INTRODUCTION
For the vast majority of people who develop epilepsy, initial therapy consists of pharmacologic treatment with one or more of the available antiseizure drugs (ASDs). These medications include the first-generation ASDs like phenytoin (PHT), carbamazepine (CBZ), valproate (VPA), barbiturates such as phenobarbital (PB), certain benzodiazepines (BZDs), and ethosuximide (ESM). The second-generation ASDs include felbamate (FBM), lamotrigine (LTG), gabapentin (GBP), topiramate (TPM), tiagabine (TGB), vigabatrin (VGB), oxcarbazepine (OCBZ), zonisamide (ZNS), levetiracetam (LEV), and pregabalin (PGB). The third-generation ASDs are made up of perampanel (PER), lacosamide (LCM), and retigabine/ezogabine (RGB/EZG). Unfortunately for some patients, complete seizure control, even with this large number of mechanistically diverse ASDs, may never be achieved, even at doses that result in various types and severities of ASD-related adverse effects. This chapter briefly reviews the preclinical animal models that led to the initial identification of the clinically available ASDs and their proposed molecular mechanisms of action.
Strategies for Antiepileptic Drug Discovery
The process by which new ASDs are discovered has evolved in the almost eight decades since phenytoin was identified by Putnam and Merritt (1). The strategies employed in the search for new ASDs are varied but for the most part have been based largely on three different approaches: (a) random drug screening and efficacy-based ASD discovery; (b) rational drug design wherein structural modifications of an active pharmacophore are synthesized and tested; and (c) mechanistic-based ASD development. All three approaches have led to the successful identification of clinically effective drugs. Unfortunately, even with the introduction of almost 20 new ASDs since 1993 there continues to be a significant need for more efficacious and better-tolerated ASDs. Regardless of the process by which a chemical entity is brought forth from the medicinal chemistry laboratory, it must first demonstrate some degree of efficacy in an animal model prior to becoming a candidate for clinical trials.
In Vivo Testing
No single laboratory test will, in itself, establish the presence or absence of antiseizure activity or fully predict the clinical utility of an investigational drug. Thus, the true test of a drug’s efficacy must always await the results of clinical trials. However, there are many available animal seizure models that have been described over the years to possess the appropriate properties to qualify as a predictive seizure model. Historically, the maximal electroshock (MES) test, the subcutaneous pentylenetetrazol (sc PTZ) test, and the hippocampal kindling model represent the three in vivo systems that have been most commonly employed in the search for new ASDs (2). Today, newer in vivo models—for example, in mice or zebra fish—are being introduced that incorporate known genetic defects that more closely resemble the human condition. In addition, the 6 Hz psychomotor seizure model has proven useful in identifying compounds that are effective against partial seizures. In particular, LEV, which is inactive in the MES and sc PTZ models, was found to be quite effective against seizures induced following corneal stimulation at 6 Hz (3). Finally, models such as the corneal kindled mouse, the lamotrigine-resistant kindled rat, and status epilepticus models that result in spontaneous recurrent seizures are being used to investigate the ability of novel and established ASDs to suppress seizure activity.
To gain a full appreciation for a new ASD’s overall spectrum of activity (i.e., narrow vs. broad spectrum), it should be screened in a variety of different seizure and epilepsy models. The reader is directed to several recent reviews pertinent to this particular topic for further discussion (4–7).
Mechanism of Action: General Considerations
It is important to note that the mechanisms of action of currently marketed ASDs are not fully understood. Ultimately, there are numerous mechanisms through which drugs can alter neuronal excitability and thereby limit or control seizure activity. However, as summarized in Table 40.1, the available ASDs appear to target one or more primary mechanisms known to contribute to ictogenesis and spread of seizure activity. For example, drugs that block sustained high-frequency firing through an effect on voltage-sensitive sodium channels can disrupt burst firing, drugs that enhance GABA-mediated neurotransmission can elevate seizure threshold, and drugs that reduce voltage-dependent low-threshold (T-type) calcium currents in thalamocortical neurons can interrupt the thalamic oscillatory firing patterns associated with absence seizures. Likewise, drugs that reduce glutamatergic-mediated excitation can be expected to reduce burst firing elicited by synaptic stimulation. Importantly, many of the available ASDs have been found to exert a number of different pharmacologic actions that likely account for their antiseizure action. In those cases where multiple actions have been defined, it is highly likely that the separate mechanisms offer some degree of synergy.
As shown in Table 40.1, the modulation of voltage-sensitive Na+, Ca2+, and K+ channels; augmentation of GABA-mediated inhibition; inhibition of glutamate-mediated excitatory neurotransmission; and interaction with synaptic vesicle proteins (that is, SV2A) can produce both synaptic and nonsynaptic effects that, when translated to an in vivo effect, is likely to contribute to a drug’s antiseizure action. Unfortunately, the ability of an ASD to modulate the function of any one of these neuronal processes can have profound consequences on both the abnormal firing of epileptic neurons as well as normal neuronal communication. As a result, the same action(s) that decreases seizure frequency are likely to contribute to a drug’s central nervous system (CNS)-related adverse effect profile. It is relevant to note that some of the drugs display certain properties that lead to a greater separation between effective and toxic effects. For example, it is widely accepted that to be a therapeutically useful Na+ channel blocker, drugs should possess both voltage- and frequency-dependent actions. These properties confer a certain level of selectivity toward those neurons that display a paroxysmal depolarization shift and high-frequency sustained repetitive firing within a seizure focus. Thus, at therapeutic concentrations, voltage- and use-dependent Na+ channel blockers would be less likely to affect normal neuronal function than a Na+ channel blocker that does not display these properties. One still needs to reconcile the cognitive impairment that has come to be associated with the voltage- and use-dependent Na+ channel blockers PHT and CBZ. This can be done in part by acknowledging the fact that these drugs can, at higher concentrations, attenuate Na+ currents at resting membrane potentials and thereby modify normal neuronal communication. Furthermore, inhibiting neuronal voltage-sensitive Na+ channels can produce a subsequent inhibition of depolarization-dependent neurotransmitter release. Given the critical role that the excitatory neurotransmitter glutamate plays in fast excitatory neurotransmission, slight modification of glutamate release in the absence of abnormal neuronal firing may contribute to the cognitive impairment observed in some patients taking these drugs. In this case, drugs that display greater voltage and use dependence would necessarily offer certain potential advantages over less selective Na+ channel blockers. As more and more is learned about the role of the various molecular subunits that comprise most receptor- and voltage-gated ion channels, it may be possible to further increase the therapeutic index of a given class of ASDs by developing subunit-selective drugs.
TABLE 40.1
The remainder of this chapter will focus on the most widely accepted mechanism(s) that have been observed at therapeutically relevant concentrations of the first-, second-, and third-generation ASDs. As mentioned previously this is not to imply that a minor, less-established action does not contribute to a drug’s antiseizure profile. The reader is referred to reviews by Rogawski and Porter (8), Macdonald and Meldrum (9), Meldrum (10), and White (11) for a more comprehensive discussion of these effects.
FIRST-GENERATION (PRE-1993) ASDS
Phenytoin (PHT) and Carbamazepine (CBZ)
The antiseizure profiles of PHT and CBZ observed in animal seizure models correlate well with their clinical efficacy in generalized tonic–clonic and complex partial seizures (Table 40.1). Thus, both drugs are effective against tonic extension seizures induced by a number of different stimuli including MES and various chemoconvulsants (12,13), and both ASDs are effective against fully expressed kindled seizures (8). Unlike ESM, both drugs are ineffective against clonic seizures induced by PTZ. Studies conducted to date provide compelling evidence that therapeutic concentrations of PHT and CBZ prevent sustained repetitive firing resulting from extended depolarization (Table 40.2). In addition, both drugs prevent post-tetanic potentiation (PTP), a process whereby high-frequency stimulation produces a transiently enhanced responsiveness to subsequent stimulation. The ability of PHT and CBZ to block PTP may explain, in part, their ability to limit seizure spread.
The voltage-sensitive Na+ channel is thought to underlie the ability of neurons to fire repetitively. As such, ASDs that inhibit sustained repetitive firing are likely to exert an effect on voltage-sensitive Na+ channels. PHT and CBZ have been found to exert an inhibitory effect on voltage-gated Na+ channels (14–16) that is both use- and voltage-dependent (Table 40.2). These two properties account for the unique ability of PHT, CBZ, and other voltage-dependent Na+ channel blockers to limit high-frequency firing characteristic of epileptic discharges without significantly altering normal patterns of neuronal firing.
PHT and CBZ have also been demonstrated to produce a shift in the steady-state inactivation curve in mammalian myelinated nerve fibers to more negative voltages (17), thereby effectively reducing the degree of depolarization required to inactivate Na+ channels. In addition, both drugs delayed the rate of Na+ channel recovery from inactivation. Whether slight differences between PHT and CBZ in time dependence of the frequency-dependent block account for differences in efficacy between these drugs has yet to be established. Thus, by stabilizing the Na+ channel in its inactive form and slowing its rate of recovery from inactivation, both drugs can prevent sustained repetitive firing evoked by prolonged depolarization such as that found in an epileptic focus.
Similarly, voltage-, frequency-, and time-dependent inactivation of Na+ channels by PHT has also been confirmed in isolated rat hippocampal neurons and Xenopus oocytes injected with human brain mRNA (18,19). All of these studies provide strong experimental evidence supporting an interaction of PHT and CBZ with the voltage-dependent Na+ channel.
Ethosuximide (ESM)
Unlike PHT and CBZ, ESM is effective against clonic seizures induced by subcutaneously administered PTZ (Table 40.1). It is also active against spike-wave seizures induced by the chemoconvulsant γ-hydroxybutyrate (20), and against spontaneous spike-wave discharges in the lh/lh mouse model of absence (21). ESM is ineffective against tonic extension seizures induced by MES and focal seizures in the kindled rat (22). In this respect, ESM’s in vivo profile is consistent with its clinical efficacy against generalized absence seizures and lack of efficacy in generalized tonic–clonic or partial seizures (Table 40.1).
TABLE 40.2
The mechanism of ESM was not elucidated until 1989, when it was shown to reduce low-threshold T-type Ca2+ currents in thalamic neurons isolated from rats and guinea pigs (23). Reduction of the T-type current was voltage-dependent and was observed at clinically relevant ESM concentrations, suggesting that this mechanism may be the basis for efficacy of ESM in controlling absence seizures (Table 40.2). This effect of ESM, which is produced at clinically relevant concentrations, is thought to represent the primary mechanism by which it controls absence epilepsy. Activation of T-channels in thalamic relay neurons generates low-threshold Ca2+ spikes that are thought to contribute to the abnormal thalamocortical rhythmicity that underlies the 3-Hz spike-and-wave EEG discharge of absence epilepsy. By preventing Ca2+-dependent depolarization of thalamocortical neurons, ESM, and dimethadione, the active metabolite of the antiabsence drug trimethadione (24) is thought to block the synchronized firing associated with spike-wave discharges.
Valproic Acid
Of the standard ASDs, valproic acid (VPA) appears to have the broadest preclinical and clinical profile (Table 40.1). It is active in a variety of animal seizure models, including electrical seizures in the MES test, chemically induced clonic seizures in the sc PTZ test, electrically kindled seizures in the rat and mouse (25), GBL-induced spike-wave, and spontaneous spike-wave discharges in the lh/lh mouse (20–22). Clinically, VPA is useful in the management of both partial and primary generalized seizures.
Based on VPA’s rather broad preclinical and clinical antiseizure profile, it might be anticipated that VPA would possess more than one mechanism of action. Indeed, a number of studies suggest that VPA possesses at least three different mechanisms of action. First, in vitro studies with VPA support an action at the voltage-sensitive Na+ channel (11). For example, VPA has been found to inhibit Na+ currents in isolated Xenopus leavis myelinated nerves (26) and in neocortical neurons in vitro (27). Furthermore, in rat hippocampal neurons VPA decreased peak Na+ currents in a voltage-dependent manner and produced a 10 mV leftward shift in the Na+ inactivation curve (28). Such an action may contribute to its ability to prevent MES-induced tonic extension in animals and generalized tonic–clonic and partial seizures in humans. Secondly, VPA, like ESM, has been shown to reduce T-type Ca2+ currents in primary afferent neurons (29). This effect, albeit modest and observed at high VPA concentrations, may contribute to VPA’s clinical efficacy in absence seizures. Lastly, VPA-mediated elevations of whole brain GABA levels and potentiation of GABA responses are also found at relatively high drug concentrations (see ref. [8] for review). This effect, coupled with its effect at the voltage-sensitive Na+ channel, may also contribute to its efficacy against kindled seizures in mice and rats and human partial seizures.
Benzodiazepines and Barbiturates
In animal seizure models, the benzodiazepines (BZDs) and barbiturates are effective at low doses against sc PTZ-induced clonic seizures and in the kindled rat model of partial seizures. The barbiturates at low doses and BZDs at higher doses are also active against MES-induced tonic extension. One important distinction between these two classes of compounds lies in their efficacy against spike-wave seizures in the GBL and lh/lh models of absence. In both models, the BZDs are effective in reducing spike-wave discharges, whereas the barbiturates actually worsen spike-wave discharges (20,21).
Once released from GABAergic nerve terminals, the inhibitory neurotransmitter GABA binds to both GABAA and GABAB receptors. The GABAA receptor complex is a multimeric macromolecular protein that forms a chloride-selective ion pore. Thus far, multiple binding sites for GABA, BZDs, barbiturates, neurosteroids, convulsant β-carbolines, and the chemoconvulsant picrotoxin have been identified (30). The GABAB receptor is coupled via a GTP-binding protein to calcium or potassium channels but does not form an ion pore and does not appear to contribute to the antiseizure action of either BZDs or barbiturates. The principle antiseizure action of the BZDs and barbiturates is thought to be related to their ability to enhance inhibitory neurotransmission by allosterically modulating the GABAA receptor complex.
GABA receptor current can be enhanced by increasing channel conductance, open and burst frequency, and/or open and burst duration. Studies indicate that the barbiturates act mainly by increasing the mean channel open duration without affecting channel conductances or opening frequency, whereas the binding of a BZD to its allosterically coupled GABAA-binding site increases opening frequency without affecting open or burst duration (31–34). Results from several reconstitution experiments conducted in a variety of laboratories wherein specific GABA receptor subunits were transiently expressed in either Xenopus oocytes, CHO cells, or human embryonic kidney cells have suggested a molecular basis for the differential regulation of GABA receptor current by these two classes of drugs. The results from these studies have suggested that the allosteric regulatory site conferring barbiturate sensitivity appears to be contained in the α and β subunits (35,36). Thus, while GABA receptors formed from α1β1 subunits are barbiturate-sensitive, they are BZD-insensitive (35,37). BZD sensitivity is restored when the γ2 subunit is coexpressed with α1 and β1 subunits (37). Transient coexpression of the γ2, α1, and β1 subunits in human embryonic kidney cells results in fully functional GABA receptors that are sensitive to the BZDs, barbiturates, β-carbolines, and picrotoxin. These effects at the GABAA receptor are likely to account for the efficacy of the BZDs and barbiturates against kindled seizures in rats and partial seizures in humans.
One important difference between these two classes of GABA modulators is that the barbiturates will directly activate a Cl– current in the absence of GABA, whereas the BZDs will not. This difference, coupled with possible anatomical differences in subunit expression, may explain in part why BZDs attenuate spike-wave seizures in both rodents and humans, whereas the barbiturates actually exacerbate spike-wave discharges (38). For example, clonazepam and diazepam selectively augment GABAA-mediated inhibition in neurons of the nucleus reticularis thalami (NRT), but not other thalamic neurons. Because the inhibitory synapses in the NRT are primarily reciprocal inhibitory circuits, augmentation of inhibition by the BZDs results in decreased output of NRT onto the thalamus. By decreasing the “pacemaker” activity of the thalamic reticular neurons impinging onto the thalamus, the BZDs selectively and effectively prevent spike-wave discharges. In contrast, barbiturates, by increasing the inhibitory drive within the thalamus, enhance the deinactivation of T-currents, which results in a stronger low threshold burst and increased thalamocortical rhythms. In contrast to the barbiturates, the BZDs do not augment inhibition within the thalamus and, thus, do not display the same proconvulsant action.
SECOND-GENERATION (1993–2001) ASDS
In some circumstances, the mechanisms of action of those ASDs introduced between 1993 and 2001 display a marked overlap with those defined previously for the pre-1993 ASDs. However, some of these compounds display a unique mechanistic profile relative to the older drugs. Increasingly, ASD development has produced novel molecules that enhance inhibitory neurotransmission by acting on GABA receptors, GABA transporters, and GABA metabolism or reduce excitatory neurotransmission mediated by glutamate.
Felbamate
Felbamate (2-phenyl-1,3-propanediol dicarbamate; FBM) received FDA approval in mid-1993 and was the first new ASD approved in the United States since 1978. Results from preclinical studies conducted by the NINDS Anticonvulant Drug Development Program demonstrated that FBM possessed a broad antiseizure profile in animal seizure models (Table 40.1) (39,40). It is effective against tonic extension seizures induced by MES and the glutamate agonists NMDA and quisqualic acid. Like VPA, FBM is also active against clonic seizures induced by a number of chemoconvulsants. In animal models of partial epilepsy, FBM has been found to reduce the seizure severity in corneal kindled rats and PTZ-kindled rats and to raise the seizure threshold in amygdala-kindled rats (41,42). In addition to its antiseizure properties, FBM has also been demonstrated to possess neuroprotectant properties both in vitro and in vivo (43–46). Consistent with FBM’s broad preclinical profile is its rather broad clinical spectrum. Upon entry into the U.S. market, FBM was approved for treatment of partial seizures, primary and secondary generalized tonic–clonic seizures, and Lennox–Gastaut syndrome.
FBM also possesses a broad mechanistic profile (see ref. [11] for review and references), which provides a basis for understanding its broad preclinical and clinical antiseizure profile, as well as its neuroprotectant action (11). FBM reduces sustained repetitive firing in mouse spinal cord neurons in a concentration-dependent manner. This action suggests an interaction with voltage-dependent Na+ channels, an effect that was confirmed in rat striatal neurons (Table 40.2) (47). FBM, at low concentrations, also appears to inhibit dihydropyridine-sensitive high-threshold voltage-sensitive Ca2+ currents (48), an effect that is consistent with decreased excitability. One mechanism that appears to be unique to FBM is related to its ability to modulate glutamate receptor function through an action at the strychnine-insensitive glycine site of the NMDA receptor. FBM has been shown to displace a competitive antagonist at the strychnine-insensitive glycine binding site of the NMDA receptor in rat brain membranes (47) and postmortem human brains (48). Furthermore, FBM exerts a neuroprotective effect in the rat hippocampal slice that is reversed by glycine (49). Likewise, its antiseizure effect is reversed by strychnine-insensitive glycine receptor agonists (50–52). In addition, FBM has been found to directly inhibit NMDA-evoked currents (53). FBM’s ability to block NMDA-evoked currents is unique among both the standard and newer ASDs. Despite its interaction with this receptor complex, FBM does not appear to produce either the behavioral or pathologic impairment of the CNS that has been associated with either competitive or noncompetitive NMDA antagonists (39).
At substantially higher concentrations, FBM has also been reported to enhance GABA-evoked chloride currents (53). The mechanism by which FBM enhances GABA-evoked currents is unknown. For example, FBM in concentrations up to 1 mM does not appear to affect ligand binding to the GABA-, BZD-, or picrotoxin-bindingsites on the GABAA receptor ionophore, nor does it enhance GABA-stimulated 36Cl– flux into cultured mouse spinal cord neurons (54).
FBM remains a mechanistically very interesting ASD with an apparently broad antiseizure profile. However, its clinical utility has been markedly limited by the serious hematologic and hepatic toxicity reported after its commercialization in the United States (55). A thorough understanding of the mechanisms underlying these idiosyncratic adverse effects and the ability to identify patients at risk will likely lead to an increased use of this highly effective drug in therapy-resistant patients.
Gabapentin
The second of the newer generation ASDs to be marketed in the United States is gabapentin (GBP). GBP, 1-(aminomethyl)cyclohexaneacetic acid, was originally designed and synthesized as a drug to enhance GABA-mediated inhibition by mimicking the steric conformation of the endogenous neurotransmitter GABA (56). Thus, GBP is one of the few examples where rational drug design resulted in a potent ASD.
In animal seizure models, GBP is active in a number of antiseizure tests (57–60). It reduces electrically and chemically induced tonic extension seizures, as well as clonic seizures induced by sc PTZ (Table 40.1), and blocks sound-induced seizures in DBA mice but not absence-like spike-wave seizures. GBP is also effective against fully kindled seizures in the kindled rat. These findings support its clinical efficacy against human partial seizures and secondarily generalized seizures.
Although the chemical structure and steric conformation of GBP were originally designed to enhance GABA-mediated inhibition, GABA mimetic activity was surprisingly absent in early studies. Despite demonstrated efficacy in both animal and human studies and numerous in vitro studies that have described several potential mechanisms of action, the precise mode of action of GBP remains unknown.
Findings from in vitro studies suggest that GBP can increase the concentration of GABA in both the glial and neuronal compartments (see ref. [61] for review and references). GBP has also been shown to increase in vivo occipital lobe GABA levels in patients with epilepsy (Table 40.2) (62). GBP may increase brain GABA turnover by interacting with a number of different metabolic processes. It has been demonstrated to enhance glutamate dehydrogenase and glutamic acid decarboxylase and inhibit branched-chain amino acid aminotransferase and GABA aminotransferase. Although any one of these effects could singly, or in concert with each other, contribute to the antiseizure action of GBP, it is not clear at this point which effects are important (58,61). After prolonged administration, a voltage- and frequency-dependent limitation of Na+– dependent sustained action potential firing in mouse cortical neurons was observed at clinically relevant concentrations of GBP (63). GBP’s ability to limit sodium-dependent sustained action potential firing in cultured mouse spinal cord neurons was voltage- and frequency-dependent. The precise mechanism of this effect is not known; however, it is unlikely that GBP inhibits sodium currents in a manner similar to that of established sodium channel blockers PHT and CBZ. The delayed effect of GBP against sustained repetitive firing is consistent with the substantial time lag between the appearance of peak plasma and brain concentrations and GBP’s time to peak antiseizure effect observed following i.v. administration (64). This delay in antiseizure effect suggests that prolonged synaptic and/or cytosolic exposure to GBP is important and supports an indirect mechanism of action for GBP.
GBP has also been reported to bind to a novel site in a rat brain that is not affected by any of the standard ASDs and is not significantly displaced by NMDA or AMPA (a-amino-2,3-dihydro-5-methyl-3-oxo-4-isoxazolepropanoic acid) receptor ligands (58). GBP is displaced stereospecifically by certain L-amino acids, demonstrating a relationship between the GBP binding site and system-L transporter membrane. GBP has also been found to bind to the α2δ regulatory subunit of the voltage-sensitive Ca2+ channel (65). The precise function of this auxiliary subunit is not known, but it has been suggested that GBP may modify monoamine neurotransmitter release through its interaction with Ca2+ channels (see ref. [60] for review and references).
In summary, GBP displays a unique antiseizure profile in animal studies and has demonstrated efficacy in human trials. Furthermore, results from a number of in vitro and in vivo studies would also suggest that the mechanism of action of GBP is unique among the existing ASDs. The two that appear most closely associated with its antiseizure action are its ability (a) to enhance GABA turnover and release and (b) to interact with the α2δ regulatory subunit of the voltage-sensitive Ca2+ channel.
Lamotrigine
Lamotrigine (3,5-diamino-6-(2,3-dichlorphenyo)-1,2,4-triazine; LTG) was the third new generation ASD to be marketed in the United States since 1993. LTG was derived from an antifolate drug development program based on the observation that chronic use of phenobarbital, primidone, and phenytoin reduced folate levels (66) and that folates induce seizures in laboratory animals (67). However, despite its structural similarity to other antifolate drugs, LTG displays only weak antifolate activity. Furthermore, results from structure–activity studies suggest that there is little correlation between antifolate activity and antiseizure potency (8).
In some respects, the preclinical profile of LTG is very similar to that observed with PHT and CBZ (Table 40.1). For example, LTG is active in the kindled rat and corneal kindled mouse and against tonic extension seizures in the MES test but is ineffective against sc PTZ-induced clonus (24,59,68,69). However, the preclinical profile of LTG differs significantly from that of PHT and CBZ in one important manner. LTG is effective in the lh/lh model of absence (60), whereas PHT and CBZ are not only ineffective, but can, upon occasion, actually worsen spike-wave seizures (Table 40.1) (19,20). In this respect, the preclinical profile of LTG supports its clinical utility against partial seizures, generalized tonic–clonic, and generalized absence seizures (70–72).
In in vitro experiments, LTG selectively blocks veratridine-evoked but not potassium-evoked release of endogenous glutamate (73). These findings suggested that LTG, like PHT, acts at voltage-sensitive Na+ channels to stabilize neuronal membranes (Table 40.2). Evidence favoring this mechanism includes the documented capacity of LTG to inhibit {3H}batrachotoxin binding and veratrine-stimulated {14C}guanidinium transport into synaptosomes (74,75), its capacity to inhibit sustained repetitive firing (74), and its demonstrated concentration-dependent inhibition of Na+ currents in mouse neuroblastoma cells (15). The effects of all three ASDs on Na+ currents were voltage-dependent and all three slowed recovery from inactivation.
However, as mentioned previously, the clinical profile of LTG appears broader than would be expected based on this single mechanism. Given the lack of efficacy of other Na+ channel blockers against generalized absence seizures, LTG’s efficacy against this seizure type is likely unrelated to this mechanism, unless, as suggested by Coulter (38), LTG exerts an effect on a particular isoform of the brain Na+ channel that is either anatomically involved in the generation of spike-wave discharges or whose expression is selectively altered in the thalamocortical circuitry of absence patients. LTG has been found to decrease voltage-gated Ca2+ currents (see ref. [48] for review and references). This effect may contribute to a decrease in neurotransmitter release and thereby contribute to its antiseizure action (Table 40.3). Additional investigations are required to resolve whether this effect contributes to the broader clinical profile of LTG vs. that of PHT and CBZ.
Levetiracetam
Levetiracetam (LEV): (S)-α-ethyl-e-oxo-pyrrolidine acetamide is the (S)-enantiomer of the ethyl analog of the nootropic drug piracetam. Levetiracetam displays a unique preclinical profile in animal seizure and epilepsy models. For example, it was found to be inactive in the traditional MES and sc PTZ-seizure models but highly active against audiogenic and fully kindled partial seizures (see ref. [76] for review and references). Furthermore, LEV has been found to inhibit pentylenetetrazol-induced spike-wave discharges (77) and spontaneous spike-wave discharges in the GAERS rat (78). In addition, LEV decreases the afterdischarge duration in the amygdala-kindled rat and inhibits bicuculline-induced increase in hippocampal population spike amplitudes (79,80). Levetiracetam has also been found to be uniquely active in the 6-Hz psychomotor seizure model of pharmacoresistance (3). For example, at a current intensity equivalent to two times the current necessary to evoke a seizure, LEV and VPA were the only two ASDs that retained their ability to block 6-Hz seizures, although there was a marked decrease in the potency of both drugs at two times the CC97 (3).
In in vitro studies using rat hippocampal slices, LEV has also been observed to reduce the amplitude and number of repetitive population spikes induced by exposure to high K+-low Ca2+ perfusion medium (81). LEV has also been found to inhibit bicuculline-induced bursts of action potentials and to decrease the frequency of NMDA-evoked bursting in hippocampal pyramidal neurons (82). Despite these observations, a unified molecular mechanism(s) has yet to be clearly defined. This is perhaps not so surprising given LEV’s unique antiseizure profile. LEV has been demonstrated to bind to a specific, saturable, and stereoselective binding site in rat brain membranes, which displays a high density in the hippocampus, cortex, and cerebellum (83). The finding that there is an excellent correlation between LEV binding and antiseizure activity in the audiogenic mouse suggests a possible functional role for the LEV binding site. Of the various actions that might be ascribed to LEV, its ability to modulate neuronal high-voltage Ca2+ currents (Tables 40.1 and 40.2) appears to be the one most likely to contribute to its ability to dampen neuronal hyperexcitability (84). At higher concentrations, LEV has also been found to prevent the negative modulatory effects of Zn2+ and β-carbolines on GABA- and glycine-gated currents (85). These effects would be expected to prolong the hyperpolarization associated with GABA- and glycine-mediated neurotransmission. Finally, the LEV binding site has been found to be highly homologous with a novel site identified as synaptic vesicle protein 2A (SV2A) (86). The precise mechanism by which modulation of SV2A protein contributes to the antiseizure efficacy of LEV is not presently known. SV2A is an abundant protein that is closely associated with synaptic vesicles and is thereby thought to contribute to docking and release of neurotransmitter substances. Thus, it is highly possible that LEV, by binding to the SV2A protein, modifies neurotransmitter release.
Oxcarbazepine
Oxcarbazepine (10,11-dihydro-10-oxo-carbamazepine; OCBZ) is structurally related to CBZ (see ref. [87] for review). The keto substitution at the 10,11 position of the dibenzazepine nucleus does not affect the therapeutic profile of OCBZ but does contribute to better tolerability in humans (88–91). In vivo, OCBZ is rapidly and completely reduced to its active metabolite (10, 11-dihydro-10-hydroxy-carbamazepine; HCBZ), which is thought to be responsible for the antiseizure action of OCBZ (92). HCBZ is a racemate that can be separated into two enantiomers, both of which appear to contribute to the antiseizure activity of HCBZ (93).
TABLE 40.3
FUNCTIONAL CONSEQUENCES OF PROPOSED MECHANISM OF ACTION OF ESTABLISHED AND NEWER AEDs | ||
Molecular Mechanism of Action | AED | Consequences of Action |
Na+ channel blockers | PHT, CBZ, LTG, FBM, OCBZ, TPM, VPA,LCM | 1. Block action potential propagation 2. Stabilize neuronal membranes 3. Decrease neurotransmitter release 4. Decrease focal firing 5. Decrease seizure spread |
Ca2+ channel blockers | ESM, VPA, GBP, LTG, PGB | 1. Decrease neurotransmitter release (N & P types) 2. Decrease slow-depolarization (T-type) 3. Decrease spike-wave discharges |
GABAA receptor allosteric modulators | BZDs, PB, FBM, TPM | 1. Increase membrane hyperpolarization 2. Elevate seizure threshold 3. Attenuate (BZDs) spike-wave discharges 4. Aggravate (barbiturates) spike-wave discharges 5. Decrease focal firing |
GABA uptake inhibitors/GABA-transaminase inhibitors | TGB, VGB | 1. Increase synaptic GABA levels 2. Increase membrane hyperpolarization 3. Decrease focal firing 4. Aggravate spike-wave discharges |
NMDA receptor antagonists | FBM | 1. Decrease slow excitatory neurotransmission 2. Decrease excitatory amino acid neurotoxicity 3. Delay epileptogenesis |
AMPA/kainate receptor antagonists | PB, TPM, PER | 1. Decrease fast excitatory neurotransmission 2. Attenuate focal firing |
Binds to synaptic vesicle protein, SV2A | LEV | 1. Currently unknown |
K+ channel opener | RGB/EZG | 1. Increases spike frequency adaptation 2. Hyperpolarizes membrane potential |
The antiseizure profiles of OCBZ and HCBZ are virtually identical to that of CBZ (Table 40.1). For example, they are both active against tonic-extension seizures induced by MES and essentially inactive against clonic seizures induced by PTZ, picrotoxin, and strychnine (93,94). Both compounds were found to possess activity against focal seizures in monkeys with chronic aluminum foci (92). In comparative clinical trials, OCBZ was demonstrated to be as efficacious and better tolerated than CBZ (89–91). Clinically, OCBZ seems to represent a less toxic and equally efficacious alternative to CBZ that appears to exert its antiseizure effect through a similar mechanism of action. Furthermore, OCBZ appears to be highly efficacious and safe as a first-line treatment in adults with partial and generalized tonic–clonic seizures (95).
OCBZ, HCBZ, and CBZ all appear to share a similar mechanistic profile (Table 40.2; see ref. [96] for review). OCBZ and HCBZ, like other drugs that block MES seizures, both block sustained repetitive firing in cultured spinal cord neurons in a voltage- and frequency-dependent manner (97). Additional results from electrophysiologic studies suggest that HCBZ may mediate some of its antiseizure effect through an action at high-threshold voltage-gated Ca2+ channels (48).
Pregabalin
Pregabalin (S-(+)-3-isobutyl-γ-aminobutyric acid; PGB) was approved for use as adjunctive therapy for the treatment of partial seizures in 2005. PGB is a structural analog of GABA, but, like the similar compound gabapentin, has no activity at either GABAA or GABAB receptors.
The preclinical profile of PGB revealed that it is more potent and bioavailable than gabapentin. In animal models of seizure activity, PGB effectively protects against tonic extension seizures induced by MES, clonic seizures induced by sc PTZ, and audiogenic seizures in the DBA/2 mouse (98). The ability of PGB to block kindled seizures accurately predicted that PGB would be effective against partial seizures. PGB was only found to block bicuculline and strychnine-induced seizures at high concentrations and it was unable to block absence seizures in the GAERS rat.
Although it is known that PGB binds to the α2d auxillary subunit of the voltage-gated calcium channel, it is still not completely clear how it exerts its antiseizure activity. Experiments performed in cultured hippocampal neurons suggest that PGB may reduce spontaneous and evoked neurotransmitter release, most likely by targeting the readily-releasable pool of synaptic vesicles (99).
Topiramate
Topiramate {2,3:4,5-Bis-O-(1-methylethylidene)-β-D-fructopyranose sulfamate; TPM} is a chemically novel ASD. In animal tests, the antiseizure profile of TPM most closely approximates that of PHT, CBZ, and LTG. For example, it is active against MES-induced tonic extension seizures. TPM does not prevent seizures induced by sc PTZ; however, it does elevate the seizure threshold for PTZ-induced seizures (100,101). In the amygdala-kindled rat, TPM reduced both the seizure score and the after discharge duration of fully expressed kindled seizures (102) and with pretreatment appears to delay the acquisition of kindling (103). This latter effect suggests an antiepileptic vs. purely antiseizure effect. In the spontaneously epileptic rat, which displays both tonic extension seizures and absence-like spike-wave discharges, TPM was as effective as PHT in reducing tonic extensions and, like ESM, decreased the duration of spike-wave discharges in a dose- and time-dependent fashion (Table 40.1) (104). Furthermore, topiramate has been shown to be effective in the GAERS model of absence epilepsy (105). These findings support the apparent broad clinical profile that has emerged for TPM. For example, it appears to be effective against a broad range of seizure types, including partial and generalized tonic–clonic seizures (Table 40.1). In addition, TPM is approved for the prophylactic treatment of migraines, a further indication of its broad spectrum activity in the CNS. Anecdotal reports have suggested that TPM may be effective against uncomplicated absence seizures, an action supported by findings in the spontaneously epileptic rat (104) but not the lh/lh mouse (61).
In studies conducted thus far, TPM has been found to possess multiple potential mechanisms of action (see ref. [106,107] for review and references and Table 40.2). In cultured hippocampal neurons, therapeutic concentrations (3–30 µM) of TPM inhibit sustained repetitive firing in a use- and concentration-dependent manner (108) and reduce voltage-activated Na+ currents in cultured neocortical neurons (109).
TPM has also been shown to reduce kainate-evoked inward currents, block kainate-evoked cobalt influx, and block kainic acid receptor-mediated postsynaptic currents in the amygdala, indicating that TPM has antagonistic effects on the kainate/AMPA subtype of glutamate receptor (110–112). The effect of TPM on kainate-evoked currents appears to be unique to TPM among the new ASDs and is consistent with a decrease in neuronal excitability and may, when coupled with its effects against Na+ currents, contribute to its efficacy against partial and generalized convulsive seizures.
Effects at the Na+ channel and AMPA/kainate receptor do not necessarily support the ability of TPM to block absence-like spike-wave discharges in the spontaneously epileptic rat and anecdotal reports suggesting efficacy against generalized absence epilepsy. TPM has been reported to enhance GABA-evoked chloride single channel currents in cultured neocortical neurons (101,113). Kinetic analysis of single-channel recordings from excised outside-out patches demonstrated that TPM increased the frequency of channel opening and the burst frequency but was without effect on open channel duration or burst duration. This effect of TPM on GABAA channel activity was similar to that observed with BZDs; however, the ability of TPM to enhance GABAA-evoked current was not reversed by the BZD antagonist flumazenil. Although consistent with TPM’s ability to block spike-wave discharges and its apparent efficacy against absence epilepsy, enhancement of GABA-mediated inhibition would not be predicted from previous in vitro studies wherein TPM did not displace radiolabelled ligand binding to known binding sites on GABAA receptors (100).
In other preclinical studies, TPM was shown to inhibit certain carbonic anhydrase isoforms, an activity that may through an alteration of HCO3– homeostasis contribute to TPM’s mechanism of action (114). Topiramate has also been reported to reversibly hyperpolarize hippocampal neurons in rat hippocampal slices (115) and to inhibit neuronal repetitive firing in rat olfactory cortical neurons by inducing a slow outward membrane current. Both of these effects are thought to be the result of activating an outward current carried by K+ ions (115,116). Furthermore, TPM has been observed to decrease high-voltage activated Ca2+ currents (HVACC) in CA1 pyramidal cells (117). This effect at both L- and non L-type Ca2+ channels required a short preincubation period.
The results to date suggest that the effects of TPM on Na+ channels, GABAA receptors, HVACC, and AMPA/kainate receptors are unique when compared to prototypical modulators of these processes. For example, TPM’s effects on all four of these protein complexes can be highly variable. TPM has been observed to produce both immediate and delayed effects; sometimes its effect is reversible and sometimes it is not; in some preparations, TPM’s action appears to be dependent on the age of neurons in culture. All of these observations, albeit frustrating, are suggestive of a unique interaction with the target protein that may be dependent in part on its molecular structure Indeed, recent results suggest that the effects of TPM, at least on GABAA receptor function, depend on the expression of specific subunits (118). Clearly, additional studies are required to further elucidate its precise mechanism of action.
Tiagabine
Tiagabine {(R)-N-(4,4-di-(3-methyl-thien-2-yl)but-3-enyl) nipecotic acid hydrochloride; TGB} is a selective GABA uptake inhibitor (Table 40.3), which emerged from a mechanistic-based drug discovery program designed to identify lipophilic GABA uptake inhibitors for the treatment of epilepsy (see ref. [119] for review and references).
In animals, TGB is effective against several types of chemically induced seizures, including DMCM-induced clonic seizures and sc PTZ-induced tonic (potent inhibitor) and clonic (partial inhibitor) seizures (Table 40.1) (119). TGB reduces both seizure severity and afterdischarge duration in the amygdala-kindled rat, was active against audiogenic seizures in DBA/2 mice, and was only partially effective against photically induced myoclonus in the photosensitive baboon. However, it is active in the MES test only at doses 2- to 3-fold higher than that producing motor impairment. TGB, like VGB, also worsens spike-wave discharges in the GAERS, GBL, and lh/lh models of absence (61).
In vitro studies have shown TGB to be a potent inhibitor of neuronal and glial GABA uptake (Table 40.2)(see ref. [119] for review and references). TGB binds selectively and reversibly to the GAT-1 GABA uptake carrier of both glia and neurons but does not stimulate GABA release. It is ineffective at other receptor binding and uptake sites evaluated to date, including the glutamate receptor, Na+, or Ca2+ channels. Inhibition of GABA uptake by TGB leads to increased synaptic concentrations of GABA and a consequent enhancement and prolongation of GABA-mediated inhibitory neurotransmission, an effect that is assumed to be the basis of TGB’s antiseizure activity against partial seizures and its ability to aggravate spike-wave seizures in rodents and potentially humans. Indeed, TGB treatment has been demonstrated to increase extracellular fluid GABA levels in vivo in both animal (120) and human brains (121). The mechanism of its proconvulsive action is probably much like that of VGB in that elevated synaptic concentrations of GABA at the level of the thalamus are thought to potentiate GABAB-mediated slow after-hyperpolarization, which leads to enhanced deinactivation of T-currents and increased amplification of thalamocortical rhythms necessary to support spike-wave discharges.
Vigabatrin
Vigabatrin {4-amino-5-hexenoic acid; (γ-vinyl GABA)} (VGB) is available in Europe, Mexico, and Canada for the treatment of partial seizures and in the United States for the treatment of infantile spasms and partial complex seizures in adults when potential benefits outweigh the risk of developing visual field deficits (see the following text). In experimental seizure models, VGB is active in photosensitive baboons, strychnine- and sound-induced seizures, and amygdala kindling. VGB is essentially inactive in the MES test and in DMCM (methyl-6-7-dimethoxy-4-ethyl-β-carboline-3-carboxylate)-induced clonic seizures and worsens spike-wave seizures in the lh/lh mouse and GAERS rat (Table 40.1) (see ref. [8,61,122, White] for review and references). Further, direct injection of VGB into the median part of the lateral thalamus of GAERS significantly increased the cumulative duration of spike-and-wave discharges (123,124). VGB’s antiseizure activity in the kindled rat and worsening of spike-wave seizures in the lh/lh mouse and GAERS correlates well with its clinical utility against partial seizures and its potential exacerbation of spike-wave seizures, respectively.
VGB, a close structural analogue of GABA, arose from a synthesis program designed to develop molecules that target GABA α-oxoglutarate transaminase (GABA-T; EC 2.6.1.19), the enzyme responsible for GABA metabolism. VGB binds to GABA-T and permanently inactivates the enzyme, thereby increasing brain GABA levels and enhancing GABAergic neurotransmission (Table 40.2). VGB administration to laboratory animals produces a prolonged, dose-related inhibition of GABA-T and corresponding elevation of whole-brain GABA levels (125,126). An increase in all brain regions examined was observed; however, quantitative differences between brain regions were noted (127). Likewise, in human studies, VGB produces a dose-dependent increase in cerebrospinal fluid GABA levels (128–130). In animal studies, there does appear to be a preferential increase in the GABA concentration in the synaptosomal vs. nonsynaptosomal pool (131). Thus, VGB treatment leads to an increased amount of presynaptic GABA available for release, which indirectly leads to increased GABAergic activity at postsynaptic GABA receptors.
The consequent increased activity of GABA on postsynaptic GABA receptors results in increased inhibition of neurons involved in seizure activity and represents the most likely basis for VGB’s clinical activity against partial seizures. The same mechanism that affords efficacy in partial seizures likely contributes to aggravation of generalized absence seizures (36,59,122). For example, increased GABA release in the thalamus will lead to a greater activation of GABAB receptors. Enhanced GABAB receptor activation leads to a prolonged hyperpolarization and subsequent deactivation of T-type Ca2+ currents, which contribute to the synchronized burst firing of thalamocortical neurons.
Unfortunately, vigabatrin use has been associated with irreversible visual field defects in both children and adults [see (132) for review and references] and the emergence of this idiosyncratic adverse event has had a significant impact on the use of this very effective ASD in children with infantile spasms.
Zonisamide
Zonisamide (1,2-benzisoxazole-3-methanesulfonamide; ZNS) was discovered as a result of routine biologic screening of 1,2-benzisoxazole derivatives. ZNS possesses a broad antiseizure profile in animal seizure models (Table 40.1) (8,133). It blocks MES seizures in a number of different species and restricts the spread of focal cortical seizures in cats. In addition, ZNS blocked tonic extension seizures in spontaneous epileptic rats and audiogenic seizures in DBA/2 mice (103). In cats and rats, ZNS also suppresses focal seizure activity induced by cortical freezing and tungstic acid gel, respectively. Moreover, in hippocampal-kindled rats, as well as in amygdala-kindled rats and cats, ZNS suppresses subcortically evoked seizures. In geniculate-kindled cats, ZNS decreases photically induced myoclonus. In the spontaneous epileptic rat, ZNS did not affect spike-wave discharges (103). Clinically, ZNS appears to possess efficacy against a number of seizure types, including partial and secondarily generalized seizures, generalized tonic–clonic, generalized tonic, atypical absence, atonic, and myoclonic seizures (134).
ZNS’s broad antiseizure profile can likely be accounted for by a similarly broad mechanistic profile (Table 40.2). For example, it blocks sustained repetitive firing in cultured spinal cord neurons (134) through an effect on voltage-sensitive Na channels (135). In voltage-clamped Myxicola giant axons, ZNS, like PHT, CBZ, and LTG, appears to retard recovery from fast and slow Na-channel inactivation and produces a hyperpolarizing shift in the steady-state inactivation curve. ZNS has also been demonstrated to reduce voltage-dependent T-type calcium currents in cultured neurons (136) and neuroblastoma cells (137). ZNS also appears to modulate GABA-mediated inhibition. For example, ZNS has been reported to decrease (3H) flunitrazepam and (3H) muscimol binding to the BZD and GABAA receptors, respectively. Similarly, (3H) ZNS binding to rat whole brain membranes is reduced by clonazepam and enhanced by GABA (138). In contrast, ZNS did not affect ion currents evoked by iontophoretically applied GABA (134). Obviously, additional experiments are required to resolve this apparent discrepancy.
Effects on voltage-sensitive Na+ channels are likely to contribute to ZNS’s ability to block MES-induced tonic extension in animals and generalized tonic seizures in humans, whereas effects on low voltage-activated T-currents and perhaps GABA receptors are more likely to correlate with its efficacy against generalized absence and myoclonic seizures, respectively.
THIRD-GENERATION (2001–2015) ASDs
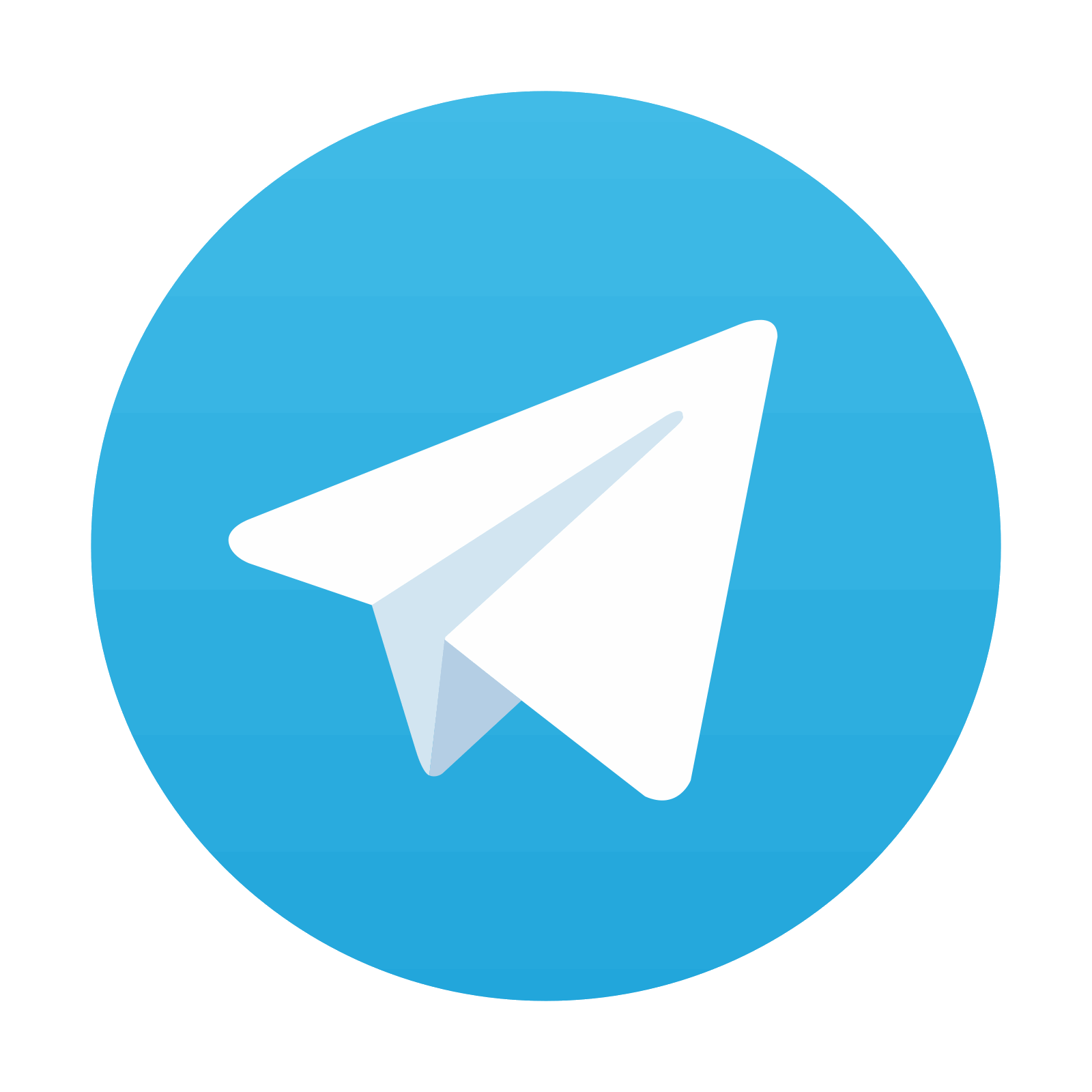
Stay updated, free articles. Join our Telegram channel
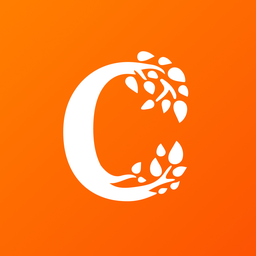
Full access? Get Clinical Tree
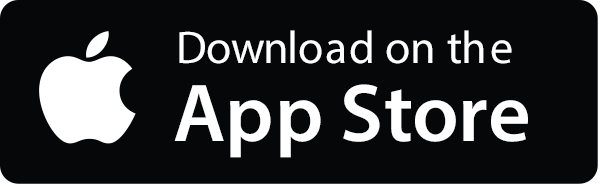
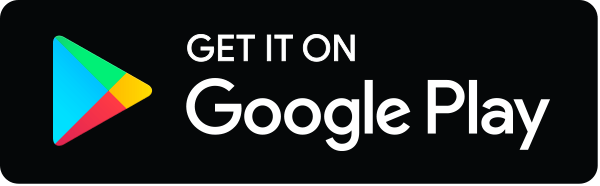