X-linked adrenoleukodystrophy is a metabolic disorder that predominantly affects the central nervous system (CNS), adrenal glands, and testicles. The clinical manifestations of this disorder relate to excessive accumulation of very long chain fatty acids (particularly hexacosanoic acid) in tissues and plasma. The underlying defect is failure of normal peroxisomal oxidative degradation (Table 18-1). Abnormalities of adrenal function are present in most children with symptomatic cerebral X-linked adrenoleukodystrophy. Almost all symptomatic patients with this disorder are hemizygotic males. MR evidence of white matter abnormalities is present in 10% to 20% of female carriers. The estimated prevalence of X-linked adrenoleukodystrophy is 1:20,000 to 1:100,000 male births.1,2
Based on the clinical features, X-linked adrenoleukodystrophy is classified into several phenotypes: childhood cerebral X-linked, adolescent cerebral X-linked, adult cerebral X-linked, adrenomyeloneuropathy, Addison disease– only type, and asymptomatic type. Childhood cerebral X-linked adrenoleukodystrophy is the most severe form. Most of these children develop normally until the later part of the first decade of life, at which time neurological alterations such as memory impairment and emotional instability develop, followed by progressive deterioration of vision, hearing, and motor function. Manifestations of adrenal dysfunction and gonadal insufficiency can also occur. The prompt institution of therapy (diet control, medication, or bone marrow transplantation) following an early diagnosis modifies the clinical course of this disorder.
In the childhood form of cerebral X-linked adrenoleukodystrophy, the typical CNS lesions include extensive demyelination in the periventricular deep white matter (especially in the parieto-occipital areas), cavitation, and perivascular lymphocytic infiltrates. There is sparing of the U fibers and cortex. The cerebral white matter lesions are divided histopathologically into 3 distinct zones: an outermost zone (Schaumburg zone 1), with active destruction of the myelin sheath and a lack of perivascular inflammatory cells; a middle layer zone (Schaumburg zone 2), characterized by perivascular inflammatory cells and demyelination, with preservation of axons; and a central zone (Schaumburg zone 3), consisting of gliosis and scattered astrocytes in the absence of oligodendroglia, axons, myelin, and inflammatory cells.
Areas of brain involvement with X-linked adrenoleukodystrophy have abnormal high signal intensity on T2-weighted MR images and diminished attenuation on CT. Diffusion-weighted sequences may demonstrate elevated apparent diffusion coefficient (ADC) values (i.e., increased diffusion). The site of greatest involvement for approximately 80% of patients is the parieto-occipital white matter and the splenium. The splenium is enlarged in the acute phase, but eventually becomes atrophic. Other potential sites include the genu of the corpus callosum, visual pathway (optic radiation, lateral geniculate body), acoustic pathway (acoustic radiation, medial geniculate body, brachium of the inferior colliculus, lateral lemniscus), corticospinal tract in the brainstem, and middle cerebellar peduncles. Areas of active inflammation undergo prominent contrast enhancement (Figure 18-1). In some patients, this appears as a band at the leading edge of demyelination.
Loes et al described 5 MRI patterns of X-linked adrenoleukodystrophy.3 Pattern 1 is primary involvement of the deep white matter in the parietal and occipital lobes and of the splenium of the corpus callosum; this may include lesions of the visual and auditory pathways (Figure 18-2). This is the most common pattern overall, and the typical finding in symptomatic young children. Pattern 2 refers to involvement of the frontal lobe or genu of the corpus callosum; this is most often identified in adolescents. Pattern 3, which most often occurs with adult-onset disease, refers to primary involvement of the frontopontine or corticospinal projection fibers. Pattern 4 is a rare finding, with primary cerebellar white matter involvement. Pattern 5 is combined involvement of the parieto-occipital and frontal white matter. There is substantial variation in disease progression between patients with X-linked adrenoleukodystrophy.
Followup MR imaging may demonstrate stabilization, improvement, or progression of the brain lesions with extension of prior lesions into adjacent brain tissue and the development of new lesions in other areas of the brain.4
Metachromatic leukodystrophy is an autosomal recessive lysosomal storage disorder that occurs due to a deficiency of arylsulfatase-A (ARSA) or the cofactor saposin-B (Table 18-2). The responsible gene is ARSA. ARSA is a lysosomal enzyme that is necessary for the normal metabolism of sulfatides, which are important constituents of the myelin sheath. In patients with metachromatic leukodystrophy, sulfatides accumulate in various tissues, including the brain, kidneys, peripheral nerves, liver, and gallbladder. In the brain, the accumulation of ceramide sulfatide within glial cells and neurons causes functional impairment. The name of this disorder derives from the resulting metachromatic reaction. Demyelination in these patients is usually most prominent in the centrum semiovale. Laboratory examination demonstrates subnormal levels of ARSA in peripheral blood leukocytes and in urine.
Disorder | Deficient enzyme |
---|---|
Metachromatic leukodystrophy | Arylsulfatase-A |
Krabbe disease | Galactosylceramide β-Galactosidase |
GM1 gangliosidosis | β-Galactosidase |
GM2 gangliosidosis | N-Acetylhexosaminidase |
Niemann-Pick disease | Sphingomyelinase |
Mucopolysaccharidosis | Various |
Fucosidosis | Fucosidase |
Mucolipidosis | N-Acetylglucosamine-1-phosphotransferase |
Wolman disease | Acid lipase |
Neuronal ceroid lipofuscinosis | Various |
The age at clinical onset is the basis for the classification of metachromatic leukodystrophy: late infantile, juvenile, and adult. In general, the age at presentation and the severity of symptoms correlate with the level of ARSA activity. The late infantile type is most common. These patients usually present between the ages of 12 and 18 months with manifestations of peripheral neuropathy. Typically, there is rapid progression in neurologic deterioration, gait disturbance, quadriplegia, blindness, and loss of speech skills. Most children with the late infantile form of the disease die within several months to a few years after the onset of symptoms. The juvenile form usually has a more insidious onset and slower progression; the peak age at presentation is between 5 and 7 years.5
Neuroimaging studies of metachromatic leukodystrophy show demyelination that most markedly involves the central cerebral white matter. This appears as white matter hypoattenuation on CT and hyperintensity on T2-weighted spin-echo, fluid-attenuated inversion recovery (FLAIR), and diffusion-weighted MR images (Figure 18-3). (Metachromatic leukodystrophy and Canavan disease are the metabolic brain disorders that most often cause restricted diffusion on MR evaluations.) Typically, MR demonstrates symmetric confluent areas of white matter involvement, with sparing of the subcortical U fibers. The earliest areas of involvement are the posterior cerebral periventricular white matter and the cerebellar white matter. There is no abnormal contrast enhancement. Relatively characteristic “tigroid” and “leopard skin” patterns of demyelination (linear lower signal intensity stripes radiating peripherally through the hyperintense white matter) are sometimes present in the periventricular white matter and centrum semiovale; this is due to sparing of the perivascular white matter. The corpus callosum, internal capsule, and corticospinal tracts often develop abnormal signal intensity later in the course. With progressive disease, the subcortical white matter may become involved. Manifestations of atrophy are common in late-phase disease. MR spectroscopy of patients with metachromatic leukodystrophy shows diminished choline.6–9
Krabbe disease (globoid cell leukodystrophy) is an autosomal recessive lysosomal storage disorder that is due to a deficiency of galactosylceramide β-galactosidase. The deficient lysosomal enzyme degrades cerebroside, which is a normal constituent of myelin. Normal myelin turnover within the developing brain of the infant with Krabbe disease leads to the accumulation of galactosylsphingosine in the lysosomes of macrophages and other cells in the white matter; this compound is toxic to neurons and glial cells. Pathologically, there is neuronal destruction in the thalami, cerebral cortex, cerebellar dentate nuclei, and inferior olivary nuclei. Demyelination and axonal destruction occur throughout the CNS white matter. The involved macrophages are the “globoid cells” that are the histological hallmark of this disorder. The mutation responsible for Krabbe disease is in the GALC gene on chromosome 14q24.3–32.1. Hematopoietic stem cell transplantation halts disease progression in some patients.10
There is substantial genetic and clinical heterogeneity of Krabbe disease. Symptoms develop prior to 6 months of age in approximately 80% of patients. As with many of the genetic white matter diseases, the clinical classification correlates with the patient age at onset of symptoms: infantile, late infantile (19 months to 3 years), juvenile (4 to 19 years), and adult. The infantile form is most common. The typical findings include hyperirritability, increased muscle tone, fever, and neurodevelopmental regression. Subsequent manifestations include myoclonus, opisthotonus, and nystagmus. Most patients suffer a rapidly progressive, ultimately fatal, course. Common features of the late infantile variant include ataxia, weakness, spasticity, visual loss, and mental regression. The juvenile onset variant is associated with slowly progressive spastic tetraplegia, sensorimotor neuropathy, and variable compromise of mental function.11
Because of microcalcification, the early CT findings of Krabbe disease include symmetric high attenuation foci in the thalami, caudate nuclei, corona radiata, posterior limbs of the internal capsules, cerebellar dentate nuclei, and brainstem. In some patients, this finding precedes the general white matter hypoattenuation that eventually develops. The appearance on MRI is often nonspecific. The affected areas of the brain produce high signal intensity on T2-weighted images. The earliest areas of involvement usually include the cerebellar white matter, cerebellar nuclei, and the posterior limbs of the internal capsules. There is subsequent involvement of the deep cerebral white matter and corpus callosum. There is sparing of the subcortical white matter early in the disease. Thalamic signal abnormality occurs in some patients, usually as a late finding. Severe generalized encephalomalacia occurs with progression. MR sometimes shows slight enhancement at the junction of the subcortical U fibers with the underlying abnormal white matter. Cranial nerve and cauda equina enhancement can also occur. MR spectroscopy in infantile Krabbe disease demonstrates elevation of choline and myoinositol, reduced N-acetylaspartate (NAA), and elevation of creatine.12–14
Maple syrup urine disease is an aminoacidopathy caused by an enzyme defect in the catabolic pathway of the branched-chain amino acids leucine, isoleucine, and valine. CNS accumulation of these 3 amino acids and their corresponding keto acids leads to encephalopathy and progressive neurodegeneration. The term “maple syrup” derives from the odor that excessive isoleucine imparts to the urine of these patients. The prevalence of maple syrup urine disease in the United States is approximately 1 per 180,000 newborns. There is a much higher frequency in some inbred populations, such as the Mennonites of Pennsylvania (1 in 176 newborns). There is no gender predilection. Dietary intervention (restriction of branched-chain amino acids) greatly diminishes the effects of the disorder and may allow for normal intellectual development; therefore, an early diagnosis is paramount.
The biochemical basis of maple syrup urine disease is a deficiency of the branched-chain α-keto acid dehydrogenase (BCKD) complex. This enzyme complex catalyzes the decarboxylation of the α-keto acids of leucine, isoleucine, and valine to their respective branched-chain acyl-CoAs. Further, metabolization produces acetyl-CoA, acetoacetate, and succinyl-CoA. The accumulation of leucine is particularly important in the neurological pathophysiology of this disease, as leucine is transported rapidly across the blood-brain barrier. Mutations of any of the 3 catalytic components of BCKD lead to maple syrup urine disease; these components are E1, E2, and E3.15
The age of onset, severity of clinical symptoms, and response to dietary treatment distinguish 5 distinct clinical phenotypes of maple syrup urine disease: classic, intermediate, intermittent, thiamine-responsive, and E3-deficient. Most common is the classic form, with rapid onset of neurological signs early in infancy. Common findings include alternating muscular hypotonia and hypertonia, dystonia, opisthotic positioning, seizures, and encephalopathy. Ketosis and the characteristic odor of the urine usually are present when the first symptoms develop. Without medical intervention, these infants may develop manifestations of increased intracranial pressure, potentially progressing to coma and death.
Intermittent maple syrup urine disease is the second most common form. These children experience normal growth and intellectual development, but may suffer acute neurological symptoms, such as ataxia, seizures, lethargy, or coma, during episodes of catabolic stress (e.g., otitis media or pneumonia). The other 3 forms of maple syrup urine disease are rare. The intermediate form is associated with neurological impairment, developmental delay, and seizures; the age at presentation and the severity vary between patients. The E3-deficient form has a similar clinical presentation as the intermediate type, with the exception of accompanying lactic acidosis.
Neuroimaging studies of the neonate with the classic form of maple syrup urine disease demonstrate localized edema in the regions of the brain that are myelinated or myelinating: the cerebral peduncles, posterior limbs of the internal capsules, perirolandic white matter, central cerebellar white matter, corticospinal tracts of the brainstem, and (variably) globi pallidi. With severe involvement or an acute exacerbation, there may be findings of generalized brain edema and increased intracranial pressure. Demonstration of localized or generalized edema in these infants is by sonography (hyperechogenicity), CT (hypoattenuation), or MR (T2 hyperintensity). With MR, diffusion-weighted images often provide the greatest conspicuity (Figure 18-4). MR spectroscopy indicates slight reduction of NAA, mild elevation of lactate, and a broad peak at 0.9 ppm due to the accumulation of branched-chain amino acids and ketoacids. In the absence of prompt effective treatment, imaging later in childhood shows encephalomalacia. Neuroimaging of older children with the milder forms of maple syrup urine disease demonstrates lack of brain myelination, most prominently in the areas described above.16–18
Figure 18–4
Maple syrup urine disease.
This 9-month-old infant with classic type maple syrup urine disease suffered an acute decompensation following a viral illness. A. There is abnormal hyperintensity in the globi pallidi and internal capsules on this T2-weighted MR image. B. A diffusion-weighted image shows hyperintensity in the internal capsules, globi pallidi, cerebral peduncles, and brainstem.

Phenylketonuria is an autosomal recessive disorder of amino acid metabolism, in which there is failure of conversion of phenylalanine into tyrosine. In most patients, the cause is a mutation of the PAH gene on chromosome 12q22.24.1, thereby resulting in a deficiency of phenylalanine hydroxylase. Deficiency of phenylalanine hydroxylase leads to the accumulation of metabolites that are toxic to the developing brain. Untreated phenylketonuria typically leads to severe intellectual impairment. Neonatal screening allows early detection of this disorder. Prompt initiation of dietary restriction of phenylalanine substantially diminishes the severity of neurological sequelae in most patients. However, there is clinical heterogeneity in the response to therapy. The adequacy of patient and parental adherence to dietary therapy also affects the clinical and radiographic manifestations of the disease.
The major CNS consequences of phenylketonuria are delayed or defective myelination, white matter vacuolation, and gliosis (Figure 18-5). Therefore, FLAIR and standard T2-weighted MR images demonstrate symmetrical white matter hyperintensity. There is usually sparing of the subcortical white matter, particularly early in the course of the disease. In some affected children, the cerebral white matter abnormality is most pronounced in the frontal and peritrigonal regions. Patients with substantial elevation of serum phenylalanine levels sometimes have restricted white matter diffusion; this finding is usually absent in patients with therapeutically controlled disease. Prominent phenylalanine in the brain leads to a peak at 7.37 ppm on proton MR spectroscopy. Individuals with phenylketonuria may have brain volume loss and secondary ventriculomegaly. Atrophy is most prominent in the pons, hippocampus, cerebellum, and corpus callosum.19–22
Figure 18–5
Phenylketonuria.
A. There is deficient myelination throughout most of the cerebral white matter on this T2-weighted image of a 4-year-old child. B. An axial FLAIR image shows more pronounced signal abnormality in the frontal and posterior parietal regions, suggesting gliosis. C. The corpus callosum and the brainstem are small.


There are various forms of hyperphenylalaninemia in addition to the classic variety of phenylketonuria described above. Some of these patients have progressive neurological symptoms despite appropriate dietary intervention that maintains normal phenylalanine levels, that is, “malignant phenylketonuria.” These patients usually have defects in the synthesis of tetrahydrobiopterin (due to 6-pyruvoyltetrahydropterin synthase deficiency), the cofactor for phenylalanine hydroxylase, or the enzymes that regenerate tetrahydrobiopterin from dihydrobiopterin. These various defects result in deficient conversion of phenylalanine to tyrosine, despite the presence of normal phenylalanine hydroxylase. MR imaging in these patients typically shows T2 hyperintensity in the cerebrum. Involvement of the lentiform nuclei, particularly the putamina, is common. Putaminal calcifications are sometimes present. Proton spectroscopy may indicate lactate peak elevation as well as elevations of the N-acetylaspartate:creatine and N-acetylaspartate:choline ratios. As with classic phenylketonuria, the neuroimaging findings correlate to some extent with the clinical severity of disease and the adequacy of therapy.23,24
Cystathionine β-synthase deficiency is an autosomal recessive disorder of homocysteine metabolism. The homozygous form of this disorder is the most frequent cause of severe hyperhomocystinemia; the prevalence is approximately 1 per 200,000 individuals. Dietary therapy or vitamin B6 supplementation is effective for many patients. CNS involvement with this form of hyperhomocystinemia is predominately due to arterial disease. These patients may develop atherosclerosis, arterial thromboembolism, and venous thrombosis. Strokes are common in patients of all ages with this disorder, including children. The most common neuroimaging finding is the presence of multiple small foci of encephalomalacia from prior infarcts. MR angiography may demonstrate carotid or intracranial arterial disease. Occasionally, MR shows findings of demyelination and spongy degeneration of the white matter.25
Methylenetetrahydrofolate reductase deficiency is a heritable metabolic error in the methionine synthesis pathway that is due to mutations of the MTHFR gene at 1p36.3. Deficient activity of this enzyme leads to elevated homocysteine levels in the blood and urine, as well as diminished methionine levels in blood and cerebrospinal fluid (CSF). Vascular pathology in these patients is usually mild, and cerebral infarcts are rare. However, the diminished methionine levels result in deficiency of S-adenosyl-L-methionine, which is involved in myelin synthesis.
Potential neurological manifestations of methylenetetrahydrofolate reductase deficiency in severely affected infants include hypotonia, marked developmental delay, apneic episodes, respiratory failure, and death. Mutations that result in a milder form of the disease may be associated with a clinical presentation later in childhood or adolescence, with intellectual impairment, seizures, and neuropathy. MR examination of patients with methylenetetrahydrofolate reductase deficiency often demonstrates T2 hyperintensity in the white matter due to demyelination. In patients with early or mild disease, there may be multiple small foci of signal abnormality in the white matter.26,27
Nonketotic hyperglycinemia is an autosomal recessive disorder of glycine metabolism. A defect in the glycine cleavage enzyme system leads to elevation of glycine levels in various tissues. Glycine serves as an inhibitory neurotransmitter in the spinal cord and as a modulator of excitation at the N-methyl-D-aspartate receptors in the cerebral cortex, hippocampus, and cerebellum. Elevation of glycine levels in the CNS leads to intractable seizures, lethargy, and hypotonia. Affected neonates may have apneic episodes. Typically, the clinical onset is within the first few days of life. The course is usually fulminant.
The major neuropathological feature of nonketotic hyperglycinemia is a spongy myelinopathy, with micro-cysts located between myelin sheaths. The areas of greatest involvement include the brainstem, posterior limbs of the internal capsules, cerebellar peduncles, optic tracts, and optic chiasm. Neuroimaging studies demonstrate generalized brain volume loss. The typical findings in young infants are T2 hyperintensity and restricted diffusion in the areas of the brain that are normally undergoing myelination at this age. With progression, larger areas of white matter become abnormal, and there is progressive brain volume loss; diffusion restriction may disappear. The corpus callosum becomes thin. Proton MR spectroscopy may show an abnormal peak at 3.56 ppm, apparently representing glycine.28,29
Salla disease is a recessively inherited lysosomal storage disease that is due to an error in sialic acid (N-acetylneuraminic acid) metabolism caused by mutation of the SLC17A5 gene. This gene encodes sialin, a lysosomal membrane sialic acid transporting protein. In addition to classic Salla disease, there are variant lysosomal free sialic acid storage diseases, such as infantile sialic acid storage disease. All forms involve the abnormal accumulation of free sialic acid in the lysosomes of various tissues because of a defect in the mechanism of transport across the lysosomal membrane. The major pathological consequence in the CNS is defective myelination.30–32
The clinical presentation of Salla disease typically consists of manifestations of psychomotor retardation that become evident around 3 to 6 months of age. These infants often have hypotonia, ataxia, and nystagmus. Disease progression is usually quite slow. There is only mild decrease in life expectancy. Young children with the classic type of Salla disease develop some language skills and the ability to walk. About one-quarter of patients have a more severe phenotype, with progressive spasticity and severe ataxia. Seizures occur in approximately 20% of patients with Salla disease.
MRI of young infants with Salla disease is sometimes normal. Subsequently, however, there is lack of normal myelination. The corpus callosum is thin. The cerebral white matter has higher signal intensity than gray matter on T2-weighted images. Some degree of myelination usually occurs in the internal capsules. Myelination of the brainstem is often delayed or abnormal. Cerebellar myelination is usually normal. In some patients, white matter signal abnormality is homogeneous and diffuse. In others, limited myelination eventually occurs in the periventricular region. Atrophy is lacking or minimal with both of these types. A third, clinically more severe, variant has generalized brain atrophy, lack of cerebral myelination, and high periventricular signal intensity on T2-weighted images. MR spectroscopy in patients with Salla disease shows elevation of creatine and NAA and diminution of choline in the affected white matter. In the basal ganglia, creatine is elevated and NAA and choline are normal.33
Pelizaeus-Merzbacher disease is a clinically severe dysmyelinating disease that results from deficiency of myelinspecific lipids. There is failure of normal brain myelination (Table 18-3). The PLP1 gene at Xq22 encodes for a protein (proteolipid protein 1) and its isoform (DM20) that are crucial for myelin formation. There are accompanying deficits of other myelin proteins of the CNS in these patients. Mutations that cause conformational change of the protein and gene duplications that induce excessive biosynthesis of the protein usually lead to the most severe clinical forms of the disease. Gene deletions that result in the total absence of proteolipid protein 1 usually are associated with a more benign phenotype.
The clinical classification of Pelizaeus-Merzbacher disease is into classic and connatal forms. The classic type begins during late infancy and has X-linked recessive inheritance. These patients usually survive until late adolescence or early adulthood. The connatal form is much less common and is clinically more severe than the classic variety. The clinical onset is at birth or in early infancy; death usually occurs in early childhood. The connatal form has either X-linked or autosomal recessive inheritance. Clinical manifestations common to both forms of Pelizaeus-Merzbacher disease include nystagmus, extrapyramidal hyperkinesias, spasticity, and poor psychomotor development.34
Neuroimaging studies of children with Pelizaeus-Merzbacher disease demonstrate marked lack of brain myelination. The abnormality is often not apparent until the second year of life. The imaging appearance of the brain in affected children is often similar to that of a normal newborn (Figure 18-6). There is no substantial inflammation, so T2 hyperintensity is not as pronounced as in some other leukodystrophies. Diffusion-weighted, T2-weighted spin-echo, and FLAIR MR images show diffuse white matter hyperintensity, or lack of the normal hypointensity of myelinated white matter. There is involvement of the subcortical U fibers. Signal changes in the internal capsule occur early in the course. In some patients, there are scattered small foci of relatively normal signal intensity within the cerebral white matter, reflecting a patchy pattern of dysmyelination and islands of preserved myelin. This pattern most often occurs within perivascular tissue, thereby producing a “tigroid” appearance. With progression, there is brain volume loss that predominantly involves the white matter. The findings on proton spectroscopy vary substantially between patients, apparently reflecting the genetic heterogeneity of the disease and alterations in brain biochemistry at different phases of the disorder. The most common finding is reduced NAA in affected white matter. Mild elevations of choline and creatine are sometimes present as well.35–37
Alexander disease (fibrinoid leukodystrophy) is a rare, nonfamilial leukoencephalopathy that typically results in macrocephaly and white matter abnormalities with a frontal preponderance. The cause of Alexander disease is a de novo mutation of the gene (GFAP) for glial fibrillary acidic protein, located at chromosome 17q21. Over expression of the GFAP gene apparently leads to cytoplasmic aggregation of an intermediate filament protein and secondary cellular dysfunction. The histological features include massive deposition of Rosenthal fibers (dense eosinophilic rodlike cytoplasmic inclusions within astrocytes) in the subependymal, subpial, and perivascular regions. There is a severe paucity of myelin. Areas of cystic degeneration within the white matter are sometimes present. The areas of greatest involvement are the frontal lobe white matter, basal ganglia, and thalami. There is relative sparing of the occipital lobes and the cerebellum.38
There are 3 clinical subgroups of Alexander disease. (1) Patients with the infantile type (most common) have early onset of macrocephaly, psychomotor retardation, and seizures. Most of these children die by 2 to 3 years of age. A neonatal variant is associated with a more rapid progression to death. (2) In the juvenile subgroup, the clinical onset usually occurs between 7 and 14 years of age. Spasticity and progressive bulbar symptoms are typical. Macrocephaly is uncommon in the juvenile subgroup. (3) In the adult subgroup, the onset of symptoms can occur at any age; the clinical manifestations sometimes mimic those of multiple sclerosis.39
Neuroimaging studies of Alexander disease demonstrate extensive white matter abnormalities that predominantly involve the frontal lobes in the early phase of the process. CT demonstrates low attenuation in the deep frontal lobe white matter (Figure 18-7). There may be contrast enhancement adjacent to the frontal horns. On MR, the affected white matter is hyperintense on T2-weighted images. Signal abnormalities are also common in the basal ganglia and thalami; the caudate heads and the anterior portions of the putamina are the initial sites of basal ganglia involvement. The cerebellar white matter is sometimes involved. Hyperintensity on T2-weighted images may be present in the dorsal aspect of the medulla and the periaqueductal region of the mid-brain. T1-weighted images often show a characteristic hyperintense periventricular rim, apparently due to an extreme density of Rosenthal fibers in the periventricular white matter. In addition to the deep frontal white matter, there is often concomitant involvement of subcortical white matter at this early phase. Regions of the brain that may enhance abnormally with IV contrast include the frontal white matter, ependyma of the trigones, portions of the basal ganglia (early in the disease), and regions of the brainstem. With disease progression, the frontal white matter hyperintensity extends posteriorly into the parietal white matter and internal and external capsules. In the late stages of the disease, cysts sometimes develop in the diseased white matter. Affected regions of the brain may have elevated concentrations of myoinositol and reduced NAA on proton MR spectroscopy. Some patients with juvenile Alexander disease have MR signal abnormalities predominantly located in the medulla and/or spinal cord, with minimal or no involvement of cerebral white matter other than a thin periventricular rim.40–42
Cockayne syndrome is a multisystem autosomal recessive disorder that leads to profound postnatal growth failure, premature senescence, and progressive multiorgan degeneration. There are 3 distinct genetic forms: (1) CS-A is linked to mutations of the CSA gene on chromosome 5. This is a less severe form that usually is associated with survival into adolescence or young adult life. (2) CS-B is a more prevalent (80%) and severe infantile form that usually results in death early during childhood. This variant is linked to mutations of the CSB gene on chromosome 10q11. (3) The XP/CS complex variant is due to mutations in any of the 4 xeroderma pigmentosum genes: XPB, XPC, XPD, and XP. Patients with the XP/CS complex experience photosensitivity as well as severe developmental and neurological dysfunctions.43–45
Neuroimaging studies of patients with Cockayne syndrome demonstrate generalized cerebral and cerebellar hypoplasia/atrophy, with parenchymal thinning and ventriculomegaly. CT shows foci of dystrophic calcification, predominantly located in the basal ganglia and cerebellar dentate nuclei (Figure 18-8). On T2-weighted MR images, there is abnormal hyperintensity in the basal ganglia, cerebellar dentate nuclei, and periventricular cerebral white matter. There is usually progressive involvement of the peripheral portions of the cerebral white matter with time. Dystrophic calcifications of sufficient size produce signal loss on gradient echo images.46,47
The classic form of galactosemia causes various CNS complications. Patients with this autosomal recessive disorder are unable to convert galactose into glucose because of deficient activity of galactose-1-phosphate uridyltransferase. The resultant accumulation of galactose in tissues causes serious complications in the liver, kidneys, eyes (cataracts), and brain. About three-quarters of untreated infants with galactosemia die. Despite early diagnosis and dietary intervention beginning at birth, most patients suffer neurological complications, such as speech and language delays, learning disabilities, and motor skill delays.
Neuroimaging studies of patients with the classic form of galactosemia show manifestations of white matter damage. CT may demonstrate diffuse hypoattenuation of the cerebral white matter in adolescents and adults with galactosemia. The brain white matter usually appears normal on T1-weighted MR images. T2-weighted images typically are also normal during infancy. In older children, T2-weighted images often demonstrate lack of normal hypointensity in the peripheral cerebral and cerebellar white matter; this may represent biochemically abnormal myelin. About one-third of patients have mild ventriculomegaly. Manifestations of cerebellar atrophy occasionally develop. Small focal cerebral white matter lesions are present in some patients. MR spectroscopy is often normal in patients with galactosemia; occasionally, the presence of elevated levels of galactitol in an untreated patient produces a doublet at 3.7 parts per million.48,49
Leukoencephalopathy with vanishing white matter (vanishing white matter disease; childhood ataxia with diffuse CNS hypomyelination) is an autosomal recessive neurological disease caused by mutations in the genes encoding subunits of the eukaryotic translation initiation factor EIF-2B. The clinical presentation is usually during the first decade of life. The predominant clinical features are ataxia and spastic diplegia.
The characteristic MRI pattern of vanishing white matter disease is diffuse cerebral white matter alteration such that the signal intensity is similar to CSF on T1-weighted, FLAIR, and T2-weighted spin-echo sequences. Early in the course, subcortical white matter involvement predominates. There is cerebellar atrophy of variable severity that is most prominent in the vermis. In the brainstem, the central tegmental tracts of the pons are involved early. MR spectroscopy of involved white matter shows decreased NAA, choline, and creatine and elevation of lactate and glucose. Early in the course, NAA/Cr and myo-inositol/Cr ratios are decreased.50,51
Huntington disease is a progressive degenerative disease of the CNS. There is an autosomal dominant pattern of inheritance and high penetrance. This disorder is caused by expansion of a CGA triplet in the IT15 gene (also called HD) of chromosome 4p16.3. This gene encodes huntingtin, a protein of unknown function. The increased number of CAG repeats causes an elongated huntingtin protein with 40 to 150 glutamine residues. The pathogenesis of Huntington disease apparently involves cleavage of the mutant huntingtin protein into fragments that retain the increased number of glutamine residues. Aberrant processing of proteins, including huntingtin, occurs and components are transported to the nucleus where they form intranuclear inclusions. This process eventually leads to cell death.52
The characteristic clinical features of Huntington disease are progressive chorea and dementia. The pathogenesis involves severe neuronal loss, initially in the neostriatum and later in the cerebral cortex. The histological hallmarks of brain damage in Huntington disease are neuronal loss, increased astroglia, and increased oligodendrocytes in the neostriatum. Clinical presentation during childhood is uncommon; approximately 5% of Huntington disease patients have symptoms prior to the age of 14 years.
Neuroimaging studies of patients with Huntington disease reveal loss of volume within the caudate nuclei and putamina. Atrophy of the caudate heads causes characteristic enlargement and rounding of the frontal horns (Figure 18-9). There is progressive generalized atrophy as well. Diffusion-weighted MR imaging shows increased apparent diffusion coefficients in the caudate nuclei and whole brain. The magnitude of these findings correlates with the clinical severity of the disease.53
The mitochondrial diseases constitute a heterogeneous group of genetic disorders in which there is a deficiency in the respiratory electron transport cascade (Table 18-4). Because of a high dependence on oxidative energy metabolism, muscle and brain are usually the sites of greatest clinical alteration in these patients. Although there is great clinical heterogeneity in the mitochondrial disorders, most patients suffer muscle weakness, lactic acidosis, and various CNS deficits. The spectrum of neurological manifestations includes seizures, developmental delay, stroke, ataxia, dementia, optic neuropathy, sensorineural hearing loss, and peripheral neuropathy. There is involvement of other organ systems to a variable extent, potentially leading to manifestations of cardiac dysfunction, renal disease, GI disease, and endocrine abnormalities. Leigh syndrome, Alpers syndrome, and Menkes disease usually present in infancy or early childhood. Mitochondrial encephalopathy with lactic acidosis and stroke-like episodes (MELAS) syndrome, myoclonic epilepsy with ragged red fibers (MERFF) syndrome, and Kearns-Sayre syndrome tend to present later in childhood or in the early adult years.
Disorder | Predominantly affected structures |
---|---|
MELAS | Basal ganglia; parietal and occipital cortex |
Leigh syndrome | Basal ganglia, thalami, brainstem |
Alpers disease | Diffuse atrophy |
MERRF | Basal ganglia |
Menkes disease | Diffuse atrophy |
Glutaric aciduria type I | Basal ganglia; white matter atrophy; enlarged sylvian fissures |
Glutaric aciduria type II | Basal ganglia, cerebral white matter |
Mitochondrial neurogastrointestinal encephalomyopathy | Diffuse leukoencephalopathy |
Kearns-Sayre syndrome | Subcortical cerebral white matter, brainstem, globus pallidus, thalamus |
The diagnosis and classification of the mitochondrial disorders are complex. There is inconsistency in the relationship between genotype and phenotype. Individuals with similar mitochondrial biochemical defects can have clinical manifestations that fit with different syndromes. Likewise, each of the mitochondrial syndromes is associated with various biochemical defects. Mitochondrial DNA alterations responsible for these disorders include single or multiple deletions, duplications, and point mutations. Defective nuclear DNA is sometimes present.
Complex I, the first step in the electron transport chain, is the most common site for mitochondrial abnormalities. About one-third of the respiratory chain deficiencies involve complex I. Often presenting at birth or in early childhood, Complex I deficiency is usually a progressive neurodegenerative disorder that presents early in infancy. Specific mitochondrial disorders that are associated with Complex I deficiency include MELAS, MERRF, and Leigh syndrome. Complex II deficiency can cause Leigh syndrome and encephalomyopathy. A fatal infantile encephalomyopathy occurs in some patients with a complex III deficiency. Others have an encephalomyopathy of later onset. Ragged-red fibers on muscle biopsy are common. The onset of encephalomyopathy in patients with complex IV deficiency (cytochrome c oxidase deficiency) usually occurs during the later part of infancy. Some patients with this deficiency have the clinical constellation of Leigh syndrome.
MR and MR spectroscopy are essential for the diagnosis and monitoring of the mitochondrial disorders. There is considerable heterogeneity in the neuroimaging findings. Most mitochondrial diseases involve gray matter early in the course, usually with greater changes in the deep cerebral nuclei than in the cortical gray matter, due to the greater dependence on oxidative metabolism in the former (Figure 18-10). Brainstem involvement is common. Mitochondrial diseases that sometimes have substantial cerebral white matter involvement include Leigh syndrome, Kearns-Sayre syndrome, and MELAS syndrome. Proton MR spectroscopy demonstrates elevation in lactate during the active phase of the process.54–56
Leigh syndrome (Leigh disease; subacute necrotizing encephalomyelopathy) is a genetically heterogeneous mitochondrial disorder that causes progressive neurodegeneration. There is an autosomal recessive pattern of inheritance in most patients. X-linked (e.g., PDHA1 gene) patterns also occur; maternal inheritance of mitochondrial proteins appears to be important in some forms. This degenerative disorder is associated with focal bilateral destructive lesions of gray and white matter in the brain and spinal cord. The common biochemical feature is defective terminal oxidative metabolism that impairs energy production within mitochondria. Most patients with Leigh syndrome have mutations in mitochondrial DNA or deficient function of pyruvate dehydrogenase. Cytochrome oxidase deficiency is responsible for some cases of Leigh syndrome; mutation of the SURF1 gene is the most common cause of this type.57,58
Although the clinical onset can occur at nearly any age, most patients with Leigh disease present between the ages of 3 months and 2 years. Common presenting features include dysphagia, poor head control, loss of motor skills, irritability, and seizures. Clinical progression is usually rapid, with worsening generalized weakness and hypotonia. Episodes of lactic acidosis can lead to functional impairment of the lungs and kidneys. Manifestations of myocardial involvement can also occur. Progressive neurodegeneration leads to respiratory failure and death early during childhood for most patients with Leigh syndrome. Rarely, the clinical presentation of the disease is during adolescence or early adulthood; progression in these patients is slower than is typical for infantile onset disease.
The biochemical defects of Leigh syndrome lead to microcystic cavitation, neuronal loss, myelin sheath disruption, and small vessel proliferation in portions of the brain and spinal cord. The brainstem, hypothalamus, thalami, lenticular nuclei, caudate nuclei, and posterior columns of the spinal cord are usually involved. Typically, the regions of most marked destruction are the globi pallidi, red nuclei, substantia nigra, and dorsal tegmentum of the midbrain and pons. In the late stages of the disease, hemispheric gray and white matter may be involved, leading to cortical atrophy.
The demyelination and brain destruction of Leigh syndrome are associated with abnormal hypoattenuation on CT and hyperintensity on T2-weighted MR. Classically, the findings are most prominent within the basal ganglia, particularly the putamina. In the acute phase of the process, there may be slight contrast enhancement at the borders of the CNS lesions. As necrosis and atrophy ensue, the lesions become smaller and better defined on CT and standard MR (Figure 18-11). Impaired mitochondrial function during the acute phase leads to reduced water motion on diffusion-weighted images, whereas destroyed brain has increased water motion (i.e., high signal intensity on ADC map images); both patterns can coexist. MR spectroscopy during the acute phase usually demonstrates elevated lactate (due to anaerobic glycolysis), elevated alanine, and diminished NAA; lactate elevation is a useful characterizing feature of Leigh syndrome. Early in the course of Leigh syndrome, abnormalities in the lentiform nuclei on MR spectroscopy can precede alterations on conventional MR images. Infants and young children with Leigh syndrome often have MR findings of delayed myelination. In the late phase of Leigh syndrome, cerebral atrophy and ex vacuo ventriculomegaly develop.59,60
Figure 18–11
Leigh syndrome.
A. A contrast-enhanced CT image of a 12-month-old boy shows hypoattenuation of the caudate nuclei and putamina. B. These structures are markedly hyperintense on a T2-weighted MR image. C. Two years later, there is interval volume loss of the hyperintense basal ganglia. Generalized cerebral atrophy is now present as well. There are areas of abnormal hyperintensity in bifrontal cortical gray matter.


The imaging features of Leigh syndrome vary somewhat with the specific biochemical abnormality. With pyruvate dehydrogenase deficiency, the basal ganglia are most prominently involved (Figure 18-12). The E1 alpha subunit deficiency subtype of pyruvate dehydrogenase deficiency is an X-linked disorder that often includes cerebral dysgenesis (dysgenesis of the corpus callosum, cerebellar dentate nuclei dysplasia, cerebral subcortical heterotopia, polymicrogyria, absent medullary pyramids, and ectopic olivary nuclei) in addition to the classic findings of Leigh syndrome. Cytochrome oxidase deficiency often results in a characteristic pattern of brainstem involvement: T2 hyperintensity and restricted diffusion in the subthalamic nuclei, periaqueductal gray matter, central tegmental tract and reticular formation of the pons, inferior olivary nuclei, and inferior cerebellar peduncles. Mutations of the adenosine triphosphatase 6 gene (i.e., complex V deficiency) are associated with predominant involvement of the globi pallidi, dorsal portions of the mesencephalon and pons, and the anterior aspects of the putamina.61
Figure 18–12
Leigh syndrome due to pyruvate dehydrogenase deficiency.
This 10-month-old infant presented with developmental delay, hypotonia and microcephaly. A. There is abnormal increased signal intensity in the globi pallidi on this T2-weighted MR image. B, C. Diffusion-weighted (B) and ADC (C) images demonstrate markedly restricted diffusion in the globi pallidi.
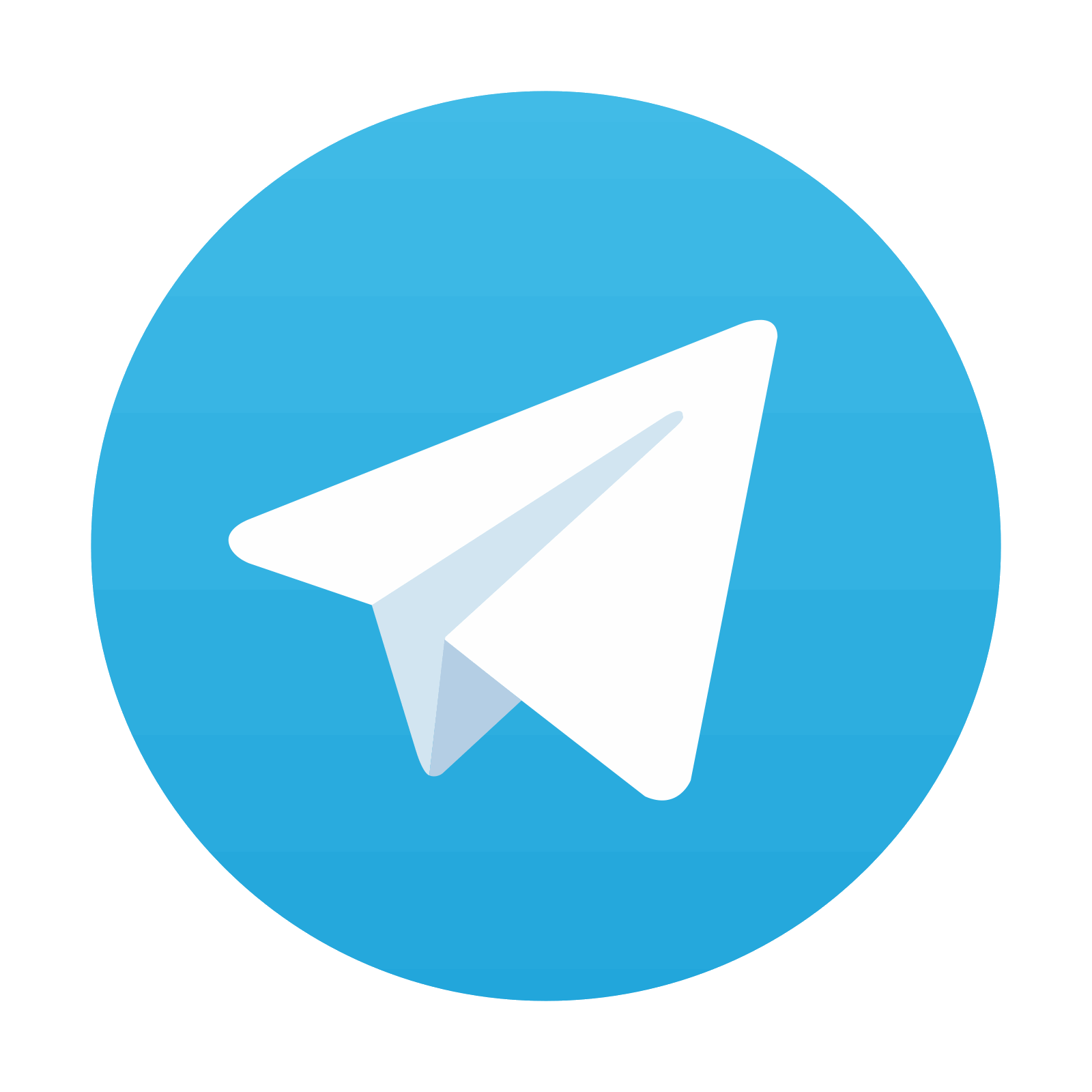
Stay updated, free articles. Join our Telegram channel
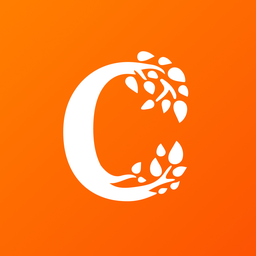
Full access? Get Clinical Tree
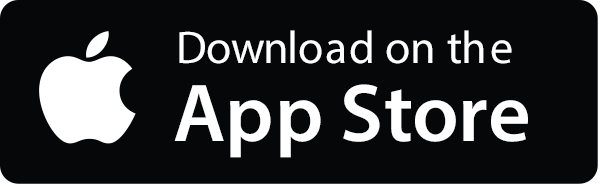
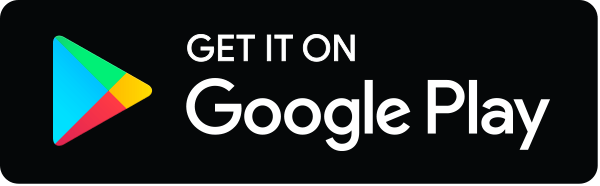
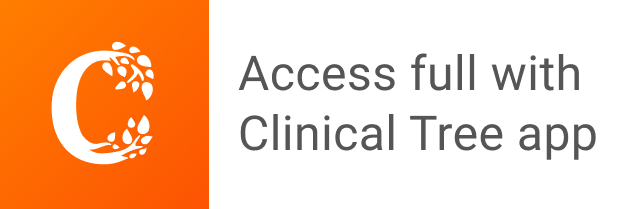