Fig. 65.1
Arrangement of the various Mapleson circuits (a–f). The circuits vary based on the site of introduction of the fresh gas flow (FGF), pop-off or airway pressure relief valve, reservoir bag, and corrugated tubing. The arrangements vary significantly in their efficiency in carbon dioxide removal during either controlled or spontaneous ventilation
The Mapleson A circuit, also known as the Magill’s circuit, has the FGF coming into a corrugated tube, a reservoir bag at the end of the corrugated tubing, and a one-way expiratory valve at the end of the corrugated tubing just proximal to the attachment to the patient (ETT, mask, or laryngeal mask airway). This arrangement is most efficient during spontaneous ventilation as rebreathing does not occur until the FGF is below 70 % of the patient’s minute ventilation (Norman et al. 1967; Kain and Nunn 1967). However, the system is inefficient during positive-pressure ventilation as the expiratory valve must be closed to such a degree that there is limited venting of exhaled gases, thereby resulting in rebreathing. As such, the device is not recommended for use during controlled ventilation (Kain and Nunn 1968; Sykes 1959). The Mapleson B differs from the Mapleson A in that the FGF is adjacent to the expiratory valve at the end of the corrugated tubing. Given the placement of the FGF, the system’s efficiency is similar during spontaneous and controlled ventilation. The position of the FGF allows fresh gas and exhaled gases to accumulate in the corrugated tubing until the pressure increases to such a point that the gas is expelled through the expiratory valve. The gas that is expelled is a mixture of fresh gas and exhaled alveolar gas with the exact percentage of the two depending on the FGF rate. Rebreathing does not occur when the FGF rate is more than twice the minute ventilation during either controlled or spontaneous ventilation (Sykes 1968). The Mapleson C system, also known as the Waters’ circuit without a CO2 absorber, has a setup and design similar to that of the Mapleson B with a much shorter piece of tubing from the bag to elbow adaptor for attachment to the patient. As with the Mapleson B, the pop-off valve is located on the elbow adaptor. The elimination of the tubing or use of a shorter piece of tubing results in greater mixing of exhaled and inspired gases. An FGF of twice the minute ventilation is required to prevent rebreathing of exhaled CO2. A similar device (Fig. 65.2) has been adapted for use during neonatal and pediatric resuscitation to provide preoxygenation and ventilation prior to endotracheal intubation. The modification of the Mapleson C device for resuscitation includes not only an inlet for the delivery of FGF, which is usually connected to a high-pressure oxygen source (wall outlet or standard E cylinder), but also a second attachment site which is hooked to a manometer to allow for pressure measurements during positive-pressure ventilation or the delivery of CPAP. When compared to a standard resuscitation bag (Ambu bag), advantages of the Mapleson C design include the ability to provide an FiO2 of 1.0 for resuscitation, preoxygenation, transport, or positive-pressure ventilation; the ability to accurately assess and change the level of positive-pressure ventilation with the use of a manometer; the ability to deliver CPAP during spontaneous ventilation; and the ability to spontaneously ventilate with limited airway resistance. Additionally, when the modified Mapleson C is attached to an oxygen blender, the FiO2 can be effectively and accurately adjusted for situations where positive-pressure ventilation with a varying FiO2 may be indicated such as infants with single-ventricle physiology or during neonatal resuscitation. The Mapleson D system differs from the Mapleson B only in the location of the pop-off valve. With the Mapleson D, the pop-off valve is located near the reservoir bag instead of on the elbow adaptor. During exhalation, the exhaled gas travels down the corrugated tubing and mixes with the FGF. The composition of the inspired gas is therefore determined by the FGF rate, the exhaled tidal volume, and the expiratory time. A long expiratory time as will occur with a slow respiratory rate results in a longer time for the exhaled gas to flow down the corrugated tubing and be washed out of the circuit through the expiratory valve by the FGF. The system is most efficient during controlled ventilation with a requirement for an FGF rate less than that of the Mapleson A, B, or C system. During spontaneous ventilation, the FGF rate should be approximately twice the minute ventilation to prevent rebreathing (Bain and Spoerel 1973; Soliman and Laberge 1978). A modification of the Mapleson D circuit, known as the Bain system, functions in a manner equivalent to that of the Mapleson D. The difference with the Bain system is that the FGF travels inside the corrugated tubing, therefore allowing the exhaled gas to warm the FGF prior to its administration to the patient. However, the disadvantage of the system is that the FGF line cannot be visualized in the event of its occlusion, disconnection, or kinking.
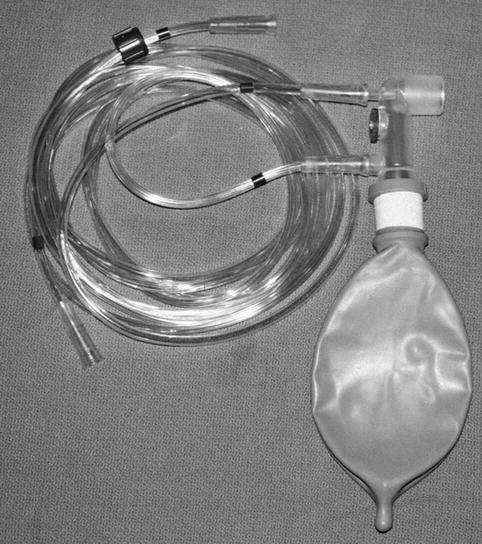
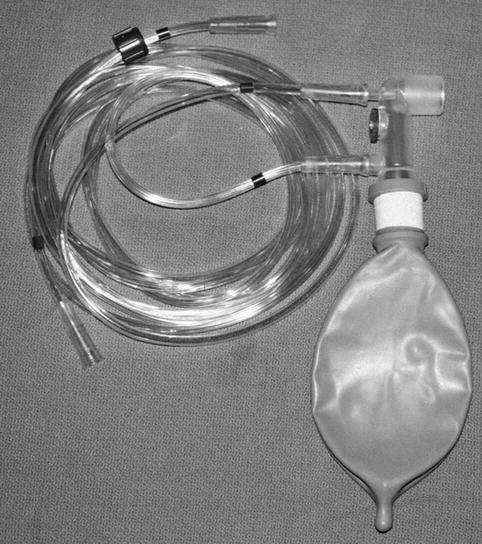
Fig. 65.2
Photograph of a Mapleson C circuit setup which is commonly used during resuscitation for positive-pressure or spontaneous ventilation through an anesthesia mask or an endotracheal tube. The tubing is connected to an oxygen source and a manometer. The adjustable pop-off valve lies between the two connection sites for the tubing
The Mapleson E and F systems are similar and differ only in the addition of a reservoir bag at the end of the corrugated tubing in the Mapleson F system. As opposed to the other Mapleson systems, neither of these systems has a pop-off valve incorporated into the elbow adaptor or the tubing system. The Mapleson E system was a modification of the Ayre’s t-piece which was developed in the 1930s by Dr. Phillip Ayre for use during pediatric anesthesia (Ayre 1937). The Mapleson E system or some modification of its design is commonly used to administer oxygen during spontaneous ventilation through an ETT. It has no valves, thereby limiting resistance to breathing and hence the work of breathing and minimal dead space, and it permits the elimination of rebreathing with FGF rates of two to three times the minute ventilation. The reservoir space of the tubing prevents entrainment of room air provided that the tidal volume is less than the dead space of the tubing. As there is no reservoir bag on the Mapleson E, it cannot be used for positive-pressure ventilation. The modification of the Mapleson E by the addition of the reservoir B at the end of the corrugated tubing results in the Mapleson F system (more commonly known as the Jackson-Rees modification of the Ayre’s t-piece). For use during positive-pressure ventilation, a pop-off value is incorporated into the reservoir bag. This opening in the bag can be occluded by the anesthesia provider to provide CPAP during spontaneous ventilation to allow for positive-pressure ventilation. Based on the FGF rate and the occlusion of the hole, the CPAP or peak inspiratory pressure can be altered. With the Mapleson F system (Jackson-Rees modification of the Ayre’s t-piece), the hole is at the distal end of the reservoir bag and can be occluded between two fingers of the anesthesia provider or with a t-clamp. Alternatively, the hole in the reservoir bag can be on its side (Kuhn’s circuit). FGF rates are similar to those described for the Mapleson D circuit.
Advantages of the Mapleson circuits which maintains their use in the practice of pediatric anesthesia include the ability to switch from controlled to spontaneous ventilation; limited inspiratory resistance and absence of valves, thereby decreasing work of breathing during spontaneous ventilation; and a long history of their use in the provision of pediatric anesthesia. Disadvantages include requirements for high FGF rates with the use of larger quantities of air/oxygen/nitrous oxide; no rebreathing, thereby increasing the requirements and cost of inhalational anesthesia; no humidification or heating of the inspired gas (this can be overcome by the inclusion of a heated humidifier to warm and humidify the FGF prior to its entry into the Mapleson system); and increased environmental pollution.
65.1.1.2 The Circle Anesthesia Breathing Systems
Given these concerns, there is increasing use of a standard circle system (Fig. 65.3) for the provision of anesthesia in the USA. The circle systems are semi-closed systems as rebreathing of the exhaled gases occurs depending on the FGF rate. As such, to prevent issues with the rebreathing of CO2, some type of CO2 absorber is required in these systems. Unidirectional values direct the FGF toward the patient and the expiratory gases toward the CO2 absorber or the exhalation value, thereby limiting rebreathing when the FGF is decreased. With these systems, although the extent of rebreathing is determined primarily by the FGF, very low FGF rates (less than 500 mL/min in an adult) can be used since the CO2 is eliminated from the exhaled gases and rebreathing serves to conserve heat, humidity, and anesthetic gases. The circle system serves to define and distinguish between the different types of anesthesia breathing systems as it can be used as a semi-open (no rebreathing), semi-closed (partial rebreathing), or closed (complete rebreathing) system based on the FGF rate (Moyers 1953). The circle system consists of several key elements including an FGF source, inspiratory and expiratory valves, inspiratory and expiratory corrugated tubing, a Y piece which connects with the standard 15 mm on the airway device (ETT, anesthesia mask, or laryngeal mask airway), a pop-off valve otherwise known as the airway pressure relief (APR) valve, a reservoir bag, and the CO2 absorber cannister. The diameter of the corrugated tubing comes in various sizes to limit the dead space for pediatric patients. Additionally, specialized tubing is available which allows adjustment of the length of the inspiratory and expiratory limbs of the system to allow for the provision of general anesthesia in situations where the anesthesia machine must be placed some distance from the patient due to the presence of other personnel or apparatus such as the cardiac catheterization suite, radiology suite, or during magnetic resonance imaging. However, when the tubing is expanded, the dead space may increase, necessitating the increase of the FGF rate to prevent rebreathing from the dead space of the tubing. During the provision of anesthesia, the amount or presence of rebreathing can easily be assessed by using the end-tidal CO2 monitor which provides not only expiratory CO2 values (end tidal) but also inspiratory values which should be zero if there is adequate gas flow to wash out the CO2 from the dead space of the circuit.
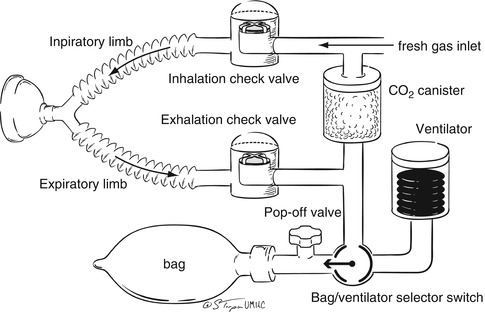
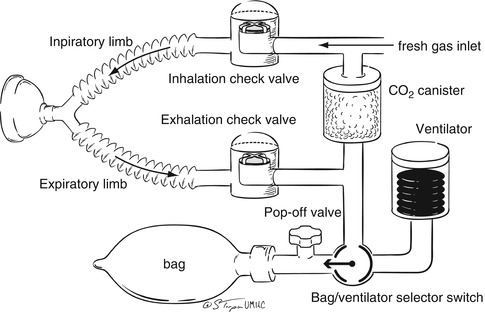
Fig. 65.3
Arrangement of the standard anesthesia circle system used in modern operating rooms for the delivery of anesthetic agents and gas mixtures (oxygen, air, and nitrogen)
65.1.1.3 Carbon Dioxide Absorbers
As some rebreathing of exhaled gases generally occurs with the use of a circle system, a mechanism for scrubbing or removing the exhaled CO2 must be in place. It has minimal dead space and no valves, thereby limiting resistance to breathing and hence the work of breathing. Additionally, it eliminates rebreathing with FGF rates of two to three times minute ventilation. In most circumstances, the rebreathing of exhaled gas is beneficial as it serves to conserve heat and humidity as the exhaled gases are already warmed and humidified as well as decreasing the cost of the care by limiting the need for the inhalational anesthetic agent. The latter is quite important as rebreathing of the exhaled gas and therefore the exhaled inhalational anesthetic agent allows for the decrease in the FGF rate to less than 1 L/min in some cases, thereby significantly decreasing the consumption of the inhalational anesthetic agent. However, whenever exhaled gases are rebreathed, hypercarbia will occur unless the carbon dioxide is removed or “scrubbed” from the exhaled gas. In clinical anesthesia, this is accomplished by passing the exhaled gas over a substance which removes the exhaled carbon dioxide. A similar process is used in other closed areas such as submarines to prevent the rebreathing of exhaled carbon dioxide.
In clinical anesthesia practice, one of three similar substances is used to remove the carbon dioxide from the exhaled gas: soda lime, baralyme, or calcium hydroxide lime (Amsorb). Soda lime, a mixture of chemicals in granular form, removes the carbon dioxide from the exhaled gases. Soda lime is produced by treating slaked lime with a concentrated sodium hydroxide solution. The main components of soda lime are calcium hydroxide or Ca (OH)2 (75–70 %), water (15–20 %), sodium hydroxide or NaOH (3–4 %), and potassium hydroxide or KOH (1 %). The exothermic chemical reaction for the removal of carbon dioxide by soda lime can be summarized as CO2 + Ca(OH)2 → CaCO3 + H2O + heat. Baralyme is a mixture of approximately 80 % calcium hydroxide and 20 % barium hydroxide. Calcium hydroxide lime (Amsorb) is the newest of the three agents available for carbon dioxide scrubbing. The impetus for the production of this agent was to provide an effective agent for carbon dioxide removal that does not contain either sodium or potassium hydroxides. The advantage of the elimination of these agents is that the new product (Amsorb) does not interact with the inhalational anesthetic agents to produce toxic by-products such as carbon monoxide or compound A (see below) (Versichelen et al. 2001).
The production of the carbon dioxide absorbers as a granular form has been studied by trial and error to produce granules which maximize the surface area for the absorption of carbon dioxide and minimize the resistance of the gas as it flows over these granules in the cannister (Hunt 1955). The granule size of soda lime and baralyme is measured in units known as mesh which refers to the number of openings per linear inch in a sieve through which the granules can pass. Soda lime and baralyme have granules that vary in size from 4 to 8 mesh which results in an adequate absorptive surface area for the removal of carbon dioxide with a minimal increase in the resistance to gas flow. In addition to the compounds which absorb carbon dioxide, an indicator is added to these agents to provide a warning that the agent is becoming exhausted or is no longer capable of removing carbon dioxide. As the carbon dioxide absorbing capacity becomes depleted, the pH of the granules decreases and the indicator (ethyl violet) turns from colorless to purple.
Although effective for the removal of carbon dioxide, recent evidence has suggested that the interaction of the carbon dioxide absorbent and various inhalational anesthetic agents may result in potentially deleterious effects including either the production of a toxic compound (compound A or carbon monoxide) or the production of excessive heat with the risk of thermal injury to the airway or production of a fire in the cannister (Fang et al. 1995; Baum et al. 1995). Only carbon dioxide absorbents that contain KOH or NaOH (soda lime and baralyme) can result in the production of carbon monoxide or compound A. No such interaction exists with the newer agent, Amsorb.
The potential interaction of inhalational anesthetic agents and the carbon dioxide absorbent is nothing new in the practice of clinical anesthesia. Such issues were first recognized in the 1950 and 1960s with the inhalational anesthetic agent, trichloroethylene which produces a neurotoxin, dichloroacetylene, and a respiratory toxin or irritant, phosgene. Such problems still potentially exist even with the use of the modern inhalational anesthetic agents. The vinyl ether, compound A, is produced during the metabolism of sevoflurane and its reaction with the soda lime or baralyme in the carbon dioxide absorber of the anesthesia machine (Morio et al. 1992; Frink et al. 1992). The safe concentration of compound A is unknown in humans as is the mechanism of the postulated renal injury (Mazze 1992). Compound A concentrations are increased by several factors including a high inspired concentration of sevoflurane, a low FGF through the system (less than 2 L/min), increasing temperatures of the soda lime cannister, the water content of the CO2 absorbent, and high concentrations of potassium or sodium hydroxides in the CO2 absorbent. Despite the demonstration of potentially nephrotoxic effects of compound A in laboratory animals, even in patients with preexisting renal dysfunction, there are no data to suggest that sevoflurane and compound A lead result in clinical consequences on renal function even during prolonged anesthetics.
Another issue of potential concern regarding the interaction of the inhalational anesthetic agents and the carbon dioxide absorbents is the production of carbon monoxide. Clinically significant amounts of carbon monoxide are produced only when desiccated soda lime or baralyme is used in conjunction with desflurane or the older inhalational anesthetic agent, enflurane (Fang et al. 1995; Baum et al. 1995). The scenario of carbon monoxide toxicity related to such issues has most commonly reported with the first case on a Monday morning when the fresh gas has been left flowing through the anesthesia machine over the weekend resulting in the desiccation of the carbon dioxide absorbent (Berry et al. 1999). Although an extremely rare event, significant morbidity and mortality may occur as carboxyhemoglobin levels of up to 30 % have been reported (Berry et al. 1999). Factors which may increase the production of carbon monoxide include the inhalational anesthetic agent used (desflurane, enflurane) and its inspired concentration, the type of carbon dioxide absorbent, and the water content and temperature of the absorbent.
One final consequence of the use of carbon dioxide absorbents is the potential for an accelerated exothermic reaction with the subsequent generation of significant amounts of heat which, in rare circumstances, can result in the production of a fire. In 2004, Abbott laboratories released a dear healthcare provider letter, and warnings were published in the Anesthesia Patient Safety Foundation letter regarding the potential for an exothermic reaction leading to fires from the interaction of sevoflurane and the CO2 absorbent, Baralyme®. To date, this has been an extremely rare occurrence with a limited number of reports in the literature (Castro et al. 2004; Wu et al. 2004; Fatheree and Leighton 2004). As was noted with the issues regarding the release of carbon monoxide from the interaction of the CO2 absorbent and the inhalational anesthetic agents (see above), the exothermic reaction from the interaction of sevoflurane and Baralyme® occurs only under extreme circumstances when the CO2 absorbent has been desiccated from prolonged high gas flows. Therefore, with proper care and attention, such issues should not be a concern during the routine provision of general anesthesia. However, the potential impact and danger of the extreme circumstances (desiccated CO2 absorbent, high fresh gas flow, and high sevoflurane concentration) are demonstrated by the study of Laster et al. using a lung analogue model (Laster et al. 2004). Baralyme® was desiccated by a heated high oxygen flow for several hours. The CO2 absorbent was then placed in a standard cannister on an anesthesia machine and a 6 L/min flow of oxygen directed through it with either 1.5 MAC of sevoflurane or both 1.5 and 3 MAC of desflurane and isoflurane. A 3-L resuscitation bag was used as a lung model and a minute ventilation of 10 L/min started. With either 1.5 or 3 MAC of isoflurane and desflurane, the temperature within the cannister increased to a peak of approximately 100 °C after 30–70 min. With 1.5 MAC of sevoflurane, the temperature increased to more than 200 °C, and in two of the five cases, flames were noted in the anesthesia circuit.
65.2 Intraoperative Mechanical Ventilation
There are several obvious differences between mechanical ventilation in the operating room and that performed in the ICU setting. In the operating room setting, airway control may be provided by an anesthesia mask, a supraglottic airway device such as the laryngeal mask airway, or an endotracheal tube. As opposed to the ICU setting, the operating room is unusual in that many patients who receive mechanical ventilation have relatively normal pulmonary function and receive endotracheal intubation and controlled ventilation only because of the requirements for the surgical procedure. However, other extremes exist in that patients may have preexisting pulmonary comorbidities which require meticulous intraoperative attention to their requirements for mechanical ventilation. Alternatively, significant intraoperative or postoperative issues may develop (bronchospasm, acid aspiration, laryngospasm) which may require impact on respiratory function.
65.2.1 Controlled Ventilation
Although generally not as efficient as ICU ventilators, the newest generation of anesthesia ventilators allows various options for the mode of ventilation including pressure- or volume-limited ventilation as well as pressure support ventilation during spontaneous ventilation. As in the pediatric ICU, the goals of mechanical ventilation are to provide ventilation (removal of CO2) and oxygenation. In many circumstances, anesthesia ventilators work in the IMV (intermittent mandatory ventilation) mode so that there is no synchronization with spontaneous ventilation. In fact, when potent inhalational anesthetic agents, high doses of propofol, or high doses of opioids are administered, they shift the CO2 response curve to the right to such an extent that the patient’s ventilatory drive is abolished. Additionally, for specific types of surgical procedures (thoracic or neurosurgery) when spontaneous ventilation may be undesirable or deleterious, neuromuscular blocking agents are added to the anesthetic regimen. Given these scenarios, CMV is used most commonly during the intraoperative period. As in the pediatric ICU, ventilation in the operating room is named by the limit variable (pressure or volume) or the parameter which is set to determine the magnitude of the tidal breath. In adult anesthesia practice, the limit variable is most frequently volume. A preset tidal volume is chosen (generally 6–8 mL/kg) and delivered at a rate per minute based on one’s assessment of the patient’s needs for minute ventilation. Alternatively, a pressure limit is used, and the tidal breath provided until a present pressure limit is achieved (pressure-limited ventilation). In general clinical practice, there are no data to demonstrate advantages of one mode over another; however, in specific clinical scenarios, there may be advantages to pressure-limited ventilation. Unzueta et al. randomized 58 adult patients with good preoperative pulmonary function scheduled for thoracic surgery requiring one-lung ventilation (OLV) into two groups (Unzueta et al. 2007). Patients received a tidal volume of 9 mL/kg delivered either by volume-limited ventilation for 30 min followed by pressure-limited ventilation for a similar period of time or by pressure-limited ventilation for 30 min followed by volume-limited ventilation for a similar duration. Although they noted no difference in the oxygenation during the two modes of ventilation, the peak airway pressure required to deliver the tidal volume of 9 mL/kg was lower with pressure-limited ventilation (24.43 ± 3.42 versus 34.16 ± 5.21 cmH2O, p < 0.001).
In addition to choosing the mode of ventilation, the pressure or volume limit, and the rate, anesthesia ventilators allow the setting of an inspiratory time. Although an often forgotten parameter, the inspiratory time can impact on both CO2 removal and oxygenation via its effects on tidal volume and mean airway pressure. With volume-limited ventilation, a specific tidal volume is set and an inspiratory time is chosen. The flow provided is then integrated based on the tidal volume and inspiratory time. For example, if a V T of 500 mL with an inspiratory time of 1 s is chosen, 500 mL will be delivered over 1 s using a gas flow of 30 L/min (500 mL/1 s = 60 L/min). Therefore, during volume-limited ventilation, increasing the inspiratory time will result in a decrease in the flow rate and the delivery of that tidal volume with a lower peak inflating pressure. As such, lengthening of the inspiratory time during the provision of general anesthesia is most frequently performed in patients with altered resistance and compliance as a means of delivering the tidal volume with a lower peak inflating pressure. As most pressure ventilators actually time cycle (end inspiration based on the inspiratory time), increasing the inspiratory time will increase the mean airway pressure and hence the exhaled tidal volume. The increased mean airway pressure improves oxygenation, while the increased tidal volume increases minute ventilation.
With normal spontaneous ventilation, the I:E ratio is 1:3 or 1:4. Therefore, the use of longer inspiratory times may be uncomfortable during spontaneous ventilation, generally not an issue in the operating room during the provision of general anesthesia. In our practice, we frequently use inspiratory times of 0.3–0.5 s for infants and up to 0.7–1.5 s in adolescents. The inspiratory time should also be adjusted based on the underlying disease process. Patients with bronchospasm and air trapping generally benefit from a shorter inspiratory time to allow for as much exhalation time as possible. Patients with alveolar space disease and poor compliance generally do better with longer inspiratory times to increase mean airway pressure and improve oxygenation. The inspiratory time can be increased up to 1.2–1.5 s as needed to increase mean airway pressure and recruit alveoli, but our practice generally restricts the inspiratory time to limit the I:E at 1:1. Reversal of the I:E ratio has been used in the management of patients with severe ARDS in attempts to augment oxygenation and allow weaning of the FiO2 (Schuster et al. 1991). However, longer inspiratory times especially when combined with higher ventilator rates can result in reversal of the I:E ratio which may result in inadequate exhalation times. This may result in air trapping, the stacking of one breath on another (inspiration for the next breath starting before exhalation), thereby resulting auto-PEEP.
Various mechanisms are available for adjusting the inspiratory time, which vary depending on the make and model of the anesthesia ventilator. The inspiratory time may be set as a fixed time (seconds), by adjusting the inspiratory flow rate, as an I:E ratio or as a percentage of the respiratory cycle. If the inspiratory time is set as an I:E ratio or as a percentage of the respiratory cycle, adjusting the rate will affect the actual inspiratory time. For example, if the respiratory rate is set at 15 breaths/min with an inspiratory time of 25 % or an I:E ratio of 1:3, this results in an inspiratory time of 1 s. Changing the rate to 20 breaths/min with the same inspiratory time of 25 % or I:E ratio of 1:3 now results in an inspiratory time of 0.75 s. Such changes can result in changes in the peak airway pressure during volume-limited ventilation or tidal volume during pressure-limited ventilation.
Another option on many anesthesia ventilators is the addition of an inspiratory pause. This time is added to the end of inspiration, thereby lengthening the inspiratory time, so its contribution to the total inspiratory time must be realized to avoid inadvertent reversal of the I:E ratio. This maneuver serves many of the same purposes as lengthening the inspiratory time including the recruitment of alveoli with long time constants (high resistance and low compliance), promotion of collateral ventilation via pores of Cohn and canals of Lambert, reversal of atelectasis, and improved matching of ventilation and perfusion. The inspiratory pause increases mean airway pressure, thereby improving oxygenation. Its effects on compliance result from the resolution of atelectasis and recruitment of lung units.
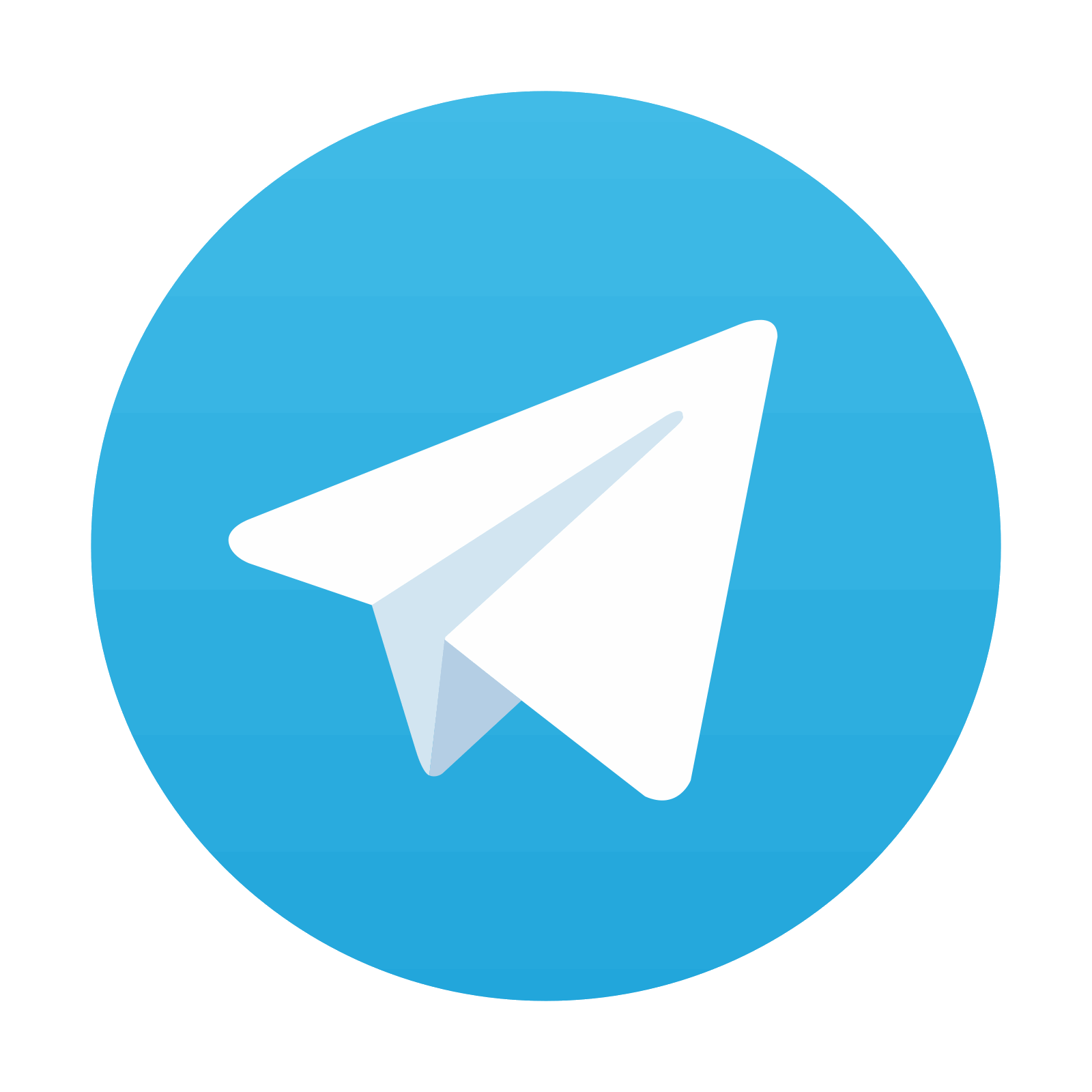
Stay updated, free articles. Join our Telegram channel
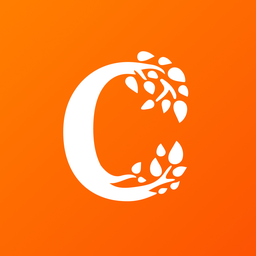
Full access? Get Clinical Tree
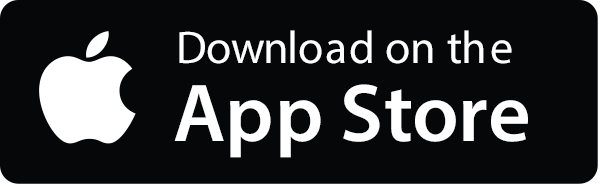
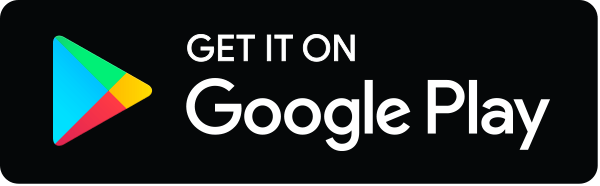