initiate treatment which is invasive or which may interfere with the establishment of breastfeeding ( ; ; ; ). Because of the healthy infant’s ability to counterregulate, problems with establishment of successful breastfeeding are equally likely to present with excessive weight loss (in excess of 10% birthweight), dehydration and jaundice as with clinically significant hypoglycaemia. Therefore, breastfeeding advice and intervention should not be based on blood glucose levels, but on full assessment of the baby – proceeding to blood glucose measurement if there are clinical concerns. Midwives and doctors must be alert to the possibility that other conditions, such as infection or, more rarely, inborn errors of metabolism, may present with the neurological signs and hypoglycaemia and specific investigations should be performed ( Table 34.4 ).
1. Identify at-risk infants (see Table 34.2 ) | |
2. Early energy provision (within 1 hour of birth) | |
If enteral feeding planned: | If enteral feeding contraindicated |
Breastfeed if mother’s wish | IV 10% glucose infusion of at least 3 ml/kg/h |
| |
3. Blood glucose monitoring | |
| |
4. Maintain energy provision | |
Increase feed interval if blood glucose ≥2.0 mmol/l (3.0 mmol/l for hyperinsulinism) | |
Mother plans to breastfeed: | Mother plans to bottle-feed: |
Encourage kangaroo care and unlimited access to breast | Start at 100 ml/kg/day |
Offer breast before formula feeds | Demand feed when blood glucose ≥2.0mmol/l (3.0 mmol/l for hyperinsulinism) |
| |
IV therapy: | |
| |
5. If blood glucose <2.0 mmol/l on at least two occasions but no clinical signs | |
If enterally fed: | If IV glucose already running: |
Increase feed volume and frequency | Increase infusion rate or concentration |
| |
6. If blood glucose <1.0 mmol/l and/or major clinical signs, e.g. fits/coma | |
| |
7. If hypoglycaemia is severe or persistent | |
Investigate as in Table 34.4 | |
8. Summary | |
|
SAMPLE | ASSAY | DIAGNOSIS |
---|---|---|
Blood | Glucose * | Confirm diagnosis |
Blood | pH * | Lactic acidosis in: glucose-6-phosphatase deficiency, fructose-1,6-diphosphatase deficiency, pyruvate carboxylase deficiency, phosphoenolpyruvate carboxykinase deficiency |
Lactate * | Acidosis in disorders of amino acid metabolism | |
Blood | Intermediary metabolites | Disorders of gluconeogenesis |
Blood | Ketone bodies | Disorders of fatty acid β oxidation (NB. low ketone body levels in preterm, intrauterine growth restriction and hyperinsulinaemic infants) |
Plasma | Ammonia | Disorders of amino acid metabolism |
Hyperinsulinism | ||
Plasma | Fatty acids | Disorders of fatty acid β oxidation |
Plasma | Insulin † | Hyperinsulinism |
Plasma | Glucagon | Isolated hormone deficiency or in association with others, e.g. septo-optic dysplasia |
Catecholamines | ||
Corticosteroids | ||
Growth hormone | ||
Plasma/urine | Amino acid profile | Disorders of amino acid metabolism |
Urine | Organic acids | Disorders of fatty acid β oxidation |
Fibroblasts/leukocytes | Enzyme activities | Selected inborn errors of metabolism |
* Analysers available for use in neonatal unit laboratory.
At-risk babies ( Table 34.2 )
For practical purposes, the following discussion focuses only on the infants who are at risk of the impaired metabolic adapatation and the neurological sequelae of hypoglycaemia ( Table 34.2 ). Early prevention of hypoglycaemia is optimal for these infants, so the first step in management must be to identify them. Although this is easy in some cases (such as the preterm baby), for others clinical observations are important (for example, to identify the wasted appearance of the growth-restricted neonate who may not necessarily have a low birthweight).
At-risk infants should have regular pre-feed blood glucose monitoring (at least 4–6-hourly initially). In addition, it is imperative that any infant with neurological signs, even if not in an at-risk group, should have urgent, accurate blood glucose measurement. The monitoring schedule for at-risk infants will vary according to local protocols, but we suggest that monitoring should be commenced before the second feed and that pre-feed monitoring be continued until the infant has had at least two satisfactory measurements. Monitoring should be recommenced if the infant’s clinical condition worsens or energy intake decreases. If monitoring is by reagent strip, low levels must be confirmed by accurate measurement (see above).
The importance of early milk feeding has been appreciated for many years ( ). Both breast and formula milks provide important gluconeogenic precursors and fatty acids for β oxidation. As they contain sources of energy other than carbohydrate they have a higher joule/ml content than 10% dextrose. In addition, enteral milk feeding stimulates the secretion of gut hormones, which may facilitate postnatal metabolic adaptation ( ). Therefore, all infants who are expected to tolerate enteral feeds should be fed with milk as soon as possible after birth, and at frequent intervals thereafter. Babies who are capable of sucking should be offered the breast at each feed (if this is the mother’s wish). If it is likely that babies will need complementary or supplementary feeds, maternal breast milk expression should be encouraged. The need for formula supplementation will vary between babies, will diminish with the successful establishment of breastfeeding, and will be guided by the availability of expressed breast milk, regular pre-feed blood glucose monitoring, the clinical condition of the baby and assessment of breastfeeding. In the breastfed baby, formula intake should be kept to the minimum necessary, so as to enhance breastfeeding and avoid suppression of normal metabolic adaptation ( ).
In the at-risk baby who is establishing oral feeds there is a potential nadir at which body stores are steadily reducing but milk feeds have not yet started to replenish these stores. Even if the baby is feeding well, this may not occur until at least 48 hours. For this reason, vulnerable babies should not be transferred to the community at less than 48 hours, and only when experienced staff are satisfied that feeding is effective.
When full enteral feeding is not anticipated, for example in the very preterm or sick infant, an intravenous glucose infusion should be commenced as soon as possible after birth. Usually 10% dextrose at 3 ml/kg/h (5 mg glucose/kg/min) is sufficient to prevent hypoglycaemia, but in some cases (such as hyperinsulinism) more is required. If the amount of glucose administered is limited by fluid restriction, more concentrated dextrose solutions may be required and central venous lines should be used, because these solutions are sclerotic to peripheral veins and cause tissue damage if they leak.
If low blood glucose levels persist or are associated with clinical signs in the milk-fed infant despite the above measures, it may be possible to increase further the volumes and/or frequencies of feeds. If this is not possible, or if the hypoglycaemia is resistant to this strategy, intravenous glucose will be required. If the infant is tolerating milk feeds these should be neither stopped nor reduced. The initial rate of 10% glucose infusion should be 3 ml/kg/h (5 mg/kg/min; Table 34.5 ), but adjusted according to frequent accurate blood glucose measurements. If the need for fluid restriction limits the amount of glucose that may be given, more concentrated solutions may need to be infused ( Table 34.5 ). If hypoglycaemia persists despite intravenous glucose, it is important to check the infusion site and the infusion apparatus to confirm glucose delivery. Leaking drips should be promptly resited. Boluses of concentrated glucose solution should be avoided because of the risk of rebound hypoglycaemia and cerebral oedema ( ); if boluses are required (for example, if there are neurological signs of hypoglycaemia), they should be of 10% dextrose (3–5 ml/kg), given slowly, and always followed by an infusion. All reductions in infusion rate should be gradual. In cases of hyperinsulinism, intramuscular glucagon will have a temporary glycaemic effect if there is delay in siting an intravenous infusion (see below).
RATE OF INFUSION | STRENGTH DEXTROSE SOLUTION (mg/kg/min) | ||||
---|---|---|---|---|---|
ml/kg/24 h | ml/kg/h | 4% | 10% | 15% | 20% |
60 | 2.5 | 1.7 | 4.2 | 6.2 | 8.4 |
72 | 3.0 | 2.0 | 5.0 | 7.5 | 10.0 |
80 | 3.3 | 2.2 | 5.6 | 8.3 | 11.2 |
100 | 4.2 | 2.8 | 6.9 | 10.4 | 13.8 |
120 | 5.0 | 3.3 | 8.3 | 12.5 | 16.6 |
150 | 6.3 | 4.2 | 10.4 | 15.6 | 20.8 |
180 | 7.5 | 5.0 | 12.5 | 18.7 | 25.0 |
200 | 8.3 | 5.6 | 13.9 | 20.8 | 27.8 |
Specific treatments
Hyperinsulinism
It should be stressed again that, when hyperinsulinism is not self-limiting and requires or is resistant to very high glucose infusion rates, referral to a specialist centre must be made ( ). The treatments outlined below should only be administered in non-specialist units on the advice of a specialist centre and as a holding measure pending transfer. The risk of precipitating heart failure, especially if there is a coexisting hypertrophic cardiomyopathy, must be considered.
Glucose delivery should be prescribed to maintain blood glucose levels above 3 mmol/l and early siting of an umbilical venous catheter or venous central line is essential to allow adequate delivery rates. If hypoglycaemia is still resistant to high glucose delivery rates, it is possible to administer diazoxide (10–20 mg/kg/day), which suppresses pancreatic insulin release. The effect is optimal if a daily dose of chlorthiazide (7–10 mg/kg) is given to potentiate the hyperglycaemic effect and prevent the fluid-retentive effect of diazoxide. In cases of persistent hyperinsulinism response to diazoxide is variable, patients with mutations in the genes coding for HI-GK and HI-GLUD tend to show the best response.
Work defining the molecular basis of hyperinsulinism has demonstrated that some cases respond to the calcium channel blocker nifedipine ( ; ).
Somatostatin analogue (octreotide, Sandostatin, Sandoz Pharmaceuticals) administered intravenously or subcutaneously at a dose of 10 µg/kg/day also suppresses insulin release ( ). However, tolerance may develop and there is concern about possible effects on the secretion of other hormones, and for this latter reason glucagon is administered simultaneously at a dose of 1 µg/kg/h ( ).
Glucagon (200 µg/kg bolus intravenously or intramuscularly or infusion 5–10 µg/kg/h) has a temporary glycaemic effect via its glycogenolytic action and given alone may be a useful holding measure, for example when resiting glucose infusions. However, its prolonged use is limited because glucagon further stimulates insulin release.
Some cases of neonatal hyperinsulinism, especially those caused by recessive mutations of the genes encoding the SUR1 and Kir6.2 proteins, are unresponsive to medical treatment and near-total pancreatectomy is required. Referral should be made to specialist surgical centres. In specialist centres, rapid genetic testing allows clinicians to identify those who are likely to have focal disease and then 18 F- l -DOPA positron emission tomography imaging is indicated for the preoperative identification and precise anatomical location of focal lesions ( ; ). This is followed by laparoscopic or open surgery for targeted focal pancreatectomy.
Intrauterine growth restriction
Glucagon has a potential role when hypoglycaemia is secondary to IUGR. It appears that its mechanism of action when given in pharmacological doses (30–200 µg/kg) is to mimic the postnatal glucagon surge and the ‘switching on’ of the enzymes of gluconeogenesis ( ). Thus it is a useful adjunct to intravenous glucose therapy.
Adrenocortical insufficiency
Although parenteral hydrocortisone has been used for many years for the treatment of hypoglycaemia of various aetiologies, its place is solely as a replacement therapy for cortisol deficiency.
Inborn errors of metabolism
The management of the rare inborn errors of metabolism varies according to diagnosis and is beyond the scope of this chapter. In general, the aim is to provide adequate calories to prevent hypoglycaemia and catabolism.
Summary
The prevention and management of hypoglycaemia in babies at risk of impaired metabolic adaptation depend upon the administration of sufficient energy via either enteral or parenteral routes. In fact, many cases of hypoglycaemia are iatrogenic as a result of a failure to ensure adequate intake for at-risk babies, or to prescribe sufficient glucose to fluid-restricted babies. Correcting these deficiencies is usually sufficient and only rarely are additional treatments required.
Neonatal hypoglycaemia is a common but usually preventable condition. Prompt recognition of risk factors and prevention and management are important to reduce the risk of neurological sequelae.
Hyperglycaemia
Neonatal hyperglycaemia has been recognised for over a century ( ) and during this time it has become apparent that it represents several distinct clinical entities. As with hypoglycaemia, much uncertainty exists regarding definition, clinical significance and treatment.
Neonatal diabetes mellitus
Diabetes mellitus has been described as first presenting in the neonatal period (and onset may be after discharge from the neonatal unit or maternity ward). It was the subject of a British Paediatric Surveillance Unit study and there are now exciting data regarding the genetics of the condition ( ; ; ; ). The condition is rare (1 : 500 000) ( ). Early reports suggested that the condition was usually transient, characteristically occurring in small-for-gestational-age infants in the first 6 postnatal weeks and presenting with very high blood glucose levels, low plasma insulin concentrations, dehydration, fever and failure to thrive despite adequate feeding ( ; ; ). The mean duration of insulin therapy, if required, was 69 days for the transient form, and it was thought that very few infants developed permanent diabetes in later life ( ). However, more recent reviews of reported cases of neonatal diabetes mellitus have confirmed its occurrence in predominantly small-for-gestational-age infants. They demonstrated that 46% developed permanent diabetes in the neonatal period, 23% developed permanent diabetes in childhood or adolescence and in 31% diabetes resolved in the neonatal period. Ten cases had coexisting clinical conditions and six families had more than one affected individual (including two pairs of twins) ( ; ).
Self-limiting neonatal hyperglycaemia
Neonatal hyperglycaemia is most often a transient disorder which resolves spontaneously and has few features in common with classic diabetes mellitus. The prevalence of transient hyperglycaemia appears to be increasing in parallel with the increased survival of extremely low-birthweight infants and the early use of parenteral nutrition solutions and corticosteroid therapy in these babies ( ). Iatrogenic or factitious hyperglycaemia must also be considered. The following sections refer to the most common condition, transient hyperglycaemia in small or sick infants.
Clinical significance
It is of the utmost importance to remember that neonatal hyperglycaemia may be a sign of a serious underlying disorder, such as infection. However, despite reports of associations between hyperglycaemia and adverse outcomes in exteremely low-birthweight babies, it is still not known whether the high glucose concentrations themselves place the infant at further risk or whether the high blood glucose levels simply reflect the fragile and unstable condition of the babies most at risk of adverse outcome ( ). Unlike adults with insulin deficiency, hyperglycaemic neonates do not develop ketosis or metabolic acidosis ( ). There is a risk that glycosuria and osmotic diuresis may cause fluid and electrolyte imbalance with dehydration. These disturbances are more likely to be comorbidities rather than the result of hyperglycaemia as studies of large numbers of infants have reported that osmotic diuresis is not an invariable consequence of neonatal hyperglycaemia ( ; ; ; ). There is also concern that changes in blood osmolality and fluid shifts may result in cerebral damage. However, cerebral pathology and adverse neurodevelopmental outcome have never been demonstrated to occur as the direct result of hyperglycaemia, and it is thought that blood glucose levels above 20 mmol/l are required to exert significant osmolar effects ( ; ; ).
As described above, neonatal hyperglycaemia is clinically significant in that it may herald a serious underlying disorder. Once such disorders have been ruled out and treated, there is no evidence that self-limiting hyperglycaemia secondary to immaturity of glucoregulation or excessive glucose intakes and not associated with osmotic diuresis has adverse effects at blood glucose levels below 20 mmol/l.
Definition and diagnosis
There is no established definition of neonatal hyperglycaemia, but blood glucose levels above 7 mmol/l are usually considered to be above the normal range and the majority of units recently surveyed define hyperglycaemia as a blood glucose level above 10 mmol/l ( ). However, the upper ‘safe’ limit of blood glucose concentration in the neonate is likely to be above this level. As with hypoglycaemia, there is great variation in terms of the diagnosis and management of hyperglycaemia ( ).
Glucose reagent strips are more useful in the diagnosis of hyperglycaemia than for hypoglycaemia because the strips are more reliable at high blood glucose levels, and inaccuracies of 0.5–1.0 mmol/l are of less clinical relevance in the context of hyperglycaemia. It may also be useful to monitor urine for glycosuria, but it should be remembered that neonates, particularly those who are preterm, have a low renal threshold for glucose and fractional excretion of glucose varies widely, so that glycosuria may be present even in normoglycaemia and is independent of circulating blood glucose concentration ( ; ).
Prevalence
Without a clear definition of hyperglycaemia it is difficult to comment on its frequency. Studies of prevalence vary according to their subjects, with hyperglycaemia found most frequently in very low-birthweight and preterm infants ( ; ). Small-for-gestational-age infants who are preterm are more at risk for developing hyperglycaemia than hypoglycaemia when receiving standard intravenous infusions ( ). Reported prevalences vary from 29% to 86% in very low-birthweight neonates ( ; ).
Mechanisms and at-risk groups
The mechanisms underlying neonatal hyperglycaemia vary and, as with hypoglycaemia, are best understood with reference to the expected metabolic changes at birth. In contrast to hypoglycaemia resulting from a low glucose production rate or a high glucose uptake rate, hyperglycaemia may be the result of a high glucose production or infusion rate or a low glucose uptake rate.
Neonatal hyperglycaemia is usually secondary to a high glucose appearance rate, that is, failure of the baby to suppress endogenous glucose production even if glucose infusion rate is high ( ; ; ). To maintain control, the infant must be able to adapt to the exogenous administration of glucose by suppressing glucose production by the liver. The ability to glucoregulate in this way has been demonstrated in normoglycaemic neonates ( ). However, there is evidence from clinical and animal studies that some neonates do not suppress glucose production in response to glucose infusion and/or increased blood glucose levels ( ; ; ).
The inability to suppress gluconeogenesis may in turn be the result of disordered glucoregulatory hormone control. Although the glucoregulatory role of insulin in the neonate is unclear and may vary between infants, it has been suggested that hyperglycaemia results from decreased insulin secretion in immature subjects ( ; ). This is analogous to the adult insulin-dependent diabetic. Animal studies have also shown that, after chronic hyperglycaemia, the fetal pancreas cannot mount an insulin response to a further glucose surge ( ). This may be analogous to the condition in preterm babies receiving constant high-rate glucose infusions, whose pancreatic response to hyperglycaemia may be ‘exhausted’.
Alternatively, circulating insulin concentrations may be appropriate for the blood glucose concentration, but hyperglycaemia may result from end-organ insensitivity to insulin. This is analogous to type 2 diabetes, which is characterised by insulin resistance. Neonatal insulin resistance has been demonstrated by the persistence of hyperglycaemia in the presence of raised insulin concentrations, by the poor hypoglycaemic response to large exogenous doses of insulin and by the high insulin concentrations needed to suppress gluconeogenesis ( ; ; ; ). Insulin resistance may be secondary to immaturity or downregulation of peripheral receptors, to the effect of high fatty acid levels resulting from infusion of fat emulsion or to the peripheral actions of counterregulatory hormones ( ).
Clinical data demonstrate that some hyperglycaemic preterm infants have inappropriately low plasma insulin concentrations and high plasma catecholamine levels, whereas others have apparently appropriate insulin concentrations and may have insulin resistance ( Fig. 34.5 ) (Hawdon et al. unpublished data). The former group were those who had other clinical complications such as infection, and the latter were either very preterm or preterm and small for gestational age.

The excess secretion of counterregulatory hormones, which themselves stimulate glycogenolysis and gluconeogenesis, may in addition block the secretion of insulin and inhibit its peripheral action, thereby contributing to insulin resistance ( ). This is the mechanism of hyperglycaemia secondary to exogenous corticosteroids, sometimes administered in large doses to neonates with lung disease (The ). It has even been suggested that antenatal corticosteroid administration contributes to the failure to suppress postnatal gluconeogenesis ( ). Aminophylline, used for the prevention of apnoea of prematurity, mimics the action of catecholamines and induces glycogenolysis ( ). To date, growth hormone has not been implicated in the aetiology of neonatal hyperglycaemia ( ).
These hormonal disturbances may be the consequence of underlying clinical stresses such as infection, respiratory distress, pain or surgery ( ; ; ; ; ; ). Studies by ; ; ) demonstrated that, with minimal anaesthesia for major surgical procedures in term and preterm neonates, high glucagon and catecholamine levels and inhibition of insulin secretion led to a number of metabolic abnormalities, including hyperglycaemia and hyperlactataemia. This response was in proportion to the severity of surgical stress and could be prevented by the addition of opioid analgesia or halothane to anaesthetic regimens. In some conditions associated with severe clinical stress, such as perinatal asphyxia, hyperglycaemia may occur as the result of high counterregulatory hormone concentrations but hypoglycaemia is more often seen, probably because the latter represents the situation found after the stress response is exhausted.
Despite the frequency with which hyperglycaemia is now observed, the aetiology and optimal management of this metabolic disorder have not been established. Clinical, animal and laboratory studies suggest that glucose production is not suppressed in the face of hyperglycaemia, but there are conflicting data regarding the role of defective glucoregulatory hormone responses.
Prevention and management ( Fig. 34.6 )
As neonatal hyperglycaemia is usually self-limiting and not associated with adverse sequelae, many clinicians choose not to treat raised blood glucose concentrations aggressively. However, the first step in management, especially in a baby who has previously been normoglycaemic, must be to seek and treat serious underlying disorders. The second step is to prevent the occurrence of high blood glucose concentrations secondary to high glucose infusion rates by instituting careful management of intravenous fluid prescriptions. Clinicians often increase fluid infusion rates to counter renal and extrarenal losses in the immature neonate. The condition is usually self-limiting and may be prevented by the more gradual increase in glucose intake in those at risk. For example, 200 ml/kg/day of 10% dextrose provides 14 mg/kg/min glucose, which is well in excess of the neonate’s requirements. Thus it is not surprising that hyperglycaemia occurs and the extra glucose given is ‘wasted’. Therefore, glucose infusion rates should be calculated and, if they are found to be excessive (for example, above 4–6 mg/kg/min), more dilute solutions should be used.

Hyperglycaemia may still occur in some neonates who are clinically stable and who are not receiving excessive glucose intakes. These infants are usually of extremely low birthweight and less than 1 week old. Often they have received early parenteral nutrition and thus fairly high rates of glucose infusion in combination with amino acids. At the same time they may have high counterregulatory hormone levels, rendering them ‘catabolic’ with or without peripheral insulin resistance, so that they cannot utilise the infused substrates. The condition is usually self-limiting and may be prevented by the more gradual increase in glucose intake in those at risk.
There are three strategies for management of hyperglycaemia when it occurs in these circumstances.
First, moderate hyperglycaemia may be ‘tolerated’ if it does not appear to be causing osmotic diuresis. In most instances the condition resolves within a few days even if no action is taken.
Second, the rate of glucose infusion may be carefully reduced to the rate at which blood glucose levels become normal, and then gradually increased as tolerated. This carries the possible disadvantage of reducing the infant’s energy intake, but it is likely that immature infants are unable to utilise effectively all the glucose offered, especially if at a rate in excess of 5 mg/kg/min ( ).
Third, insulin may be administered in order to lower the blood glucose concentration without reducing the glucose infusion rate. If insulin is to be used, there needs to be priming of infusion tubing as insulin is adsorbed to plastic to a variable extent. Controlled studies of insulin administration to adult intensive care patients on intravenous nutrition have demonstrated that, although there is a short-term improvement in nitrogen balance, there is no advantage in terms of either weight gain or body composition, and that a number of patients become hypoglycaemic ( ). Although there are a number of reports of this practice in the neonatal literature, there is no consistency regarding the clinical situations in which insulin has been given, only short-term outcome measures have been reported and there are few prospective controlled trials ( ; ; ; ; ). All of these studies reported hypoglycaemia in some infants even after the discontinuation of insulin infusion. Therefore, insulin should not be prescribed without availability of prompt and accurate blood glucose testing. Recent systematic reviews have not supported the strategy of routinely infusing insulin to achieve a specific glucose delivery rate or prevent neonatal hyperglycaemia, and recommend reserving the use of exogenous insulin for infants with severe hyperglycaemia (above 14–16 mmol/l) because the safety of the practice has not yet been determined ( ; ).
There is marked variation between and within studies regarding the doses of insulin required, and some authors have raised the possibility of the development of tolerance in some babies, so that hyperglycaemia recurs despite large increases in insulin dosage ( ; ; ). Considering the clinical observation that without insulin treatment neonatal hyperglycaemia is usually a transient and self-limiting condition, it may be that insulin administration in some way hinders spontaneous recovery.
Epidemiological studies have suggested that intrauterine nutritional and endocrine status may influence adult metabolism and susceptibility to disease ( ). The long-term clinical significance of early high energy intakes in association with large doses of exogenous insulin in the preterm neonate, and of possible up- or downregulation of insulin receptors, has not yet been considered.
Finally, the introduction of enteral feeding with small volumes of milk as soon as the infant’s gastrointestinal tract will tolerate this may hasten the control of blood glucose homeostasis by inducing surges of gut hormones which promote insulin secretion (the enteroinsular axis) ( ).
Accidental hyperglycaemia should be prevented by strict attention to stocked glucose solutions, pharmacy total parenteral nutrition production quality control and glucose infusion devices.
The pathogenesis of neonatal hyperglycaemia and the mechanism of action of exogenous insulin administration are not clearly understood. Therefore, the increasing practice of prescribing insulin to neonates without understanding its mode of action is of concern. It is not known whether insulin administration promotes linear growth in neonates, or merely converts glucose into fat. Further prospective randomised controlled trials of insulin therapy in preterm neonates who become glucose-intolerant would assess the impact of insulin therapy on glucose tolerance, metabolism and growth in the short and long term. It is currently impossible to judge whether insulin therapy does confer clinical advantage over expectant management of a condition which is usually self-limiting.
Summary
An approach to the controversy surrounding the definition, diagnosis and management of babies with neonatal hypoglycaemia has been presented. The present pragmatic approach, based on the available evidence and clinical experience, is unlikely to be established on a more secure scientific basis in the near future. The following summarises the key questions that still need to be addressed.
First, the long-term effects of moderate hypoglycaemia must be established. For example, we do not know whether the preterm infant’s brain is more or less vulnerable to hypoglycaemia than that of the term infant, what duration of hypoglycaemia results in permanent disability and whether the effects of hypoglycaemia are exacerbated by concurrent complications such as hyperbilirubinaemia. We do not know whether the differences that have been demonstrated between preterm and term infants in metabolic adaptation persist beyond the first postnatal week, and whether there are implications for the preterm infant in terms of persistent impairment of metabolic responses.
Second, more information must be gathered relating to other factors regulating glucose availability to the brain, namely cerebral blood flow, the ontogeny of glucose transporter proteins and the role of the astrocyte in neuronal metabolic support.
Finally, as we realise the inadequacy of glucose reagent sticks for the diagnosis and monitoring of hypoglycaemia, improved accurate near-patient systems should be developed for the measurement of blood glucose concentrations and the ability to measure blood ketone body concentrations in these circumstances would markedly enhance management.
In urged the physician caring for children to be constantly aware of the risk of hypoglycaemia. This exhortation is still relevant in the 21st century, but much still needs to be learned and there are many challenging areas of research for the clinical investigator.
Acknowledgement
The author wishes to thank Dr K Hussain for contributing to the section on hyperinsulinism.
Endocrine disorders
- Tim Cheetham
- Daniel J Schenk
Endocrine disorders in the newborn may be serious and potentially life-threatening, but are nearly always treatable. It is therefore particularly important that they are identified as soon as possible.
Advances in the field of molecular biology have now clarified the cellular basis of many endocrine disorders but this chapter will focus on the presentation, differential diagnosis and management of these conditions. For fuller discussions of the clinical and molecular aspects of endocrine disease the reader is referred to works on paediatric or general endocrinology, such as , , ) or .
Hypothalamus and anterior pituitary
Normal function
The hypothalamus and anterior pituitary form a neuroendocrine unit that mediates between the central nervous system (CNS) and peripheral tissues. The hypothalamic nerve fibres release humoral substances into the capillaries of the primary plexus in the median eminence, to be carried by the portal vessels to excite or inhibit the secretion of the cells of the anterior pituitary. The principal hypothalamic-releasing hormones are corticotrophin-releasing hormone (CRH), thyrotrophin-releasing hormone (TRH), gonadotrophin-releasing hormone (GnRH) and growth hormone-releasing hormone (GHRH), with two inhibitory hormones, somatostatin and dopamine. These factors regulate the secretion of the pituitary trophic hormones adrenocorticotrophic hormone (ACTH), thyroid-stimulating hormone (TSH), follicle-stimulating hormone (FSH), luteinising hormone (LH), growth hormone (GH) and prolactin into the circulation. They, in turn, stimulate the production of cortisol, thyroxine (T 4 ), sex steroids and insulin-like growth factor-I (IGF-I), which will then feed back and modulate hypothalamic and pituitary hormone release. Many other neurotransmitters and neuropeptides are involved in the regulation of pituitary hormone release and there is considerable interaction between the various axes; GH production is, for example, modulated by T 4 , cortisol and sex steroids as well as by IGF-I.
Hypothalamic and anterior pituitary disease
Children with congenital pituitary hormone deficiency (PHD) have quantitative and qualitative abnormalities of the production of one or more pituitary hormones and represent a spectrum of hormonal and associated midline abnormalities. At one end of the spectrum is the child with isolated GH deficiency and a structurally normal brain. At the other end of the spectrum is the patient with septo-optic dysplasia (SOD) and associated combined PHD (CPHD) ( ; ). Many children with ‘hypopituitarism’ have an abnormal hypothalamus which cannot manufacture and release the relevant hypothalamic hormones appropriately. The incidence of isolated GH deficiency is approximately 1 in 4000–10 000 ( ; ) and that of SOD around 1 in 10 000 ( ). Inheritance of CPHD may be recessive, dominant or X-linked and chromosomal defects such as 18p – may be associated with PHD ( ).
There is an association between PHD and midline CNS malformations as well as midline malformations elsewhere in the body such as cleft lip or cleft palate ( ; ) or a single central incisor ( ). More than 90% of those with CPHD have midline CNS abnormalities on magnetic resonance imaging (MRI). These include an abnormal pituitary gland and/or hypothalamus as well as the absent septum pellucidum and optic nerve hypoplasia characteristic of SOD ( ; ; ). The fact that SOD is more common in areas of high unemployment and high teenage pregnancy has led to suggestions that environmental factors may play a role in disease development ( ). A normal MR in infancy and the MR from a patient with combined PHD is shown in Figure 34.7 .

Holoprosencephaly is a midline brain malformation where the forebrain fails to develop into separate hemispheres correctly. Holoprosencephaly is associated with aplasia of the olfactory bulbs and craniofacial abnormalities and patients may also have anterior and posterior PHD. Patients may have an underlying chromosomal abnormality ( ) and a number of single-gene defects have been identified in these babies ( ; ). The prevalence appears to be higher in young mothers ( ) and in mothers with diabetes ( ). MR images may show an abnormal pituitary gland as well as the characteristic forebrain abnormalities seen in this disorder.
Hypopituitarism has been linked to traumatic delivery in the past and there is a high incidence of breech and forceps delivery in some series ( ). calculated that a male firstborn delivered by breech has an 11-fold increased risk of GH deficiency. Most children with hypopituitarism still present in the cephalic position and altered movement in utero secondary to the underlying defect in brain development may be the common link between hypopituitarism and breech delivery ( ; ).
Molecular basis
The molecular background to some cases of PHD has been established. Isolated deficiencies of GH ( ; ), TSH ( ), ACTH ( ; ) or gonadotrophins ( ; ; ; ) may reflect mutations of the respective genes. Mutations of the GH-1 gene can result in recessive and dominantly inherited isolated GH deficiency ( ; ; ; ) and defects of the GHRH receptor can also result in isolated GH deficiency ( ). Severe isolated ACTH deficiency presenting in the neonatal period may be due to mutations in TBX19 ( TPIT ) ( ).
A defect in one of the genes involved in the development of the pituitary may result in a more severe endocrinopathy with deficiencies of more than one hypothalamic and/or pituitary hormone. Defects of the gene POU1F1 ( Pit-1 ) are typically associated with a deficiency of GH, TSH and prolactin ( ; ; ; ) whilst the gene PROP1 , active at an earlier stage of pituitary gland development, is associated with deficiency of GH, TSH, prolactin, gonadotrophins ( ; ) and sometimes ACTH deficiency as well ( ; ) ( Table 34.6 ). HESX1 has a fundamental role in CNS development and homozygous and heterozygous mutations of HESX1 are a recognised cause of SOD. Heterozygous defects can also cause isolated GHD ( ; ; ). Defects of SOX3 may result in PHD in association with mental retardation whilst defects in LHX4 may result in PHD in association with abnormalities of the cerebellar tonsils.
GENE | PHENOTYPE | INHERITANCE |
---|---|---|
POU1F1 | CPHD: GH, TSH, prolactin deficiencies; usually severe; small or normal AP | Recessive, dominant |
PROP1 | CPHD: GH, TSH, LH, FSH, prolactin deficiencies; evolving ACTH deficiency; small, normal or enlarged AP | Recessive |
HESX1 | IGHD, CPHD, septo-optic dysplasia; APH, EPP, absent infundibulum, ACC | Recessive, dominant |
LHX3 | CPHD (GH, TSH, LH, FSH, prolactin deficiencies), short neck, limited rotation; small, normal or enlarged AP, short cervical spine | Recessive |
LHX4 | CPHD (GH, TSH, ACTH deficiencies); small AP, EPP, cerebellar abnormalities | Dominant |
SOX3 | IGHD and mental retardation, panhypopituitarism; APH, infundibular hypoplasia, EPP | X linked |
SOX2 | Hypogonadotrophic hypogonadism; APH, bilateral anophthalmia/microphthalmia, abnormal corpus callosum, learning difficulties, oesophageal atresia, sensorineural hearing loss | De novo |
Clinical features ( Table 34.7 )
Clinical recognition of hypopituitarism in the newborn can be difficult. GH deficiency leads to relatively subtle changes in body form and whilst some authors report a reduction in length of about 1 sd with a normal birthweight ( ) other studies indicate that birth length is largely unaffected ( ; ). GH-deficient children grow slowly in infancy and may be more than 2 sd below the mean for length by the end of the first year of life ( ). Micropenis can be a feature of GH deficiency but is more typically associated with gonadotrophin deficiency ( ). GH promotes longitudinal growth but also has lipolytic and ketogenic activity. Ketones are an important fuel in the newborn period and babies with isolated GH deficiency are therefore more susceptible to hypoglycaemia. Hypoglycaemia is typically more profound in the infant with adrenocortical insufficiency and a deficiency of one or both of these hormones should be considered in the hypoglycaemic baby. Prolonged neonatal jaundice with a conjugated hyperbilirubinaemia is a further well-recognised feature of congenital hypopituitarism ( ) and is closely linked to cortisol deficiency. All neonates with suspected PHD should have an opthalmological assessment because of the possiblility of underlying SOD.
Clinical features |
|
Investigations |
Baseline investigations |
|
Biochemistry at the time of hypoglycaemia |
|
Other |
|
Further investigations ( Table 34.7 )
Diagnosing PHD typically involves piecing together a range of clinical and biochemical parameters. The neonate with microphallus, hypoglycaemia and a low free T 4 (fT 4 ) is at high risk of having CPHD. The diagnosis is even more likely if the infant has a conjugated hyperbilirubinaemia and small optic discs on opthalmological examination. Random measurements of pituitary hormones (particularly TSH and fT 4 ) can be helpful but hypoglycaemia may not be an effective stimulus for GH and cortisol production (babies who prove to be GH- and ACTH-‘sufficient’ may have low levels at the time of hypoglycaemia). Hence stimulation tests may be required as part of the diagnostic work-up. Biochemical testing can be extremely helpful but requires careful planning and is potentially misleading because, like all tests, there are false positives and negatives. GH levels are often elevated in the first days of life and a low random GH concentration raises the possiblity of hypothalamopituitary disease.
It is important to remember the TSH surge and associated increase in thyroid hormone levels that occur in healthy babies around the time of delivery when interpreting thyroid function tests ( Fig. 34.8 ). Laboratories may not have appropriate age-related data for babies in the first days of life and so a ‘normal’ adult fT 4 may be abnormally low at this time of life. TSH levels can, paradoxically, be mildly elevated in secondary hypothyroidism and there may be an exaggerated response to TRH ( ). The increase in TSH levels and exaggerated response in hypothalamic hypothyroidism may reflect the presence of detectable but bioinactive TSH ( ) or alternatively the abnormal production of TSH in the absence of hypothalamic hormones such as somatostatin. Although the TRH test has its advocates ( ), many feel that the diagnosis of secondary hypothyroidism can be made with a single or serial measurements of TSH and thyroid hormone ( ).
Cortisol deficiency secondary to ACTH deficiency can be associated with hyponatraemia because cortisol inhibits arginine vasopressin (AVP) production and is required to excrete a water load. Potassium levels are not typically raised because mineralocorticoid production is primarily regulated by the renin–angiotensin system and not ACTH. The Synacthen test is still an appropriate test with which to investigate possible secondary hypoadrenalism (ACTH deficiency) prior to commencing hydrocortisone in the infant with hypoglycaemia and suspected hypopituitarism. The CRH test has theoretical advantages but both tests are hampered by concerns about sensitivity and specificity, a lack of reliable normative data as well as the more complex and, in the case of the CRH test, more limited availability of the ACTH assay. GnRH administration with assessment of LH and FSH levels may be helpful ( ) but the assessment of other axes is more important if there is a limited amount of blood available. It should be remembered that drugs such as dopamine may interfere with endocrine investigations ( ).
It can be difficult to balance the desire to obtain comprehensive biochemical data with the need to treat the sick, hypoglycaemic neonate. If the clinical picture strongly suggests hypopituitarism then it is wise to treat and then reassess the neonate biochemically at a later stage. Hormone deficiencies can evolve with time (see above) and a neonate who is ACTH-‘sufficient’ can become ACTH- and hence cortisol-‘insufficient’ in later childhood.
MRI can provide extremely helpful information about pituitary/hypothalamic anatomy as well as information about other CNS developmental abnormalities such as optic nerve hypoplasia or migrational abnormalities such as schizencephaly, which are more common in babies with CPHD ( Fig. 34.7 ).
Treatment
Hypopituitarism is treated by replacing the missing pituitary hormone (GH) or the product of its target organs (T 4 or hydrocortisone). Although the cortisol production rate in the newborn is 6.6–8.8 mg/m 2 /24 h ( ), the absorption and bioavailability of hydrocortisone can vary ( ). Adrenal replacement (secondary to ACTH deficiency) should be with hydrocortisone in a dose around 8–10 mg/m 2 /24 h (in three or four divided doses). There are concerns about the bioavailability of some hydrocortisone suspensions and these need to be borne in mind ( ). For thyroid replacement, T 4 in a single dose of approximately 8–10 µg/kg/24 h in the newborn is suitable initially. The objective is to obtain T 4 levels in the upper part of the normal range and the dose should be adjusted accordingly. TSH levels will be low in secondary hypothyroidism once therapy has commenced and cannot be used to guide replacement. GH replacement may be needed to control the hypoglycaemia of hypopituitarism if this proves intractable and therapy may also be warranted from an early stage if growth is to be optimised.
Resistance to the actions of anterior pituitary hormones
Endocrine disorders in which hormone action is impaired or absent owing to abnormalities of the receptor or abnormal postreceptor events are well recognised. The phenotypic features of these rare conditions may suggest hypopituitarism, but the levels of pituitary hormones are usually normal or elevated. The best-described example is insensitivity to GH (Laron syndrome), which can present with hypoglycaemia and micropenis in the newborn period ( ; ).
Abnormalitites of the TSH receptor leading to hypothyroidism (see below), the ACTH receptor leading to glucocorticoid deficiency and the LH receptor leading to genital ambiguity are considered in more detail in the appropriate sections below ( ; ; ).
Posterior pituitary ( )
Normal function
AVP, a nonapeptide, is the major determinant of renal solute-free water excretion. AVP acts to conserve body water by altering the permeability of the distal convoluted and renal collecting tubules, thereby increasing urine osmolality. AVP is produced by secretory neurons residing in the supraoptic nuclei and paraventricular nuclei from the end of the first trimester. The neurons project along the supraoptic–hypophyseal tract and terminate in the posterior pituitary. The generation and release of mature peptide from the axon terminal is the consequence of the processing and cleavage of a large precursor molecule, preproAVP, encoded by the AVP-neurophysin II gene. Cleavage of the signal peptide occurs as preproAVP translocates into the endoplasmic reticulum. Dimerisation occurs as the molecules pass into the neurosecretory granules from the Golgi apparatus. Further oligomerisation and processing occur as the neurosecretory granules move along towards the axon terminal, with cleaved neurophysin II and copeptin binding to AVP. Exocytosis and release of the mature AVP–neurophysin complex from the nerve endings in the posterior pituitary follow neurotransmitter-induced depolarisation. The AVP–neurophysin complex then dissociates to release free hormone. Secretion is primarily controlled by the osmoreceptors of the hypothalamus, although non-osmotic stimuli, notably blood volume and blood pressure, also influence vasopressin release via the stretch receptors of the left atrium and the baroreceptors in the carotid sinus. Mature AVP has a circulating half-life of 5–15 minutes.
Cranial diabetes insipidus ( )
In the absence of vasopressin, urine volume and tonicity are changed only minimally in the distal tubule and collecting ducts. Urine volume can reach nearly 10% of the glomerular filtrate, with an osmolality of 100 mmol/kgH 2 O or less. The infant with AVP deficiency (cranial diabetes insipidus or CDI) will therefore tend to pass inappropriately large amounts of dilute urine for a given plasma osmolality.
The causes of diabetes insipidus in the newborn are shown in Table 34.8 and include congenital brain malformations such as SOD and holoprosencephaly ( ). Trauma can cause diabetes insipidus, although the site of injury will determine whether or not this is permanent. Damage to the supraoptic nucleus or high stalk section causes permanent CDI, with more distal lesions usually causing transient dysfunction.
Primary |
Genetic |
|
With congenital malformations (which may also have a genetic, single-gene basis) |
|
Secondary |
|
Clinically the condition is characterised by excessive fluid output and intake. Diagnosis is difficult in the newborn because a high urine output is easily overlooked and persistent crying and weight loss can easily be attributed to a cause other than water loss. Infants may therefore present late, with non-specific symptoms such as anorexia, vomiting, poor weight gain, constipation or delayed development. Failure to thrive can occur ( ) but is less pronounced than in children with nephrogenic diabetes insipidus, because the urine tends to be less dilute. The low osmolar load associated with breastfeeding may help to maintain a normal serum osmolality and reduce symptoms.
The diagnosis is confirmed by a failure to concentrate the urine in spite of plasma hypertonicity, with reversal by administration of the vasopressin analogue desmopressin (DDAVP). Polyuria due to hypercalcaemia or potassium deficiency must be excluded, and allowance made for the lesser concentrating power of the neonatal kidney. Circulating vasopressin concentrations can be measured, although a relatively large amount of plasma is required. The principal differential diagnosis is nephrogenic diabetes insipidus, where there is resistance to the effects of circulating AVP
Treatment
Treatment is usually with the vasopressin analogue DDAVP. This compound is more potent than the native molecule and has a longer half-life ( ). It is important to be aware of the danger of water overload, which will develop if urine is inappropriately concentrated for a given fluid intake. Babies have been treated with DDAVP administered nasally but the likelihood of water intoxication is markedly reduced with oral therapy and this is the preferred route of administration ( ; ). Capillary or serum sodium osmolality should be checked regularly, particularly in the initial phase as the dose is being adjusted. It is safer in both the short and the long term for an infant with diabetes insipidus (and an intact sense of thirst) to be allowed to compensate for a relatively low dose of DDAVP by feeding or drinking more and babies should be offered water on occasions as well as milk. Parents should be actively involved in management from an early stage and encouraged to gauge fluid input and output.
A suitable starting dose of oral DDAVP is around 1–4 µg daily, increased to twice a day once the impact of the first dose has been established. The dose can be administered orally using the desmopressin injection solution (4 µg/ml), although some people have used an aliquot of a DDAVP tablet dispersed in water. If the baby is sleeping longer overnight then the logical time to administer a single daily dose or the larger dose of a twice-daily regimen is in the evening. We do not recommend the nasal route of administration but if there is no alternative then the standard solution (100 µg/ml) can be diluted with 0.9% NaCl solution to create a more manageable volume (for example, 10 µg/ml – discuss with the hospital pharmacy). This can then be instilled into the nose using a 1-ml syringe. A suitable starting dose in the newborn is 0.1–1.0 µg once or twice daily. Initially it is wise to administer the second dose only when the impact of the first has been established.
Low-dose subcutaneous DDAVP has been advocated as a safe means of managing CDI in infancy ( ). A low dose (usually 0.01 µg, s.c.) used once a day can be initiated and then increased to maintain a normal serum sodium. DDAVP (4 µg/ml) can be diluted 1 : 10 in a syringe with the required dose ranging from 0.02 to 0.08 µg DDAVP s.c. twice daily.
Finally, 5 mg/kg of chlorothiazide administered twice a day has also been used to treat CDI on the basis that, as with nephrogenic diabetes insipidus, the associated increase in urine osmolality will compensate for vasopressin deficiency ( ).
Excess vasopressin secretion (syndrome of inappropriate antidiuretic hormone secretion)
This is described in Chapter 18 .
The hormonal regulation of appetite and feeding behaviour
An increasing number of hormones and receptors are known to be involved in the regulation of feeding behaviour and growth. The hormone leptin is produced by the ob gene in the adipocyte and circulating levels provide the hypothalamus with information about nutritional status. This in turn will influence feeding behaviour, as vividly illustrated by rare examples of leptin deficiency or resistance ( ; ). Leptin levels are a reflection of fat mass and cord leptin levels are positively related to birthweight ( ; ). Feeding behaviour will influence growth, and leptin status has been shown to be related to weight gain in the first months of life ( ).
As many as 5% of children with severe early-onset obesity may have defects of the MC4 receptor ( ) and patients with rare abnormalities of the POMC gene can present with ACTH deficiency and demonstrate obesity with associated rapid growth in early life ( ).
Other components of the appetite-regulatory pathway are steadily being elucidated and the gut peptide PYY has been shown to inhibit food intake ( ) whilst ghrelin ( ), a GH secretogogue produced by a number of tissues including the gastrointestinal tract and the hypothalamus, promotes feeding. Reduced insulin levels between feeds are associated with a rise in ghrelin release and GH production. Hence there is a complex interplay between feeding, fasting, hormone production and the regulation of intermediate metabolism and fuel supply in the newborn period ( ; ; ).
Thyroid
Disorders of thyroid gland development and/or function are relatively common, affecting approximately one newborn infant in 3000. Recent advances in this field reflect the development of more refined biochemical assays, the elucidation of genes involved in thyroid gland development and hormone synthesis, as well as information gained from screening programmes for congenital hypothyroidism (CHT).
Normal function
Thyroid function in utero
TSH, modulated by TRH, stimulates the synthesis and release of T 4 and triiodothyronine (T 3 ) from the thyroid into the plasma, where they circulate, strongly bound to proteins. Maternal T 4 , but not TSH or T 3 , can cross the placenta in physiologically significant amounts and this explains the relatively normal phenotype in infants with CHT at birth with levels that are one-third to one-half normal values in babies unable to manufacture thyroid hormone ( ). The importance of maternal T 4 delivery to the fetus in early gestation, whilst the fetal thyroid axis is developing, is supported by studies of neurodevelopment in infants of mothers with relatively low T 4 levels ( ; ). The significance of T 4 transfer in the latter stages of pregnancy is illustrated by the life-threatening illness seen in hypothyroid infants of hypothyroid mothers ( ; ).
The greater part of circulating T 3 is derived from peripheral monodeiodination of the outer ring of T 4 , which therefore acts as a reservoir or prohormone for the more active T 3 . An alternative monodeiodination affects the inner ring of T 4 and produces inactive reverse T 3 (rT 3 ). This mechanism permits a balance between production of the most and least active thyroid hormones. There are three deiodinases – type I (D1), which has inner and outer deiodination activity; type II deiodinase (D2), which catalyses outer-ring deiodination; and type III deiodinase (D3), which catalyses inner-ring deiodination.
Fetal thyroid hormone metabolism is characterised by a predominance of D3 activity outside the CNS in tissues such as the liver, kidney and placenta. This converts maternal and endogenous T 4 preferentially to rT 3 and presumably helps to reduce tissue thermogenesis and enhance tissue anabolism. T 4 (rather than T 3 ) is required by the developing brain, and the appropriate deiodinases (particularly D2) are expressed in a temporal and spatial manner in different brain regions. Sulphotransferase enzymes also have an important role in thyroid hormone metabolism. T 4 sulphation blocks outer-ring deiodination to T 3 whilst promoting inner-ring conversion to inactive rT 3 . The activity of sulphotransferase enzymes in tissues like the liver will also, therefore, play a key role in determining thyroid hormone availability ( ). A key determinant of thyroid hormone transport into cells has been identified with defects of this protein resulting in a phenotype that includes profound neurodevelopmental delay ( ).
Thyroid function in the neonatal period
After birth there is an acute discharge of TSH which reaches a peak at 30 minutes, before falling towards basal levels within the first 3 days. There is an associated release of thyroid hormones and enhanced peripheral conversion of T 4 (closely linked to D2 activity), which results in a pronounced increase in T 3 in the first hours of life. There is a further increase in total T 3 and free T 3 levels for about 36 hours around the time of the postnatal peak in T 4 ( Fig. 34.8 ) and fT 4 levels remain relatively high for the first weeks of life ( ). In preterm infants the levels of T 3 remain higher than the cord values of babies of equivalent gestational age for several weeks ( ). Preterm infants (down to 30 weeks) show similar but lesser changes in TSH and thyroid hormone concentrations, although the most preterm babies (23–27 weeks) have relatively low T 4 values ( ). By 1–2 months of age, thyroid hormone levels are comparable to those in term infants. In the case of infants under 30 weeks’ gestation, the postnatal surge does not occur ( ) and T 4 levels frequently fall to a nadir around 1–2 weeks of age, which is more pronounced with increasing prematurity ( ; ). T 4 levels remain below those of full-term infants through the first few weeks of life ( ) and climb gradually to normal postnatal levels.

Congenital hypothyroidism
This is a heterogeneous group of patients, some of whom will have functioning thyroid tissue and some of whom will not. In those with functioning tissue, the gland may be normally sited and of normal size, hypoplastic or ectopic. Despite significant developments in our understanding of the aetiology of CHT, the cause is still unknown in most patients. Malformations besides defects of thyroid gland development and function are more common in infants with CHT ( ). It is helpful to divide patients into those with thyroid dysgenesis and those with an abnormality of one of the enzymes or factors involved in thyroid hormone synthesis (dyshormonogenesis).
Thyroid dysgenesis
Embyrological defects resulting in thyroid dysgenesis are the most common cause of CHT, and range from thyroid agenesis (no gland present) to an ectopic and/or hypoplastic gland. Some thyroid tissue is demonstrable in approximately two-thirds of affected infants, and there is a spectrum of biochemical severity. The frequency is around 1 in 3000–4000 and is twice as common in females as in males, with only minor racial and seasonal differences in incidence described. As genetic factors involved in thyroid development and thyroid-specific gene expression have been described, so abnormalities of these same genes have been identified in a small number of patients with thyroid dysgenesis. Mutations in the TSH receptor (TSHR) ( ; ) and transcription factors such as TTF1 (NKX2-1), TTF2 and Pax-8 have been published, but a strict mendelian inheritance pattern is relatively uncommon ( ; ) and the aetiology of thyroid dysgenesis in most patients is unknown ( ).
Thyroid hormone replacement abolishes the physical manifestations of hypothyroidism but intellectual and neurological prognosis is compromised in those babies at the more severe end of the spectrum unless treatment is started within the first few weeks of life ( ).
Dyshormonogenesis ( Fig. 34.9 )
Recessively inherited biochemical defects of iodothyronine synthesis are the second most common cause of permanent CHT, with a frequency in Europe and North America of approximately 1 in 30 000–50 000, accounting for some 10–15% of patients identified by screening. The sex incidence is equal. A goitre may be present at birth but, unless large, may be difficult to detect and may not develop until later in life. The degree of hypothyroidism is variable with some patients clinically and even biochemically euthyroid. Dyshormonogenesis can be a cause of transient hypothyroidism when the extra demands in early life cannot be met. Thyroid ultrasonography usually shows normal thyroid anatomy and site (a eutopic gland). In many dyshormonogeneses there is excessive discharge of iodide from the thyroid gland after administration of perchlorate (the perchlorate discharge test), due to an abnormality of iodide organification into thyroglobulin. Recognised abnormalities of thyroid hormone synthesis include defects in iodide transport across the basolateral membrane (sodium iodide symporter) ( ; ) and then into the colloid lumen ( ; ). Other causes include abnormalities of the thyroglobulin gene ( ), thyroid peroxidase ( ; ) and THOX ( ), which is involved in the generation of H 2 O 2 required by thyroid peroxidase to oxidise iodide. Delineation of the precise biochemical defect in these disorders does not alter the need for T 4 replacement, but can help when counselling families about the likelihood of recurrence.

Neonatal screening for congenital hypothyroidism ( ; ; )
The development of radioimmunoassays for measurement of T 4 and TSH in very small samples of serum or whole blood spotted on to filter paper resulted in the rapid adoption of screening in many countries. Most programmes used a single blood sample taken between the fifth and 10th days of life, by which time relative stability has returned to the thyroid axis after the abrupt postnatal perturbation. T 4 screening alone proved inadequate because of the overlap between normal and hypothyroid values. The alternatives were to add a TSH assay in all cases or for the lower range of T 4 values, or to use TSH as the primary test and accept that the rare cases of secondary hypothyroidism (hypopituitary hypothyroidism) may be missed. Many European countries have adopted the primary TSH approach whilst a combined approach with the simultaneous measurement of both TSH and T 4 is used in some parts of the USA ( ). A comparison of the three principal strategies is shown in Table 34.9 .
DISORDER | INCIDENCE IN NEWBORNS | PRIMARY TSH | PRIMARY T 4 , FOLLOW-UP TSH | COMBINED T 4 AND TSH |
---|---|---|---|---|
Primary CHT | ~1 in 2500–4000 | Good | Good | Very good |
Hypopituitary hypothyroidism (most will have combined pituitary hormone deficiency) | ~1 : 16 000 | No | Some | Some |
Transient hypothyroidism and hyperthyrotropinaemia | ~1 in 30 000 | Yes, but may require second specimen in preterms | Some | Yes |
Recall rate | 0.3–1% (cut-off-dependent) | 1–2% | ~2% |
The returns from screening proved even greater than had been expected: in both the USA and Europe, the frequency of CHT was approximately 1 in 3500, almost double that suggested by retrospective surveys ( ). The early manifestations of CHT are non-specific and development of the typical features usually occurs slowly ( Table 34.10 ). Even in the most medically advanced countries, no more than half the affected infants could be diagnosed clinically by the age of 3 months ( ). With screening, treatment could be started by 4 weeks and ideally by 2 weeks of age ( ; ).
At birth |
|
Early signs |
|
Later signs |
|
One of the key issues in countries using bloodspot TSH as a screening tool centres on the question of the TSH screening cut-off. TSH assays have become more refined and there has been a temptation to use an increasingly lower cut-off value. Reducing the screening cut-off and collecting samples relatively early (within the first 2 days of life) will increase sensitivity but reduce specificity. Whilst it is possible that true CHT is becoming more common ( ), reports of a rising incidence will, in some instances, reflect changes in screening practice. A lower TSH cut-off and repeat testing will detect subtle fluctuations in TSH or T 4 that may occur in the absence of classical thyroid dysgenesis or dyshormonogenesis (see the sections on a delayed TSH rise, transient hypothyroidism and hyperthyrotropinaemia below). The incidence of CHT in countries using the combined TSH/T 4 approach is around 1 in 2500. Dropping the TSH screening cut-off in a region of Italy using a primary TSH strategy resulted in the incidence rising from around 1 in 2600 to around 1 in 1400 ( ). Not surprisingly, the proportion of babies with established defects of thyroid gland development tends to fall as the reported incidence of CHT increases. Only longer term surveillance will establish which infants have permanent CHT. A key question is the extent to which babies with the more subtle biochemical abnormalities benefit from intervention with T 4 .
Diagnosis
The diagnosis of CHT is confirmed by finding low serum thyroid hormone concentrations (fT 4 ) and a high TSH. The fT 3 level is variable and is preserved in the normal range for longer. It remains essential to exclude hypothyroidism in any infant in whom there is clinical suspicion, because, first, errors inevitably occur in all screening programmes; second, mild hypothyroidism may escape detection by all screening methods (see below); and third, there may be a late rise in TSH levels. The major clinical features of CHT are summarised in Table 34.10 . Infants with low T 4 values are more likely to have clinical signs such as feeding difficulties, lethargy, prolonged jaundice, umbilical hernia and macroglossia ( ). Such infants are more likely to have an aplastic rather than a hypoplastic or ectopic gland. A gestation over 40 weeks, induction of labour and a birthweight above 3500 g are all more common among infants with hypothyroidism.
Further investigation
The laboratory tests of thyroid function primarily determine whether an infant requires T 4 treatment. Isotope scanning (technetium or 123 I) can be undertaken before or within a few days of starting T 4 replacement treatment, ideally when the TSH levels are still high ( ). Thyroid ultrasonography is also performed in some centres ( ) and, whilst it is a highly operator-dependent technique, it can provide information about gland morphology and hence more detail than isotope scanning alone. An ultrasound can be conducted on the neonatal unit and may be of particular value in the infant exposed to iodine-containing compounds whose isotope scan may be hard to interpret.
In thyroid dysgenesis, an isotope scan or ultrasound will reveal an absent, small or ectopic gland. This condition has a low risk of recurrence in further children ( ). The infant with thyroid aplasia or ectopia will need treatment for life and is unlikely to benefit from a trial off therapy in later childhood. If the gland is anatomically normal and the isotope uptake is high, then hypothyroidism is probably due to a dyshormonogenesis and may be inherited in an autosomal recessive (AR) manner. Absent isotope uptake in a normally sited gland on ultrasonography also suggests a dyshormonogenesis with abnormal iodine trapping.
Advocates of investigations in CHT highlight the fact that this information can be used to refine the T 4 dose ( ) and can provide information about recurrence risk and prognosis. On the other hand investigations can be operator-dependent, difficult to interpret and may involve intravenous access and ionising radiation. Some clinicians still prefer to rely on the biochemistry (TSH and thyroid hormone levels) alone. A plain radiograph of the knee to assess epiphyseal maturity was frequently performed in the past. This may reflect the degree of fetal hypothyroidism but is of limited clinical value.
A TRH stimulation test is rarely indicated, but has been used in babies with mild thyroid dysfunction. An exaggerated and prolonged TSH response is observed if TSH levels are mildly elevated because of a compromised thyroid gland ( ). TRH testing has been advocated as a way of teasing out central hypothyroidism in babies found to have low T 4 levels on screening ( ).
Treatment
T 4 is used for replacement and there is no advantage in giving T 3 (see above). It is a matter of some urgency to start treatment, and when the diagnosis is reasonably certain it may be preferable to collect further specimens and start treatment without waiting for the results.
The aim of therapy is to normalise T 4 and TSH concentrations quickly, and an appropriate starting dose of T 4 in the newborn with low T 4 levels is 10–12 µg/kg/24 h given as a single daily dose ( ; ; ). Full-term infants of normal birthweight should be commenced on 37.5–50 µg daily. This will usually bring the TSH into the normal range quickly and the dose of T 4 may then need to be reduced. Babies with significant endogenous thyroid hormone production may normalise TSH values on a smaller dose. The smallest tablet available contains 25 µg T 4 but as the hormone has a long half-life (in excess of a week) the dose can be adjusted in smaller increments by (for example) giving a higher or lower dose on alternate days as well as by dividing them. T 4 solutions are available, although there have been concerns about the stability of some of these preparations and parents need to be aware that they may come in different strengths.
Relatively high fT 4 levels may be seen after treatment is commenced but this picture does not necessarily indicate overtreatment if TSH levels are normal or raised. The dose of T 4 will fall with time when calculated according to weight and a suppressed TSH indicates overtreatment. The heterogeneity of CHT has been highlighted earlier and babies with only mild or moderately elevated baseline TSH levels may require smaller doses of T 4 than babies with agenesis, in whom the baseline TSH is typically very high ( ). Replacement is usually satisfactory if the serum total T 4 and fT 4 are in the upper part of the quoted laboratory normal range for age, but suboptimal compliance needs to be excluded if TSH values remain elevated despite a substantial T 4 dose.
There is evidence to suggest that higher initial starting doses of T 4 may have a beneficial impact on neurodevelopment ( ; ; ), although there has been concern that this might be at the expense of an increase in behavioural problems ( ; ). Severe overdosage may also cause accelerated growth and even craniosynostosis, but the danger of impaired neurological development from underdosage is probably greater.
Higher doses of T 4 treatment in early life as well as a milder baseline biochemical picture have been associated with increased stature in mid-childhood and at final height ( ; ; ), although this has not been a consistent observation ( ).
Follow-up and prognosis
Growth, clinical progress and thyroid function should be checked after 2 and then 4 weeks on treatment, and then at 1–2-monthly intervals in the first 6 months and then every 3–4 months up to 3 years of age. Definitive reassessment of thyroid status can be undertaken after the age of 2 years, when brief cessation of treatment will have no adverse effects. Most children with a permanent defect of thyroid hormone generation will develop abnormal biochemistry without any adverse symptoms if T 4 is stopped for 2–4 weeks at this stage. In addition to a biochemical assessment of thyroid function, the opportunity can be taken to obtain or repeat an isotope thyroid scan. If the T 4 dose has been increased during the first 2 years of life because of an elevated TSH then this may not be necessary.
CHT is associated with impaired motor development, a reduced intelligence quotient (IQ) and impaired hearing and language problems ( ; ; ). These features are related to the severity of hypothyroidism at birth ( ). In one study, total T 4 levels below 42 nmol/l in the neonatal period were associated with a 10-point deficit in IQ at school entry ( ). Individuals with T 4 values above 42 nmol/l were no different from controls, suggesting a ‘threshold’ effect. Thus it seems that the degree of pre- and perinatal hypothyroidism has implications for CNS function in the long term. A formal hearing assessment is recommended in the hypothyroid infant because of the 10-fold increase in hearing loss in these babies ( ).
Neonatal screening and the preterm infant: the delayed TSH rise
A well-recognised phenomenon is that of the infant in whom the initial neonatal screening TSH is normal but who then develops an elevated TSH in the context of low thyroid hormone levels in the subsequent few weeks. Many such cases are infants born preterm or of low birthweight. referred to this as ‘atypical hypothyroidism’ and this pattern is the principal reason why some countries opt for a second bloodspot screen in all babies or in those born preterm. It is likely that many of the preterm infants will have transient abnormalities of thyroid function related to illness, exposure to drugs such as dopamine, a negative iodine balance or exposure to topical iodine-containing compounds. Many paediatricians feel that abnormalities of thyroid gland development per se are not more common in preterm infants but that some preterm infants with CHT, in contrast to term infants, may not generate increased levels of TSH because of ‘immaturity’ of the hypothalamopituitary–thyroid axis. Lowering the initial TSH screening threshold may identify ‘at-risk’ infants and reduce the need for a second dried bloodspot sample ( ).
Other abnormalities of thyroid function in the neonatal period
Transient hypothyroidism and hyperthyrotropinaemia (subclinical hypothyroidism)
In this heterogeneous group of disorders, the TSH is raised and thyroid hormone concentrations are within the age-related reference range (hyperthyrotropinaemia) or temporarily low (hypothyroidism). Babies may have had a mild abnormality of thyroid function detected by the screening programme or may have had thyroid function checked for other reasons. Although thyroid function may normalise within the first few months of life, some of these infants may have a permanent, albeit subtle, abnormality of thyroid function ( ; ). There are well-established causes of transient thyroid dysfunction but some of these children will lie at the mild end of the CHT phenotypic spectrum. The overlap with CHT is illustrated by the transient hyperthyrotropinaemia that can occur in infants with an underlying dyshormonogenesis ( ). These babies are presumably unable to meet the demand for T 4 in the early neonatal period. Some babies with hyperthyrotropinaemia will have abnormal thyroid gland morphology ( ).
A list of causes of hyperthyrotropinaemia/transient hypothyroidism is given in Table 34.11 . Noteworthy causes of transient hypothyroidism include intrauterine exposure to antithyroid drugs ( ), iodine deficiency ( ; ) and exposure to topical iodinated antiseptic agents. The last are readily absorbed and should be avoided or carefully removed following initial application ( ; ; ). Transient hypothyroidism may also be due to the transplacental passage of thyrotropin receptor-blocking antibodies in approximately 2% of cases of CHT ( ) and it is wise to check a mother’s thyroid status in these circumstances. Causes of permanent hyperthyrotropinaemia include abnormalities of thyroid gland development ( ) and abnormalities of the TSH receptor ( ). Abnormal thyroid imaging is, not surprisingly, more common in babies with permanent hyperthyrotropinaemia ( ). Transient hypothyroidism and hyperthyrotropinaemia appear to be more common in the preterm infant with some infants demonstrating the delayed TSH rise described above. Hence iodine deficiency or exposure to excess iodine could result in a ‘delayed’ TSH rise as well as transient hypothyroidism and hyperthyrotropinaemia.
|
Intervention is important in those patients with low thyroid hormone levels and some clinicians may treat relatively subtle increases in TSH that persist on the basis that this is a marker of thyroid hormone insufficiency within the developing CNS ( ; ). Intervention is of unproven benefit but likely to be safe provided the infant is monitored appropriately and TSH suppression avoided.
Transient hypothyroxinaemia ( )
All premature infants have some degree of hypothyroxinaemia because cord serum T 4 values increase with gestational age. Low T 4 levels in the preterm infant reflect the loss of the normal maternal T 4 supply, immaturity of the hypothalamopituitary–thyroid axis, as well as other factors such as the ongoing fetal tendency to convert thyroid hormone to inactive rT 3 . Low thyroid hormone values persist in the first 1–2 weeks in association with low fT 4 and normal TSH levels. This biochemical picture tends to be more profound in the most preterm babies ( ). This was thought to be of little consequence, but more recent evidence indicates that there is a relationship between T 4 concentrations in preterm and low-birthweight (LBW) infants and subsequent neurodevelopmental outcome ( ; ; ; ).
The impact of thyroid hormone administration on the short- and longer-term outcome following preterm delivery has yet to be established. Improvements in survival have been reported in some studies ( ) but not others ( ) and early neurodevelopmental outcome does not appear to be affected by treatment. A subgroup analysis in the study by ) suggested a potential benefit of T 4 treatment (8 µg/kg/day) on the Bayley Mental Development Index in babies of 25–26 weeks’ gestation. However, evidence from the same and other studies has suggested that increasing T 4 delivery in babies born at 27–29 weeks may have an adverse effect on neurodevelopment. A more recent report from the same group, as the children reach school age, has confirmed the improved IQ with a reduction in behavioural problems in those receiving T 4 at less than 27 weeks, and lower IQ with more developmental problems in those receiving T 4 at 29 weeks ( ). The effects of T 4 administration may therefore depend on the gestation and age of the infant treated, with protective mechanisms ensuring an adequate supply of T 3 in all but the most preterm. At this time, the routine supplementation of babies of any gestation in whom TSH levels are normal is not recommended, but we do recommend following the thyroid hormone levels until they normalise.
It is important to remember that preterm infants with hypothyroxinaemia may have a permanent abnormality of thyroid function which will only become apparent with longer term follow-up ( ).
Secondary (hypopituitary) hypothyroidism
Secondary hypothyroidism (TSH deficiency) should be suspected if fT 4 and T 3 levels are low in the presence of a low, normal or paradoxically mildly elevated TSH. The differential diagnosis of this biochemical picture includes euthyroid illness and prematurity (see below). Isolated TSH deficiency is very rare and most infants with secondary hypothyroidism will have other PHDs with associated clinical and biochemical features. Screening programmes using the combination of TSH and T 4 suggest that the incidence of secondary hypothyroidism is around 1 in 16 000. The TSH response to TRH in patients with secondary hypothyroidism may be normal, poor or even exaggerated ( ).
The ‘low T 3 syndrome’ (non-thyroidal illness)
This term is used to describe thyroid function tests characterised by low serum T 3 concentrations, normal or raised rT 3 , variable T 4 and normal TSH. Fetal T 3 levels are low throughout gestation because of enhanced conversion of T 4 to rT 3 , and this picture is frequently observed in preterm infants. As in older patients, T 3 levels may be further reduced by intercurrent illness and by poor nutrition in infants of all gestational ages. Low T 3 levels, like T 4 , have been linked to a reduction in IQ in later life ( ). T 4 administration to infants of less than 30 weeks’ gestation does not increase T 3 levels ( ).
Peripheral abnormalities of thyroid hormone binding, transport or action
Abnormalities of binding proteins: thyroxine-binding globulin deficiency and familial dysalbuminaemic hyperthyroxinaemia
The major carrier protein of the thyroid hormones is thyroxine-binding globulin (TBG). Deficiency of TBG is usually inherited as an X-linked dominant trait and has an incidence in male infants of 1 : 2400 with an overall frequency around 1:4000–4300 in North America ( ; ). Affected patients are euthyroid. Total T 4 and T 3 levels are low, but the free fractions are normal and the resting and stimulated TSH values are also normal. TBG measurement is needed for a definitive diagnosis.
Familial dysalbuminaemic hyperthyroxinaemia is a relatively common disorder, affecting 1 in 100 people. It is characterised by increased T 4 levels in clinically euthyroid individuals because of an abnormal albumin molecule that has an increased affinity for thyroid hormone. The abnormal albumin molecule will also interfere with some thyroid hormone assays, leading to spurious and potentially confusing results ( ). This condition underlines the importance of measuring TSH concentrations in infants with raised thyroid hormone concentrations.
Abnormal thyroid hormone transport into the cell
Thyroid hormone transport across the cell membrane is an active process that involves the membrane protein monocarboxylase transporter 8 (MCT8). MCT8 action is crucial if thyroid hormone is to enter the cell and then exert its biological effects by binding to nuclear thyroid receptors. Mutations in MCT8, located on the X chromosome, result in severe psychomotor retardation with a biochemical picture that classically involves elevated T 3 levels and a normal or mildly elevated TSH. Hemizygous males with MCT8 mutations are severely affected whilst heterozygous females with one abnormal and one normal allele have a normal phenotype in the presence of a milder biochemical abnormality ( ).
Thyroid hormone resistance ( ; )
Resistance to thyroid hormone is caused by mutations in the thyroid hormone receptor (TR beta) gene. The clinical presentation of resistance to thyroid hormone is highly variable and the majority of individuals are completely asymptomatic. Occasionally neonates will be found to have this disorder because of a goitre or because of a raised TSH. A minority may have symptoms suggestive of hypothyroidism such as growth retardation and impaired cognitive ability, whilst others have signs of thyroid hormone excess such as tachycardia or hyperactivity ( ). The typical picture is of a healthy, clinically euthyroid infant with a raised TSH and raised fT 4 and fT 3 . The disorder is usually inherited in an autosomal dominant (AD) manner and so a parent may have similar biochemistry.
Hyperthyroidism
Neonatal thyrotoxicosis
Neonatal thyrotoxicosis is a rare but potentially serious condition, usually caused by the transplacental passage of TSH receptor-stimulating antibodies from the serum of a mother with active, inactive or treated Graves disease ( ). It may also occur when the mother has autoimmune thyroid disease other than Graves ( ). The disease is usually transient, resolving within the timespan of the circulating antibodies. Neonatal hyperthyroidism is underrecognised but it can often be predicted and treated prenatally.
TSH receptor-stimulating antibodies may be demonstrated in the majority of patients with Graves disease and they cross the placenta freely. Thyrotoxicosis in the fetus may lead to preterm labour, LBW, stillbirth and neonatal death. Approximately 1% of babies of mothers with Graves disease become overtly thyrotoxic, although the absolute risk is related to the concentration of TSH receptor-stimulating antibodies in the maternal serum ( ; ; ) and some babies will be biochemically toxic but asymptomatic. Thyroid dysfunction has been detected in as many as 16.5% of babies born to women with Graves disease ( ). TSH receptor-blocking antibodies may be present as well, and the clinical and biochemical picture will reflect a range of factors, including the impact of altered maternal and fetal thyroid hormone levels on fetal hypothalamopituitary function as well as the nature and concentration of prevailing antibody concentrations. Exposure to antithyroid drug treatment in utero will also influence the neonatal picture. Hence, an infant who is initially hyperthyroid can subsequently become hypothyroid (requiring T 4 replacement) and an initially hypothyroid infant can become hyperthyroid ( ; ; ).
Although neonatal thyrotoxicosis is usually due to the transplacental passage of TSH receptor-stimulating antibodies, activating mutations of the TSH receptor can also give rise to a similar picture. This form of hyperthyroidism is typically inherited in an AD fashion (a parent may have a history of thyroidectomy) and will not resolve spontaneously. Treatment is with total thyroidectomy ( ; ).
Clinical features ( Table 34.12 )
The signs of thyrotoxicosis in the fetus include tachycardia and intrauterine growth retardation. Infants with perinatal thyrotoxicosis may show signs of hyperthyroidism immediately after birth, but symptoms may be delayed as long as 4–7 weeks ( ; ). This may be due to the effect of maternal antithyroid drugs or to the relative effects of both blocking and stimulating antibodies. Infants may have a palpable goitre, and although eye signs, especially proptosis and lid retraction, can be present at birth, they are often mild or absent throughout the course of the disease. Rarely, a mother with euthyroid ophthalmic Graves disease may produce an infant with eye involvement but no evidence of thyrotoxicosis. Signs of CNS stimulation, such as irritability, restlessness and jitteriness, usually predominate and there is tachycardia and occasionally arrhythmia, which may progress rapidly to severe and intractable heart failure. Other signs of hypermetabolism include an excessive appetite with weight loss or inadequate weight gain, diarrhoea, sweating and flushing. Less predictable clinical features include hepatosplenomegaly, jaundice and accelerated bone maturation, which can cause premature closure of the skull sutures. Mortality rates of 16–25% have been reported and the long-term outcome is uncertain ( ).
Thyroid | Goitre |
Central nervous system | Irritability, restlessness, jitteriness, microcephaly |
Eyes | Stare, lid retraction, periorbital oedema, exophthalmos |
Cardiovascular system | Tachycardia, cardiac failure, arrhythmia, hypertension |
Gastrointestinal tract | Excessive appetite, weight loss, failure to thrive, diarrhoea, hepatosplenomegaly |
Other | Sweating, flushing, acrocyanosis, lymphadenopathy, thymic enlargement, thrombocytopenia, bruising, petechiae, hyperviscosity, advanced skeletal maturation, craniosynostosis, microcephaly |
Management ( Fig. 34.10 )
Hyperthyroid pregnant women should be treated with the antithyroid thiourea derivatives carbimazole or propylthiouracil ( ). The use of radioactive iodine is absolutely contraindicated during pregnancy ( ) and surgery may precipitate preterm delivery. The antithyroid drugs cross the placenta, and the lowest dose that controls the hyperthyroidism should be used. Propylthiouracil is excreted into breast milk in lower concentrations than carbimazole and hence may be preferable. The ‘block and replace’ regimen, using higher doses of antithyroid drugs in combination with replacement T 4 , should be avoided. If maternal antithyroid treatment causes goitre formation and bradycardia in the fetus, it is possible to give T 4 by intra-amniotic injection. There may be biochemical evidence of transient hypothyroidism in clinically euthyroid babies of mothers treated with antithyroid drugs ( ). If fetal tachycardia suggests hyperthyroidism, treatment should be adjusted to maintain the fetal heart rate below 160 beats/min and careful assessment of fetal growth is necessary. Cordocentesis may be used to confirm the diagnosis.

Severe hyperthyroidism carries a high mortality in the newborn. The key to successful management is, first, anticipation and prevention, then control of thyroid status until the disease runs its self-limited course. If the fetus was hyperthyroid and the mother received a thionamide during pregnancy, then the wisest course will usually be to continue the thionamide medicine (CBZ is preferred in children: ) in a suitable neonatal dosage, which is carbimazole 0.5–1.5 mg/kg/24 h in divided doses every 8 hours. Regular assessment of thyroid function is necessary. Babies who are clinically euthyroid but subsequently show signs of hyperthyroidism will also need to be treated. Neonatal thyrotoxicosis usually remits after 2–5 months and so antithyroid medication can be withdrawn around this time.
Administration of a generous dose of antithyroid drug and simultaneous replacement with T 4 (‘block and replace’) has been used in thyrotoxic infants but the relatively short duration of the hyperthyroid phase makes this approach impractical in most babies. If a baby is thought to be at particular risk of hyperthyroidism, if a sibling was symptomatic or if there is a high titre of maternal antibodies, then close clinical and biochemical surveillance should be continued for the first weeks of life. Treatment with an antithyroid preparation should be started promptly if necessary.
If acute thyrotoxicosis does occur, then in addition to a thionamide, propranolol 2.0 mg/kg/24 h in divided doses by mouth 6–8-hourly can be used to control the peripheral stimulatory effects of thyroid hormones and/or potassium iodide, as aqueous iodine solution (5% potassium iodide, 130 mg/ml) 0.05–0.1 ml 8-hourly can be administered to prevent synthesis and release of thyroid hormones from the gland. Radiographic iodine-containing agents such as sodium ipodate (0.5 mg every 3 days) have also been used in the treatment of neonatal Graves disease ( ). Severely ill babies can be treated with sedatives as well as a glucocorticoid such as prednisolone (2 mg/kg/day) which suppresses T 4 to T 3 conversion.
Occasionally an infant born to a mother with Graves disease will be found to have a hypothyroid picture with low thyroid hormone levels and a raised or suppressed TSH. In some instances, a low TSH and low T 4 reflect suppression of the hypothalamopituitary–thyroid axis by the transplacental passage of thyroid hormone from a hyperthyroid mother, and these babies will need T 4 replacement whilst the axis recovers ( ; ; ). The transplacental passage of blocking antibodies and exposure to antithyroid drug may account for low thyroid hormone levels.
Disorders of calcium metabolism
Normal physiology
Calcium is the most plentiful mineral in the body and has a central role in many physiological processes including haemostasis, hormone secretion and action, enzyme activation and inhibition, transmission across cellular membranes and muscle contraction. Calcium is also key to the formation of mineralised connective tissue that provides the skeleton with its structural integrity.
Of the total body stores of calcium, approximately 99% is found in bone and can be considered metabolically inactive as it is mobilised from this pool only slowly. Of the remaining 1%, around half (0.5% of the body total) is the ‘biologically active’ ionised form. A further 40% is bound to circulating proteins albumin (80%) and globulin (20%), although the exact proportion varies with the serum concentration of albumin and pH. The remainder exists as diffusible non-ionised calcium present in complexes with phosphate and citrate ( ). Because hydrogen ions compete with calcium for protein-binding sites, an increase in acidity will result in a release of calcium from albumin and increase the ionised fraction of total body calcium. An alkalosis will result in the opposite effect.
The maintenance of serum calcium levels in the physiological range is a complex process that primarily reflects the function of, and interaction between, parathyroid hormone (PTH), vitamin D and the calcium-sensing receptor (CaSR) ( Fig. 34.11 ).

The calcium-sensing receptor
The extracellular CaSR is one of a group of G protein-coupled cell surface receptors. The CaSR modulates the production and secretion of PTH by the chief cells in the parathyroid gland ( ). The CaSR has been termed the ‘calciostat’ ( ) because of its fundamental role in maintaining the narrow normal range ( ) of ionised calcium in blood. Rising calcium levels inhibit chief cell activity whilst falling levels of serum calcium will result in a rise in CaSR-induced cellular function. The CaSR is also known to be expressed in other tissues involved in calcium homeostasis, such as bone, gut and kidneys.
Gene defects that result in impaired or enhanced receptor activity can alter the ‘set-point’ and lead to hypo- or hypercalcaemia. For instance, AD familial benign hypocalciuric hypercalcaemia (impaired receptor function) and hypercalciuric hypocalcaemia (enhanced receptor function) may present in the neonatal period. These rare but important causes of neonatal hyper- and hypocalcaemia are described in more detail below.
Parathyroid hormone
PTH, a polypeptide with 84 amino acid residues, is secreted by the four (occasionally up to seven) parathyroid glands that usually lie behind the thyroid gland. The 34 amino-terminal residue has full biological activity and the function of the remainder of the polypeptide is unknown . Reduced calcium binding on the extracellular side of the CaSR results in an increase in PTH release via exocytosis from the chief cell of the parathyroid gland ( ). An increase in circulating phosphate will have a similar effect as it will complex with free ionised calcium to form calcium phosphate which is not detected by the CaSR. Low magnesium levels inhibit PTH secretion and so serum levels of this mineral should be measured whenever hypocalcaemia is being investigated.
When PTH is released, it is rapidly degraded into a number of fragments, the more active of which include the amino-terminal sequence. The half-life of PTH is a few minutes but some inactive fragments remain in the circulation longer.
PTH has three key actions:
- 1
osteoclast stimulation with an associated release of calcium from bone
- 2
to promote renal phosphate excretion (and sodium, potassium and bicarbonate) and decrease renal tubular calcium reabsorption (and magnesium and hydrogen ions)
- 3
the stimulation of 1-α hydroxylase enzyme activity in the proximal renal tubule, thereby increasing the formation of 1,25-dihydroxyvitamin D. This will, in turn, promote intestinal calcium absorption.
The first two actions will regulate calcium levels acutely whilst the third has a more delayed onset of action.
Vitamin D
The two naturally occurring forms of vitamin D are ergocalciferol (D 2 ), which is derived from ingested vegetables, and cholecalciferol (D 3 ), which is formed from 7-dehydrocholesterol by the effects of ultraviolet light on the skin. Vitamin D 3 is also absorbed from animal sources in the diet by the upper part of the small intestine. The formed cholecalciferol and absorbed ergo-/cholecalciferol are hydroxylated, primarily by the liver, to 25-hydroxyvitamin D, which is the major circulating vitamin D metabolite. This undergoes 1-hydroxylation in the kidney to 1,25-dihydroxyvitamin D, the metabolically active form, or to the less active 1,24,25-trihydroxyvitamin D or 24,25-dihydroxyvitamin D. 1-hydroxylation is stimulated and 24-hydroxylation inhibited by hypocalcaemia, hypophosphataemia and PTH. Only 1–3% of circulating vitamin D is free, with most bound to vitamin D-binding protein, an α 2 -globulin.
The major effect of vitamin D is to increase serum levels of calcium and phosphate by facilitating their absorption from the gut. Vitamin D also enhances the mobilisation of calcium and phosphate from bone when dietary calcium is inadequate and promotes bone mineralisation by maintaining adequate concentrations of calcium and phosphate in the vicinity of unmineralised osteoid. Fetal 1,25-dihydroxyvitamin D is probably the major stimulus for the placental transfer of calcium.
Other peptide hormones with a more peripheral role in calcium homeostasis
PTH-related peptide (PTHrP)
The circulating levels of PTHrP are low but it is found in breast milk and may have a role in calcium homeostasis in the fetus ( ). PTHrP has an important paracrine role in chondrocyte proliferation and maturation whilst the production of PTHrP by tumour cells is responsible for some cases of tumour-related hypercalcaemia.
Calcitonin
This 32-amino-acid peptide hormone is secreted by the parafollicular C cells of the thyroid in response to hypercalcaemia. It lowers serum calcium by inhibiting bone resorption, increasing urinary calcium and phosphate loss, and probably by decreasing intestinal calcium absorption. Although it can produce hypocalcaemia in humans, neither removal of the thyroid gland nor massive hypersecretion in medullary thyroid carcinoma affects the serum calcium. Hence its physiological significance in humans is unclear, although it may have a role in promoting fetal skeletal mineralisation.
Perinatal calcium metabolism ( )
A baby accumulates 30 g of calcium by term, with 80% of this process occurring during the third trimester ( ). Active transport of calcium and phosphate to the fetus across the placenta is driven by a magnesium adenosine triphosphate-dependent pump which maintains a 1:1.4 mother/fetus gradient, thereby ensuring that the fetus is relatively hypercalcaemic compared with mother ( ; ). This active transport is regulated by CaSRs present in the placenta. The fetus is not affected directly by maternal PTH, 1,25-dihydroxyvitamin D or calcitonin levels as these hormones do not cross the placenta. However, maternal 25-hydroxyvitamin D does cross the placenta, where it may then promote fetal bone mineralisation by stimulating bone osteoblast activity ( ).
Serum total and ionised calcium concentrations are high in cord blood but fall quickly in the first few hours of life as the maternal supply is interrupted. The greatest fall is in ionised calcium, with three-quarters of the total reduction in serum calcium occurring in this fraction ( ). A degree of PTH resistance may also be present, although the levels of this hormone are not usually low ( ). A low milk intake, or blood transfusions that can cause non-ionisable salt formation, may exacerbate hyocalcaemia still further. The nadir in ionised calcium levels occurs around days 2–4 of life, with the low levels stimulating PTH release and a return to adult levels by 2 weeks of age ( ).
Hypocalcaemia ( )
The clinical features of hypocalcaemia in infants include irritability, tremors, twitching and seizures. In contrast, others may be lethargic, feed poorly or vomit. The Chvostek ( ) and Trousseau signs can be unreliable in the newborn examination but the QT interval of the ECG is increased ( ).
Causes
The causes of hypocalcaemia are shown in Table 34.13 .
|
Treatment
Severe symptoms of hypocalcaemia, such as seizures, may be treated with 10% calcium gluconate 0.5–2 ml/kg (0.11–0.46 mmol/kg) by slow i.v. injection over 5–10 minutes. If necessary, this may be followed by continuous infusion of diluted 10% calcium gluconate, 2.5 ml/kg/24 h, with careful monitoring of cardiac rate and rhythm and serum calcium concentration. Calcium solutions are irritant to veins and should ideally be administered via a central venous catheter. Oral calcium supplements (0.25 mmmol/kg qds adjusted according to response) and vitamin D or a vitamin D analogue may be needed for long-term management.
Early hypocalcaemia
Symptomatic hypocalcaemia during the first 3 days of life occurs more commonly in preterm babies, infants of mothers with pre-eclampsia or diabetes and those who suffer birth asphyxia, sepsis or other perinatal stress. To some extent this can be viewed as an exaggeration of the normal postnatal fall in calcium levels. In the preterm infant this reflects a somewhat delayed PTH response to low calcium levels which is compounded by a relative renal resistance to the phosphaturic actions of of PTH. Many infants with early neonatal hypocalcaemia, especially those with diabetic mothers, also have low serum magnesium levels, which, like the hypocalcaemia, usually improve spontaneously.
Late hypocalcaemia due to inappropriate feeds
This clinical picture is now much less common than it was when unmodified cow’s milk formulas were widely used. It usually presented with seizures in apparently normal term infants on the fifth to 10th days of life. Classically this was due to the high phosphate and relatively low calcium content of cow’s milk. Increased absorption of phosphate caused hyperphosphataemia, which in turn depressed the serum calcium.
Hypoparathyroidism ( )
Hypoparathyroidism is a rare cause of hypocalcaemia in the newborn period. It usually presents after 5 days of age with overt signs of hypocalcaemia. Other biochemical findings include hyperphosphataemia, hypomagnesaemia and a normal or low alkaline phosphatase. The diagnosis is confirmed by the finding of low or absent immunoreactive PTH levels at the time of hypocalcaemia.
The condition may be familial, with X-linked recessive, AD and AR inheritance patterns described, and the underlying cause typically reflects an abnormality of gland development, abnormalities of the PTH molecule itself or abnormalities in other aspects of cellular function. Hypoparathyroidism may be an isolated disorder or it may occur in association with other abnormalities.
Isolated hypoparathyroidism
Defects in the the preproPTH gene can give rise to AD and AR isolated hypoparathyroidism ( ; ). The transcription factors GCMB and SOX-3 are involved in parathyroid gland development and mutations can give rise to AR and X-linked recessive hypoparathyroidism, both of which can present in the neonatal period.
DiGeorge syndrome
The most well-known syndrome associated with hypoparathyroidism is the DiGeorge syndrome, which overlaps with the velocardiofacial syndrome ( Ch. 32 ). In this spectrum of phenotypes hypoparathyroidism, thymic aplasia, congenital abnormalities of the heart and great vessels, and other dysmorphic features result from deletions within chromosome 22q11. The deletions involve a variable length of chromosome 22 but when hypoparathyroidsm is present there is typically an abnormality of the TBX1 gene. A small number of cases of DiGeorge syndrome have defects in other chromosomes, notably 10p13 ( ). The presence of hypocalcaemia with congenital abnormalities should prompt detailed genetic analysis, although it should be remembered that calcium levels may return to normal spontaneously.
Other causes of neonatal hypoparathyroidism
Causes of hypoparathyroidism also include the HDR syndrome (hypoparathyroidism, deafness and renal dysplasia) due to defects in the GATA3 gene at 10p15 ( ). This is an AD disorder which can present with hypocalcaemia in early life, although the diagnosis of hypoparathyroidism may be delayed by many years. Other rare causes of hypoparathyroidism include the Kenny–Caffey syndrome (short stature, osteosclerosis and ocular abnormalities) and Sanjad–Sakati syndrome (short stature, ocular abnormalities and learning difficulties). Mitochondrial disease such as Kearns–Sayre syndrome and MELAS (mitochondrial encephalomyopathy, lactic acidosis and stroke-like episodes) can also be associated with hypoparathyroidism.
Management of hypoparathyroidism
The potent water-soluble analogues of vitamin D should be used to treat hypoparathyroidism. A suitable dose in the newborn is alpha-calcidol (1α-hydroxycholecalciferol) or calcitriol (1,25-dihydroxycholecalciferol) 0.03–0.08 µg/kg/24 h up to a maximum of 1–2 µg. The maintenance dose needed varies not only between patients but also in the same patient at different times; frequent measurement of the serum calcium is therefore necessary, with dose adjustments to keep the level within the normal range. Supplementary oral calcium is not essential but may help to stabilise the serum calcium. It should be remembered that a suboptimal vitamin D status is common in early life and so a vitamin D supplement may be required as well (see below).
Other causes of hypocalcaemia
Hypomagnesaemia
Hypomagnesaemia may cause clinically significant hypocalcaemia both by inhibiting the secretion of PTH and by reducing PTH responsiveness ( ). As many as 80% of infants with hypocalcaemic fits may be relatively hypomagnesaemic as well, and the hypocalcaemia may prove to be difficult to correct until the hypomagnesaemia has been addressed. This is most easily done by giving intramuscular (i.m.) or intravenous (i.v.; over at least 10 minutes) magnesium sulphate 0.4 mmol/kg Mg 2+ (100 mg/kg magnesium sulphate) every 8–12 hours. The serum magnesium should therefore be measured in all infants with persistent hypocalcaemia.
There are other, rare, primary abnormalities of intestinal magnesium absorption (AR familial hypomagnesaemia with hypocalcaemia) and renal magnesium handling (primary hypomagnesaemia) which need to be considered in the hypomagnesaemic, hypocalcaemic patient. Hypomagnesaemia may be a feature of Gitelman syndrome and Bartter syndrome.
Maternal hypercalcaemia
In the presence of maternal hypercalcaemia, the fetus is exposed to chronic hypercalcaemia from excessive transplacental passage of calcium and subsequent parathyroid suppression after birth. Whilst this generally resolves spontaneously, maternal calcium levels should be checked whenever there is unexplained hypocalcaemia in the newborn ( ).
Hypercalciuric hypocalcaemia
Hypocalcaemia with hypercalciuria due to activating mutations of the CaSR may present in childhood with seizures. This rare condition should be suspected when hypocalcaemia is associated with hypercalciuria and PTH levels that are within the normal range, in contrast to infants with hypoparathyroidism ( ). It is important to be aware of this condition because vitamin D administration may lead to nephrocalcinosis. No active treatment is needed.
Vitamin D deficiency
Maternal and hence infant vitamin D status is a key determinant of bone mineralisation in the newborn ( ). Vitamin D deficiency can present as neonatal seizures before any change in skeletal phenotype has become apparent. The vitamin D status of an infant will reflect the mother’s diet and her exposure to sunlight, as well as postnatal factors such as the type of feed. Maternal vitamin D deficiency, although particularly common in racial groups with pigmented skin, is found in up to a third of all mothers at the time of delivery even in industrialised nations ( ). Less common but important manifestations of vitamin D deficiency include dilated cardiomyopathy ( ).
Most commercial milk preparations contain enough vitamin D to prevent rickets, but supplementation is still advisable ( ; ). Current recommendations are that all breastfed infants receive 280–400 IU of vitamin D daily from the age of 6 months if consuming <500 ml/day of infant formula, and that ‘at-risk’ groups should start supplements before 6 months. Babies with vitamin D deficiency should be treated with vitamin D (ergo- or cholecalciferol) in a dose of 3000 IU for 2–3 months, with supplements thereafter.
Vitamin D-dependent rickets (VDDR)
VDDR type I is due to defective renal hydroxylation of vitamin D ( ) whereas in VDDR type II there is cellular resistance to hormone action, which is usually due to mutations in the steroid-binding domain or the DNA-binding domain of the vitamin D receptor ( ). Both of these disorders can present with rickets in infancy. Levels of 1,25-dihydroxyvitamin D are reduced in type I VDDR but elevated in type II. The terminology is confusing because VDDR type II will not respond to physiological levels of vitamin D but might to very high doses. VDDR type I is treated with hydroxylated vitamin D metabolites – calcitriol or 1α-calcidol.
Pseudohypoparathyroidism
The term ‘pseudohypoparathyroidism’ is used to describe several related disorders characterised by peripheral unresponsiveness to the action of PTH because of a receptor or postreceptor defect. This is an uncommon cause of hypocalcaemia in infancy which can, for similar reasons, result in TSH resistance and neonatal hyperthyrotropinaemia (see above). The characteristic biochemical findings are hypocalcaemia with hyperphosphataemia, raised levels of PTH and an absent or impaired response to exogenous PTH. These conditions are usually inherited as an AD trait with variable penetrance. Treatment of hypocalcaemia in this disorder requires calcium supplements and a vitamin D analogue (see below).
Miscellaneous causes of neonatal hypocalcaemia
Hypocalcaemia may occur in alkalosis, with citrate administration in blood transfusion, and in hypoalbuminaemic states. It may also arise in infants with renal failure who have hyperphosphataemia.
Hypercalcaemia ( )
Hypercalcaemia is an uncommon problem in infancy and can be defined as a serum calcium above 2.75 mmol/l. The causes of hypercalcaemia are shown in Table 34.14 and are described in more detail below, but conceptually can be thought of as reflecting vitamin D or calcium excess, disease associated with reduced bone formation (hypophosphatasia) or increased bone resorption (e.g. some tumours). Occasionally a raised serum calcium can reflect an abnormality of the CaSR (hypocalciuric hypercalcaemia) ( ) with an associated altered ‘set-point’, and it may also be a manifestation of Williams syndrome. Primary hyperparathyroidism (as opposed to neonatal severe hyperparathyroidism resulting from a CaSR defect) does not occur in infancy. Clinical manifestations of hypercalcaemia include hypotonia, weakness and irritability, poor feeding, weight loss, constipation, vomiting, polydipsia and polyuria. Hypercalciuria can give rise to nephrocalcinosis.
Treatment
Whilst the primary cause must be corrected if possible, the key component of short-term treatment of neonatal hypercalcaemia is a reduction in dietary calcium intake using low-calcium feed. Generous hydration and furosemide diuresis promote urinary calcium loss if a more acute reduction is needed. Glucocorticoids (e.g. hydrocortisone 1 mg/kg 6-hourly) reduce intestinal calcium absorption but the effect is slow and they should not be used if neoplasia is suspected. Calcitonin (10 U/kg i.v.) may be useful and has its maximum effect in 1 hour; infusions may be repeated 4-hourly. The hormone is antigenic and so the synthetic derivative salcatonin should be used in the longer term. Experience with the bisphosphonates is increasing ( ) and pamidronate in a dose of 0.5–1.0 mg/kg as an i.v. infusion may be worth considering in particularly difficult cases ( ). Long-term safety data following administration in infancy are not yet available.
Causes of hypercalcaemia ( Table 34.14 )
|
Hypercalcaemia in association with excess vitamin D
Infantile hypercalcaemia was relatively common in the UK when foods were liberally supplemented with vitamin D. It generally presented with signs of hypercalcaemia in the early months of life, but was generally mild and self-limiting (Lightwood syndrome). The incidence fell when the use of vitamin D supplements was reduced. It is thought to have affected infants with a particular sensitivity to vitamin D in whom the cumulative daily vitamin D dose could reach 2000–4000 IU or more. Hypercalcaemia due to excessive vitamin D intake has been reported in association with prolonged administration of preterm formula ( ).
Williams syndrome ( Ch. 31 )
Williams syndrome is a contiguous gene deletion disorder caused by hemizygous loss of 1.5–1.8 Mb on chromosome 7q11.23 (see Ch. 32 for details of the phenotype). Hypercalcaemia is present in around 15% and tends to resolve spontaneously, but the other features persist. The condition is usually sporadic, although familial AD inheritance has also been observed. In the hypercalcaemic phase, treatment is with a low-calcium milk/diet and then a cautious reintroduction of calcium once the biochemistry has been normalised. Controlling serum calcium does not affect the progression of the other features of the disease.
Hypercalcaemia due to phosphate depletion
Severe hypercalcaemia may occur in LBW preterm infants with hypophosphataemia due to a low phosphate intake from breast milk or parenteral feeding. The hypercalcaemia responds to phosphate repletion ( ).
Benign familial hypocalciuric hypercalcaemia and neonatal severe hyperparathyroidism
These disorders are associated with mutations in the extracellular calcium ion-sensing receptor which lead to loss of function. The ‘set-point’ at which PTH is released is therefore altered. Benign familial hypercalcaemia is inherited as an AD trait (one abnormal allele), whereas the severe neonatal form is associated with both heterozygous and homozyous mutations of the CaSR gene ( ). Factors influencing the phenotype in this disorder include the number of mutant genes as well as the extent to which the calcium receptor is compromised. The phenotype may also be influenced by the pattern of inheritance: if the infant inherits the mutant allele from the father, then PTH production will be more exaggerated, because of the discrepancy between the maternal and fetal CaSR ‘set-point’ in utero. The neonatal form can lead to hypotonia, respiratory distress and failure to thrive, in association with hypercalcaemia and elevated PTH levels. There is skeletal undermineralisation, with rib fractures and subperiosteal erosions. The treatment of severe cases, usually homozygous for receptor mutations, may entail urgent parathyroidectomy, but some neonatal cases appear to run a milder, self-limiting course ( ). Some of these cases will be heterozygote infants with the abnormal allele inherited from the father. Earlier reports of primary hyperparathyroidism in neonatal life probably included children with calcium receptor abnormalities. Bisphosphonates may help to protect the skeleton from the harmful effects of hyperparathyroidism prior to surgical intervention ( ).
Hyperparathyroidism secondary to maternal hypoparathyroidism
Secondary hyperparathyroidism may be due to untreated maternal hypoparathyroidism, which causes fetal hypocalcaemia and parathyroid hyperplasia; this condition is self-limiting but the hypercalcaemia may need treatment with hydration and calcitonin.
Subcutaneous fat necrosis
Hypercalcaemia may occur in association with extensive neonatal subcutaneous fat necrosis, which is seen especially after traumatic delivery of large infants, or cold injury. This may be due to unregulated production of 1,25-dihydroxyvitamin D by the affected adipose tissue ( ).
Hypophosphatasia ( )
Hypophophatasia is an AR disorder due to defects in the gene for tissue non-specific (bone/liver/kidney) alkaline phosphatase.
The perinatal form is characterised by extreme skeletal hypomineralisation and babies often die in utero or in early postnatal life. The infantile form is defined by a presentation before the age of 6 months. Clinical features include poor feeding, hypotonia, craniotabes, blue sclerae and a rachitic deformity of the limbs. Hypercalcaemia and hypercalciuria reflect poor skeletal growth and mineralisation. The infantile form of the disease is fatal in approximately 50% of patients and, whilst treatment options are limited, trials of recombinant alkaline phosphatase are showing promising results.
Idiopathic infantile hypercalcaemia ( )
Idiopathic infantile hypercalcaemia remains a poorly defined entity that is diagnosed once other causes have been excluded. Infants can present with the symptoms and signs of hypercalcaemia, including polyuria and polydipsia, and some have features in common with Williams syndrome, such as facial dysmorphism and cardiac defects ( ). There is recent evidence to suggest that the hypercalcaemia is linked to abnormal vitamin D metabolism as well as abnormal PTHrP production. The hypercalcaemia can be more difficult to treat than in Williams syndrome and may persist for longer.
Miscellaneous causes of hypercalcaemia
Vitamin A excess, adrenal failure, drugs such as thiazide diuretics, muscle disease with associated inactivity and malignancy are rare causes of hypercalcaemia in early life. Constitutively activating PTH receptor mutations result in Jansen syndrome and a phenotype that includes hypercalcaemia and short-limbed dwarfism ( ).
Adrenal
Normal development and function
The adrenal gland lies adjacent to the upper pole of the kidney and has two embryologically and functionally distinct components. The medulla is formed from neural crest cells which enter the gland at about the seventh week of gestation and secrete catecholamines. Clinically significant abnormalities of medullary function are not recognised in the newborn. The cortex is derived from mesodermal cells near the cephalic part of the mesonephros. It shares common primordial cells with the gonads, which also secrete steroids and may express similar enzyme deficiencies. The fetal adrenal is 20–30 times larger than the adult gland relative to body weight (twice as large in absolute terms), which reflects its contribution to oestrogen production in utero. At birth both glands together weigh 7–9 g. They are largely composed of the histologically distinct fetal cortex, which constitutes 80% of the gland at birth and which then involutes rapidly, reducing to half its size by 2 weeks and disappearing by 6 months.
The enzyme activities of the fetal adrenal and the placenta are complementary; thus the placenta lacks the enzymatic activity necessary to generate androgens from the precursors, pregnenolone and progesterone (17α-hydroxylase and 17,20-desmolase; Fig. 34.12 ), whereas the fetal adrenal lacks the enzymatic activity to produce oestrogen from androgens, until the later stages of gestation. The adrenal androgens (primarily dehydroepiandrosterone and its sulphate) are converted to oestrogen by the placenta, which expresses the necessary sulphatase and aromatase enzyme activity. Hence the fetal adrenal provides the precursors necessary for placental oestrogen production, and steroid production by the fetoplacental unit as a whole promotes maturation of organs such as the lungs ( ). A deficiency of placental aromatase leads to virilisation of both the mother and female infants during pregnancy ( ; ).
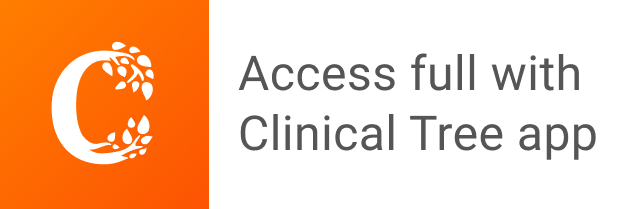